1. Introduction
The central parts of the Balkan Peninsula in Serbia are characterized by extensive Tertiary calc-alkaline magmatism (e.g. Cvetković et al. Reference Cvetković, Prelević, Downes, Jovanovic, Vaselli and Pécskay2004; Prelević et al. Reference Prelević, Foley, Romer, Cvetković and Downes2005; Prelević, Foley & Cvetković, Reference Prelević, Foley, Cvetković, Beccaluva, Bianchini and Wilson2007). Along with widespread volcanic rocks, there are numerous granitoid plutons occurring within the composite terranes of the Vardar Zone and Serbo-Macedonian Massif (Fig. 1; e.g. Karamata et al. Reference Karamata, Delaloye, Lovrić and Knežević1992a, Reference Karamata, Vasković, Cvetković and Knežević-Đorđević1994). They represent part of a regional Tertiary plutonic–volcanic belt stretching from the Periadriatic tonalite line (e.g. von Blanckenburg et al. Reference von Blanckenburg, Früh-Green, Diethelm and Stille1992, Reference von Blanckenburg, Kagam, Deutsch, Oberli, Meier, Wiedenbeck, Barth and Fischer1998; von Blanckenburg & Davies, Reference von Blanckenburg and Davies1995) in the north, through Serbia and further to the SSE in the F.Y.R.O.M. (Former Yugoslav Republic of Macedonia; e.g. Karamata et al. Reference Karamata, Stojanov, Serafimovski, Boev and Aleksandrov1992b), south Bulgaria and northern Greece (e.g. Eleftheriadis et al. Reference Eleftheriadis, Christofides, Mavroudchiev, Nedyalkov, Andreev and Hristo1989; Mavroudchiev et al. Reference Mavroudchiev, Nedyalkov, Eleftheriadis, Soldatos and Christofides1993; Christofides et al. Reference Christofides, Soldatos, Eleftheriadis, Koroneos, Christofides, Marchev and Serri1998).

Figure 1. Distribution of Tertiary granitoids of the Balkan Peninsula. Box with solid outline shows the location of the Mt Cer pluton, whereas the dashed line box shows the location of dacites, and minettes/leucominettes from the area of Veliki Majdan. 1 – area of Miocene granitoids cropping out along the southern margin of the Pannonian basin; 2 – area of Oligocene granitoids cropping out in the Dinarides. Geotectonic units/terranes after Karamata et al. (Reference Karamata, Vasković, Cvetković and Knežević-Đorđević1994): DOBT – Dinaride Ophiolite Belt Terrane; DIT – Drina-Ivanjica Terrane; JBT – Jadar Block Terrane; VZCT – Vardar Zone Composite Terrane; SMCT – Serbo-Macedonian Composite Terrane; CBCT – Carpatho-Balkanides Composite Terrane.
Oligocene plutons are predominant in the area and mainly show I-type characteristics. They originated during post-orogenic dextral transpressional movements associated with wrench tectonics and formation of lacustrine basins along the central axis of the Balkan Peninsula (Fig. 1; Marović et al. Reference Marović, Mihailović, Đoković, Gerzina and Toljić2001). The Miocene Mt Cer and Mt Bukulja plutons are located in the southern Pannonian area. These rocks show S-type characteristics and are distinguished from the Oligocene granitoids of the Dinaride suite on the basis of age, petrography, geochemistry and metallogeny (Fig. 1; Steiger, Knežević & Karamata, Reference Steiger, Knežević and Karamata1989; Karamata et al. Reference Karamata, Delaloye, Lovrić and Knežević1992a).
The Mt Cer pluton is a composite pluton (Cvetković et al. Reference Cvetković, Koroneos, Christofides, Poli, Knežević and Erić2002) comprising both I- and S-type granitoid rocks. It is situated at the junction between the northern Dinarides and the southern margin of the Pannonian Basin, and is, hence, a key point for elucidating the geodynamic significance of the Tertiary granitoid magmatism in this part of the Balkan Peninsula.
A precise geodynamic interpretation of the Tertiary granitoids of Serbia and adjacent regions is still a matter of debate. Pamić (Reference Pamić1977, Reference Pamić1985/1986) considered all Tertiary granitoids of the central Balkan Peninsula and southern Pannonian domain as related to a magmatic arc setting, suggesting that active subduction processes played an important role in this region during Tertiary times. Pamić, Balen & Herak (Reference Pamić, Balen and Herak2002) argued that these granitoids formed during the Oligocene to Early Miocene post-collision (e.g. Liégeois et al. Reference Liégeois, Navez, Hertogen and Black1998). Cvetković, Knežević & Pécskay (Reference Cvetković, Knežević, Pécskay and Janković2000), Cvetković et al. (Reference Cvetković, Koroneos, Christofides, Poli, Knežević and Erić2002) and Pécskay et al. (Reference Pécskay, Knežević, Cvetković and Resimić-Šarić2001) gave evidence for post-collisional origin of the Oligocene I-type granites, whereas the Miocene S-type granitoids were interpreted as having resulted after the Pannonian extension.
On the basis of Sr–Nd isotopic data, Steiger, Knežević & Karamata (Reference Steiger, Knežević and Karamata1989) differentiated Mt Cer rocks into mantle-derived I-type granitoids and upper crustally derived S-type granitoids. This distinction was further supported by Karamata et al. (Reference Karamata, Steiger, Đorđević and Knežević1990, Reference Karamata, Delaloye, Lovrić and Knežević1992a, Reference Karamata, Vasković, Cvetković and Knežević-Đorđević1994) and Knežević, Karamata & Cvetković (Reference Knežević, Karamata and Cvetković1994), who reported different ages and metallogenic affinities for the two groups and concluded that I-type and S-type granitoids originated by melting of different crustal materials in response to the uprising of isotherms. Recently, Cvetković et al. (Reference Cvetković, Poli, Christofides, Koroneos, Pecskay, Resimic-Saric and Eric2007) argued that the two-mica granites from the Mt Bukulja pluton, situated some 80 km southeast from the Mt Cer pluton, originated during tectonomagmatic events controlled by the early extensional phases during the opening of the Pannonian basin.
In this study we present new geochronological, mineralogical, geochemical and isotope data for Mt Cer granitoids. We compare mineralogical and geochemical characteristics between the Mt Cer and Mt Bukulja granitoid rocks and adjacent lamprophyric rocks of similar age. In particular, the composition of Mt Cer granitoids is compared with the composition of Veliki Majdan minettes/leucominettes and dacites situated a few tens of kilometres to the southwest (Prelević et al. Reference Prelević, Foley, Cvetković and Romer2004). We develop the idea of Cvetković et al. (Reference Cvetković, Poli, Christofides, Koroneos, Pecskay, Resimic-Saric and Eric2007), who suggested that the Mt Bukulja and Mt Cer plutons are petrogenetically linked, and that lamprophyric magmas have probably played an important role in the genesis of the Mt Bukulja pluton. The present work aims to clarify the origin and evolution of the Mt Cer pluton and to elucidate timing and petrogenesis of two different magmatic episodes interpreted as the result of slight changes in geodynamic conditions.
2. Geology and petrography of the Mt Cer pluton
The Mt Cer pluton is an E–W-elongated laccolith-like intrusion covering an area of about 60 km2 (Figs 1, 2). The country rocks are, in fact, dipping periclinally from the pluton and the angles are less steep in the eastern and western parts. The pluton intrudes metamorphic rocks of Jadar Block Terrane (Karamata et al. Reference Karamata, Vasković, Cvetković and Knežević-Đorđević1994), made by a Middle Devonian to Early Permian series composed of weakly metamorphosed sedimentary protoliths now mostly represented by phyllites and sericite–chlorite and chlorite schists (Filipović, Sikošek & Jovanović, Reference Filipović, Sikošek and Jovanović1993) metamorphosed under greenschist-facies conditions. These metamorphic rocks surround the whole pluton except in the south, where the pluton is cut by the E–W-oriented Lešnica normal fault (Fig. 2). Geophysical data (Labaš, Reference Labaš1975) revealed a negative gravimetric anomaly with a maximum around 5 km to the south of the Lešnica structure, implying that the pluton continues southwards. This part is today covered by 600–800 m thick Miocene breccias containing granite boulders. Thus, only the northern edge of the exposed intrusive body corresponds to the real margin of the pluton. The Stražanica intrusion crops out in the northwest as an N–S-elongated small body (~ 7 km2) separated from the main pluton by a narrow belt of contact-metamorphosed rocks (Fig. 2). It is worth noting that thermal metamorphic effects are weaker around the main body (from rocks unaffected by contact metamorphic to tourmaline hornfels) and stronger around the Stražanica intrusion (andalusite-, biotite- and (rarely) garnet-bearing spotted hornfels: Knežević, Reference Knežević1962). Such different metamorphic assemblages can be explained by contact metamorphism occurring at higher and lower pressures, around the main body and the Stražanica intrusion, respectively. Petrofabric investigations reveal the presence of a foliated magmatic fabric in the northern margin of the pluton, represented by northerly dipping mica flakes and elongated crystals of plagioclase and hornblende. Other Mt Cer granitoid lithologies do not show detectable microfabric anisotropy.
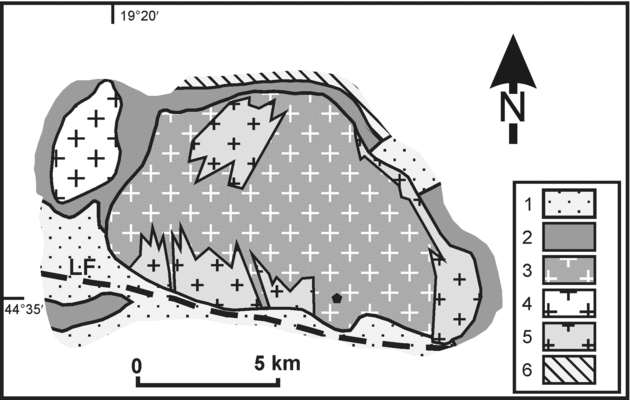
Figure 2. Simplified geological sketch map of the Mt Cer composite pluton. 1 – Neogene and Quaternary sediments; 2 – contact metamorphic rocks; 3 – quartz monzonites to quartz monzodiorites (QMZD); 4 – granodiorites to quartz monzonites from Stražanica (GDS); 5 – areas of occurrence of two-mica granites (TMG); 6 – Palaeozoic schist. Black pentagon – area of occurrence of large enclave; LF – Lešnica fault (mostly covered).
The Mt Cer granitoids are classified following the classification scheme of Streckeisen & Le Maitre (Reference Streckeisen and Le Maitre1979) (Fig. A1; online Appendix at http://journals.cambridge.org/geo), and on the basis of petrography. Three main groups of rocks can be recognized: quartz monzonites to quartz monzodiorites (QMZD), two-mica granites (TMG), and Stražanica granodiorites to quartz monzonites (GDS). The spatial distribution of the various rock types is shown in Figure 2.
Quartz monzodiorite rocks compose the central parts of Mt Cer constituting around 60 vol. % of the whole pluton. They crop out within deep creeks as well as on some hills. Two-mica granites are found in the eastern, northwestern and southwestern peripheral parts of the Mt Cer pluton (Fig. 2). They occur as metre-thick veins, dykes, or larger irregular bodies and always show sharp contacts towards QMZD. Stražanica granodiorites to quartz monzonites constitute a quite homogeneous body situated at the northwesternmost side of Mt Cer.
Quartz monzodiorite rocks are grey to dark grey in colour, massive but with some magmatic foliation in the northern parts. The texture is allotriomorphic granular, but porphyritic varieties with large grains of K–feldspar also occur. Selected chemical microprobe analyses of feldspars, biotite, muscovite and amphibole are reported in Table A1 (online Appendix at http://journals.cambridge.org/geo). Xenomorphic and undulose quartz (~ 27 vol. %), K–feldspar (~ 26 vol. %; Or87.3–90.0), plagioclase (~ 30 vol. %; An31.3–37.3/cores to An29.9–35.8/rims), biotite (8–18 vol. %), and hornblende (0–8 vol. %) are the main constituents of QMZD, whereas titanite, allanite, magmatic epidote, zircon, apatite, rutile, magnetite and ilmenite are present as accessory minerals.
Two-mica granites are fine- to medium-grained, white-grey in colour with hypidiomorphic granular texture and massive structure. They mainly consist of quartz, (29–41 vol. %), K-feldspar (27–38 vol. %; Or86.5–90.1), plagioclase (14–32 vol. %; An8.7–13.4/cores to An7.8/rims), muscovite (5–15 vol. %) and biotite (usually <5 vol. %), as well as accessory garnet, tourmaline, apatite, rutile and zircon.
Stražanica granodiorite to quartz monzonite rocks are light-grey in colour and display a uniform hypidiomorphic medium-grained texture. They are composed of quartz (~ 25 vol. %), plagioclase (~ 47 vol. %; An26.4/cores to An21.1/rims), K–feldspar (~ 15 vol. %; Or91.6), and biotite (~ 10 vol. %), while zircon, allanite, apatite, muscovite, epidote and titanite are accessory phases.
Mafic enclaves (ME) are found in QMZD exclusively. They crop out only in the marginal parts of QMZD as scarce dark-green elongated schlieren and lensoidal to rounded enclaves. They are around 5 × 10 cm in size, rarely smaller, and show a hypidiomorphic granular texture. They have amphibole (up to 73 vol. %), plagioclase (~ 16 vol. %; An31.3/cores to An33.3/rims) and biotite (~ 8 vol. %) as main constituents, and K–feldspar (Or88), titanite, apatite, allanite and epidote as accessory minerals. On the southern slopes of Mt Cer, a large (1 × 1.5 m) mafic enclave was found in QMZD (Knežević, Karamata & Cvetković, Reference Knežević, Karamata and Cvetković1994). This enclave is substantially richer in biotite (~ 45 vol. %) and K–feldspar (~ 28 vol. %) and poorer in plagioclase (~ 15 vol. %) and amphibole (~ 10 vol. %), in comparison to the smaller mafic enclaves. Knežević, Karamata & Cvetković (Reference Knežević, Karamata and Cvetković1994) argued for a lamprophyric character for this mafic enclave because they found higher MgO contents in biotite and hornblende from the enclave than in biotite and hornblende from the host rock (QMZD). Unfortunately, this lithology is no longer available for sampling due to the extensive cover, ruling out more detailed study of this type of enclaves.
The dominant ferromagnesian phase in all the rock types is biotite, except in the enclaves where hornblende prevails. Determination of the biotite composition was made for the main groups of Mt Cer rocks. In an Altot v. Fe no. plot (Fig. 3a) the compositions of most QMZD biotites plot in the minette/leucominette field, as do the compositions of biotites from enclaves, which display, however, lower Fe no. values. By comparison, biotites from hornblende–biotite granites (H-BG) of Mt Bukulja (Cvetković et al. Reference Cvetković, Poli, Christofides, Koroneos, Pecskay, Resimic-Saric and Eric2007) have the same Altot range, but higher Fe no. than biotites from QMZD. Biotites from TMG are compositionally similar to those from two-mica granites from Mt Bukulja and have high Fe no., as expected for such evolved rock compositions.

Figure 3. Altot v. Fe/(Fe+Mg) (Fe no.; cation basis) diagram showing the chemical compositions of biotite of the Mt Cer granitoid (a). The compositions of biotites of H-BG (hornblende–biotite granite) and TMG (two-mica granite) rocks from Bukulja pluton (Cvetković et al. Reference Cvetković, Poli, Christofides, Koroneos, Pecskay, Resimic-Saric and Eric2007) and of minettes/leucominettes from Veliki Majdan (Prelević et al. Reference Prelević, Foley, Cvetković and Romer2004) are also displayed. (b) and (c) show probability density distribution diagrams for analysed amphibole for pressure (MPa) and temperature (°C), respectively. The number of analyses is 15 and 9 for QMZD and ME, respectively.
Mafic enclave and quartz monzonite/quartz monzodiorite rocks were selected for plagioclase–hornblende thermobarometry. All selected samples have a mineral assemblage of plagioclase, hornblende, K-feldspar, biotite, titanite, magnetite and quartz, which is an important prerequisite for applying aluminium-in-hornblende barometry (e.g. Hammarstrom & Zen, Reference Hammarstrom and Zen1986; Schmidt, Reference Schmidt1992; Anderson & Smith, Reference Anderson and Smith1995; Stein & Dietl, Reference Stein and Dietl2001). Because the influence of temperature (and oxygen fugacity) on pressure calculations has to be considered, we have chosen the calibration of Anderson & Smith (Reference Anderson and Smith1995) to calculate crystallization pressures. Equilibration temperatures for hornblende–plagioclase assemblages were calculated on the basis of iterations started from pressures calculated using Anderson & Smith (Reference Anderson and Smith1995) and using various thermometers. Preferred values according to Anderson (Reference Anderson1996) have been accepted (see also Anderson et al. Reference Anderson, Barth, Wooden, Mazdab, Putirka and Tepley2008; Holland & Blundy, Reference Holland and Blundy1994). Accordingly, temperature and pressure values were calculated using Microsoft Excel spreadsheet ‘Plagioclase–Hornblende Thermobarometry’ (Anderson et al. Reference Anderson, Barth, Wooden, Mazdab, Putirka and Tepley2008: http://www.minsocam.org/MSA/RIM/RiM69_Ch04_hbld_plag_thermo-jla.xls).
Histograms of pressure (Fig. 3b) and temperature (Fig. 3c) estimates for amphibole rims are reported as probability density plots. Although the errors for both geobarometer and geothermometer are high, owing to errors connected with regression of data, chosen temperature values and analytical imprecision, the values of around 60 MPa and 30 °C can be considered conservative for pressure and temperature, respectively (e.g. Zhang, Zhao & Song, Reference Zhang, Zhao and Song2006). On the other hand, our goal was to discuss differences in crystallization pressures between QMZD and ME, rather than estimating the absolute values. The ranges of crystallizing pressures and temperatures for QMZD rocks are 350–550 MPa and 700–770 °C, respectively. The maximal crystallizing pressure for QMZD rocks is about 500 MPa with a minimum at lower pressures at about 350 MPa. The maximum temperature is estimated at about 730 °C. Estimated crystallizing pressures for ME rocks have two peaks at 400 MPa and 250 MPa, whereas the maximum temperature is 680 °C with a lower peak at about 740 °C. Although there is a clear overlap of data, QMZD and ME rocks show different crystallizing pressures and temperatures. Relatively higher pressures obtained for QMZD are in accordance with the presence of primary magmatic epidote in some QMZD samples (e.g. Zen & Hammarstrom, Reference Zen and Hammarstrom1984; Vyhnal, McSween & Speer, Reference Vyhnal, McSween and Speer1991).
2.a. Age
New and available radiometric data (Table 1), along with geological and petrological evidence, provide some constraints for the age interpretation. 40K–39Ar data on hornblende reveal ages of c. 21 Ma for QMZD, whereas biotite and feldspars (Kf + Plg) from the same rock give younger ages (17–16 Ma). The GDS biotite and feldspar fractions (Kf + Plg) yielded ages of c. 17 Ma and 19 Ma, respectively. The pressure estimates for QMZD rocks (~ 400–600 MPa) imply that these rocks have started to crystallize rather deep in the crust, in accordance with a weak contact–metamorphic aureole. Consequently, the 40K–39Ar hornblende age of QMZD (c. 21 Ma) can be regarded as a minimum emplacement age, whereas the 40K–39Ar determinations on biotite can be regarded as minimum cooling ages. On the other hand, the possibility that the intrusion of TMG could have induced thermal rejuvenation effects on micas and feldspars of older ME and QMZD seems unlikely, because TMG intrude the QMZD body only locally, and therefore the thermal effect must have been more localized. Much stronger contact-metamorphic effects around GDS suggest that their crystallization and emplacement must have occurred in shallower levels. The 40K–39Ar estimate on GDS biotite can be regarded as a minimum cooling age, and the similarity with the age obtained on QMZD biotite could indicate that GDS and QMZD crystallized roughly contemporaneously. Feldspars (Kf + Plg) and muscovite from TMG rocks gave the youngest ages and the lowest age range of 15–16 Ma; this is in accordance with the mode of their occurrence. Two-mica granites sharply cut the QMZD rocks, hence they must have been emplaced during the final stage of the evolution of the Mt Cer pluton. A mafic enclave sample exhibits the largest age range, from around 26 Ma obtained on hornblende to around 18 Ma and 17 Ma obtained on biotite and feldspars (Kf + Plg), respectively. The values of 26 and 18 Ma cannot be considered as minimum initial crystallization and cooling ages, respectively, because 8 Ma is too large a time span from the onset of crystallization to its termination for such a small (decimetric) magmatic body. Consequently, we consider the estimated value of 26 Ma as having resulted from Ar retention, which often happens in K/Ar dating of hornblende (e.g. McDougall & Harrison, Reference McDougall and Harrison1999). On the other hand, the 40K–39Ar biotite age of 18 Ma can be regarded as a minimum cooling age. It is noteworthy that such an age is similar to the 40K–39Ar biotite age obtained for QMZD.
Table 1. New and published 40K–39Ar ages of Mt Cer granitoid rocks

3. Geochemical characterization of the Mt Cer granitoid rocks
On the basis of their spatial distribution, mineralogy, texture and extent of alteration, nineteen samples were selected for major and trace element analyses (Table 2). Nine samples were analysed also for REE (Rare Earth Elements; Table 3), and seven samples for Sr and Nd isotopes (Table 4). In addition, major element and Sr isotope data for six samples were taken from the literature (Karamata et al. Reference Karamata, Steiger, Đorđević and Knežević1990; Knežević, Cvetković & Resimić, Reference Knežević, Cvetković and Resimić1997). Analytical techniques are reported in the Appendix A1 available online at http://journals.cambridge.org/geo.
Table 2. XRF chemical analyses of major (wt%) and trace element (ppm) contents of selected samples from the Mt Cer pluton

*From Karamata et al. Reference Karamata, Steiger, Đorđević and Knežević1990. †From Knežević, unpub. data. §From Knežević, Cvetković & Resimić, 1997. ASI (aluminum-saturation index) = molecular Al/(Ca+1.67*P+Na+K).
Table 3. Rare earth element and trace element contents for representative Mt Cer granitoids, analysed by ICP-MS

Eu* = Eu anomaly, calculated by dividing the chondrite-normalized Eu concentration of a rock by the half-sum of the normalized concentrations of Sm and Gd.
Table 4. Sr and Nd isotopic compositions of representative Mt Cer granitoids

References for published samples are given in Table 2. n.d. – not determined.
This study reveals complex spatial–chemical relationships between the above-described types of granitoid rocks. Quartz monzonite/quartz monzodiorite rocks predominantly show a high-K calc-alkaline affinity, whereas TMG samples plot in the high-K calc-alkaline–shoshonitic fields according to Peccerillo & Taylor (Reference Peccerillo and Taylor1976) (Fig. A2; online Appendix at http://journals.cambridge.org/geo). Mafic enclaves are variable but shoshonitic in character, whereas GDS samples are mainly medium-K calc-alkaline. Quartz monzonite/quartz monzodiorite rocks and GDS are metaluminous, whereas TMG are peraluminous with ASI up to 1.35. Petrographic and geochemical characteristics indicate that QMZD and GDS are I-type rocks, whereas TMG are S-type rocks.
Contents of some major oxides and trace elements (TiO2, CaO, P2O5, V, Co, Cr, Zr, Ba; Fig. 4a) follow a single trend from minettes/leucominettes of the Veliki Majdan area (Prelević et al. Reference Prelević, Foley, Cvetković and Romer2004) to two-mica granites from both the Mt Cer and Mt Bukulja plutons. Al2O3, Na2O, Fe2O3tot and Y contents (Fig. 4b) are uniform in intermediate-acid rocks, but increase noticeably from minettes/leucominettes to dacites. K2O, Rb, Th and Nb concentrations (Fig. 4c) follow a trend from minettes/leucominettes to two-mica granites, although the latter samples are very scattered. Quartz monzonite/quartz monzodiorite and GDS samples plot at the lower end of the trend formed by dacite of the Veliki Majdan area (Prelević et al. Reference Prelević, Foley, Cvetković and Romer2004) and Mt Bukulja hornblende–biotite granites (H-BG), whereas TMG samples from Mt Cer plot at the upper end of the TMG Mt Bukulja group. The behaviour of Sr contents (Fig. 4d) is peculiar: minettes/leucominettes, dacites, all the Mt Bukulja rocks, and TMG rocks from Mt Cer follow a single trend, whereas QMZD and GDS samples show distinctively higher Sr contents. Regarding the ME group, the three ME samples, as already mentioned, have heterogeneous compositions. The sample poorest in SiO2 does not fit to any of the groups reported in Figure 4, whereas the other two samples plot within the dacite group for many elements, except for K2O, Rb, Th, Nb and Sr. In addition, the two ME samples with similar SiO2 contents show remarkable differences in K2O, Rb, Th and Nb contents.

Figure 4. Major and trace element variation diagrams for the Mt Cer plutonic rocks. The whole rock composition of H-BG and TMG rocks from the Mt Bukulja pluton (Cvetković et al. Reference Cvetković, Poli, Christofides, Koroneos, Pecskay, Resimic-Saric and Eric2007), as well as dacites (square hatched field) and minettes/leucominettes from Veliki Majdan (Prelević et al. Reference Prelević, Foley, Cvetković and Romer2004) are also shown. Other symbols as in Figure 3.
Rare earth element patterns are fractionated and total REE contents decrease from ME to TMG (Fig. 5a). REE concentrations decrease also from minette/leucominette to TMG (Fig. 5b–d), and mimic the trend followed by K2O (Fig. 4c). The negative Eu-anomaly slightly decreases from minette/leucominette to hornblende–biotite granite, whereas it displays high values in both Mt Bukulja and Mt Cer two-mica granite (Fig. 5e). Values of (La/Yb)n are very scattered for all groups of samples, with minette/leucominette plotting into two quite distinct areas (Fig. 5f).

Figure 5. Chondrite normalized REE patterns (chondrite values from Haskin et al. Reference Haskin, Frey, Schmitt, Smith, Ahrens, Press, Runcorn and Urey1966), La, Nd, Yb, (La/Yb)n, and Eu/Eu* v. SiO2 for the Mt Cer plutonic rocks, H-BG and TMG rocks from the Mt Bukulja pluton (Cvetković et al. Reference Cvetković, Poli, Christofides, Koroneos, Pecskay, Resimic-Saric and Eric2007), as well as dacites and minettes/leucominettes from Veliki Majdan (Prelević et al. Reference Prelević, Foley, Cvetković and Romer2004). Symbols as in Figures 3 and 4.
Quartz monzonite/quartz monzodiorite and dacite samples from the Veliki Majdan area show similar primitive mantle-normalized spider diagram patterns, except for Sr and Nb (Fig. 6a). On the other hand, two-mica granites from Mt Cer display similar patterns to those shown by TMG from Mt Bukulja (Fig. 6b), whereas GDS rocks show no geochemical similarities either with TMG or with QMZD rocks (Fig. 6b).

Figure 6. Primitive mantle-normalized multi-element spider diagrams for QMZD in comparison to dacites and minette/leucominettes from Veliki Majdan (a), and for GDS and TMG in comparison to Mt Bukulja two-mica granites (b). Data for dacites and minettes/leucominettes are from Prelević et al. (Reference Prelević, Foley, Cvetković and Romer2004). Data for Bukulja two-mica granites are from Cvetković et al. (Reference Cvetković, Poli, Christofides, Koroneos, Pecskay, Resimic-Saric and Eric2007). Normalization values for primordial mantle are from Wood et al. (Reference Wood, Joron, Treuil, Norry and Tarney1979), but for Pb from McDonough et al. (Reference McDonough, Sun, Ringwood, Jagoutz and Hofmann1992). Symbols as in Figures 3 and 4.
Sr and Nd isotope compositions are shown in Figure 7. Generally, the initial 87Sr/86Sr and 143Nd/144Nd ratios decrease and increase with evolution, respectively. Quartz monzonite/quartz monzodiorite rocks have similar isotopic compositions to dacites of the Veliki Majdan area, whereas TMG rocks from Mt Cer exhibit the highest 87Sr/86Sri and the lowest 143Nd/144Ndi values of the entire suite. It is noteworthy that two-mica granites from Mt Cer and Mt Bukulja are different in terms of Sr and Nd isotopes.

Figure 7. SiO2 (wt %) v. (87Sr/86Sr)20Ma and (143Nd/144Nd)20Ma for the Mt Cer plutonic rocks. H-BG and TMG rocks from Bukulja pluton (Cvetković et al. Reference Cvetković, Poli, Christofides, Koroneos, Pecskay, Resimic-Saric and Eric2007) as well as dacites and minettes/leucominettes from Veliki Majdan (Prelević et al. Reference Prelević, Foley, Cvetković and Romer2004). Symbols as in Figure 3.
4. Discussion of source and evolution processes
Any petrogenetic model intended to explain the genesis and differentiation of the granitoid facies of the Mt Cer pluton must take into account their field, petrographic and geochemical characteristics, namely: (1) lack of clear evidence of magma interaction both from a petrographic and geochemical point of view; (2) compositional similarity between QMZD and dacites of the Veliki Majdan area, the latter being genetically related to minettes/leucominettes (Prelević et al. Reference Prelević, Foley, Cvetković and Romer2004); (3) major and trace element compositional similarity between Mt Cer and Mt Bukulja TMG rocks, while having different Sr–Nd isotope ratios; (4) geochemical and isotope dissimilarity between GDS and any other above-mentioned rock group.
In the following discussion, we try to unravel source and evolution processes of each granitoid facies, also aiming to understanding their mutual genetic relationships.
4.a. QMZD
An important issue in deciphering the origin and evolution of the main body of the Mt Cer pluton is the petrogenetic significance of the ME group. Following the classification of Didier & Barbarin (Reference Didier, Barbarin, Didier and Barbarin1991), mafic enclaves could be either ‘mafic microgranular enclaves’ (hereafter MME), implying that they derive from interaction processes between different magma batches (e.g. Poli, Tommasini & Halliday, Reference Poli, Tommasini and Halliday1996; Janousek et al. Reference Janousek, Braithwaite, Bowes and Gerdes2004), or cognate enclaves, suggesting that they represent accumulations of fractionated phases (e.g. Nitoi et al. Reference Nitoi, Munteanu, Marincea and Paraschivoiu2002; Donaire et al. Reference Donaire, Pascual, Pin and Duthou2005).
Structures found in the field are important for deciphering the true genesis of enclaves. The Mt Cer ME are found in the peripheral parts of the QMZD body and they are all decimetric in size with only a few smaller enclaves. These are uncommon features for MME, because the MME are commonly found throughout the plutons, and they have different dimensions (e.g. Barbarin & Didier, Reference Barbarin, Didier, Didier and Barbarin1991). The ME samples have lobate and rounded shapes exhibiting rare chilled margins. These features are common both in MME (Blake & Fink, Reference Blake and Fink2000) and cognate enclaves (Nitoi et al. Reference Nitoi, Munteanu, Marincea and Paraschivoiu2002), and it is not uncommon to find them occurring contemporaneously in the same MME enclave. These findings cannot give unequivocal indications as to the real nature of the ME rocks. A very common MME feature at the macro-scale is the occurrence of host-derived crystals (usually plagioclase, K-feldspar, biotite), either within the enclaves or even cross-cutting the contacts between the enclaves and host (e.g. Collins et al. Reference Collins, Richards, Healy and Ellison2000; Barbarin, Reference Barbarin2005). These features are considered as proofs of mass transfer between the interacting magmas and as indications that the enclaves were in a liquid state upon their incorporation into the more felsic magma (e.g. Vernon, Reference Vernon1984; Castro, Mereno-Ventas & de la Rosa, Reference Castro, Mereno-Ventas and de la Rosa1991; Perugini et al. Reference Perugini, Poli, Christofides and Eleftheriadis2003). However, this feature is lacking in the ME rocks both in the field and on a thin-section scale.
Apart from the large enclave found in the southern part of the pluton, the mineralogical assemblage of the Mt Cer ME rocks is dominated by mafic minerals (> 80 vol. %). This feature is uncommon for mafic microgranular enclaves, usually composed of both mafic minerals and feldspars (e.g. Barbarin & Didier, Reference Barbarin, Didier, Didier and Barbarin1991; Qin et al. Reference Qin, Lai, Grapes, Diwu, Ju and Li2009). Mafic microgranular enclave mineralogical composition depends on the bulk compositions of mafic and felsic end-members and the extent of their chemical interaction. Enclaves derived by subtle chemical interactions are poorly evolved and richer in mafic minerals, so they are compositionally closer to the mafic end-member. In this context, ME rocks from Mt Cer cannot represent the mafic end-member of any magma interaction process because such large amounts of mafic minerals are uncommon for mafic compositions of calc-alkaline (sensu lato) rocks, such as non-cumulitic gabbros and diorites (e.g. Blundy & Sparks, Reference Blundy and Sparks1992). Poikilitic texture is common in some Mt Cer ME rocks, although clear cumulitic textures with cumulus and intercumulus phases are rare.
Compositions of amphibole and biotite from the enclaves differ from those shown by these minerals from the host (Table A1; online Appendix at http://journals.cambridge.org/geo). This is very unusual for enclaves and hosts derived by magma interaction processes because the mechanical interaction and predominant diffusion between the two magmas tend to reduce compositional differences of the mineralogical phases (e.g. Pe-Piper, Reference Pe-Piper2007).
Chemical compositions of the Mt Cer ME rocks vary greatly. For instance, the enclave with the lowest silica content (113a) has similar or even lower concentrations of compatible elements in comparison to the silica-rich enclaves. This indicates that the Mt Cer ME cannot be considered as having crystallized from liquids. In addition, the ME and QMZD define clear geochemical trends only for a few elements. The geochemical trends defined by MME and their hosts are controlled by relative compositions of end-members and by bulk partition coefficients (e.g. Poli & Tommasini, Reference Poli and Tommasini1991; Elburg, Reference Elburg1996; Karsli et al. Reference Karsli, Chen, Aydin and Sen2007). Compatible element contents in typical MME and hosts usually form continuous trends, but this is not the case for the Mt Cer ME and QMZD.
All these findings support the hypothesis that the Mt Cer ME have a cognate origin, that is, that they likely represent clusters of fractionated phases that formed during the evolution of the QMZD magmas, rather than mafic microgranular enclaves. By contrast, the large enclave found in the southern part of the pluton may represent a MME because it has a typical MME mineralogical assemblage. This suggests that magma interaction processes might have had some role, even if a minor one, in the genesis of QMZD.
It is commonly accepted that textures such as boxy cellular crystals, oscillatory zoning, calcic spike zones and combinations of these within individual plagioclase crystals are indicative of disequilibrium crystallization (Hibbard, Reference Hibbard1995). Such textures are interpreted as having formed in a dynamic magmatic environment, which facilitated magma interaction on a variety of scales and at different stages between the source and the emplacement levels. However, such a direct evidence of magma interaction is missing in the QMZD and ME rocks, indicating that such a process cannot be invoked as the main process responsible for the genesis of QMZD.
Chemical variability of QMZD, albeit not very large, could be explained by aqueous transport of chemical elements (Alderton, Pearce & Potts, Reference Alderton, Pearce and Potts1980; Kirschbaum et al. Reference Kirschbaum, Martínez, Pettinari and Herrero2005). This hypothesis can be ruled out, however, because the studied rocks and minerals are completely fresh and they lack common alteration products (e.g. sericite, chlorite etc). The observed variations might have been produced if QMZD had strongly interacted with exceptionally REE-rich fluids, but such fluids were missing in the Mt Cer pluton.
Another important issue is the compositional similarity between QMZD rocks and dacites of the Veliki Majdan area (Figs 4–7) that could suggest a similar genesis. In addition, the variation trends and REE patterns given in Figures 4 and 5 show that the Veliki Majdan dacites and QMZD rocks plot between minettes/leucominettes and GDS rocks. This suggests that the dacites and QMZD may have originated by evolutionary processes starting from minettes/leucominettes and/or by interaction processes between minettes/leucominettes and GDS-like magmas.
A closed-system crystal fractionation starting from any given basic magma from the Veliki Majdan area (Prelević et al. Reference Prelević, Foley, Cvetković and Romer2004) cannot be the model for QMZD origin and evolution because of variable isotopic compositions. Fractional crystallization coupled with assimilation (AFC process; De Paolo, Reference De Paolo1981) can explain the variability in isotopic composition, but QMZD rocks have a less crustal-like Sr–Nd isotopic signature (lower 87Sr/86Sr and higher 143Nd/144Nd) in comparison to the Veliki Majdan basic rocks (minettes/leucominettes). Thus a possible contaminant must have very peculiar isotopic compositions, with 87Sr/86Sr lower than 0.707 and 143Nd/144Nd higher than 0.5124, and crustal rocks with such isotopic compositions are unknown in the area.
An alternative hypothesis could be a model of mixing plus fractional crystallization (MFC; e.g. Wei, Congqiang & Masuda, Reference Wei, Congqiang and Masuda1997; Poli & Tommasini, Reference Poli and Tommasini1999; Janousek et al. Reference Janousek, Braithwaite, Bowes and Gerdes2004; Gagnevin et al. Reference Gagnevin, Waight, Daly, Poli and Conticelli2007), with a mathematical formulation analogous to AFC (De Paolo, Reference De Paolo1981). A reasonable approximation for the felsic end-member could be the composition of GDS rocks, whereas an average composition of minettes/leucominettes from the Veliki Majdan area could be a proxy for the mafic end-member. Results of the MFC modelling are presented in Figure 8a, b, showing the variation of 87Sr/86Sr initial ratio versus Sr contents and 143Nd/144Nd initial ratio versus Nd contents, respectively. In the model for Sr isotopes (Fig. 8a), two lines of descent are calculated by varying the values of r (ratio rate of assimilation over rate of crystallization) and DSr (bulk partition coefficient) in the De Paolo (Reference De Paolo1981) equations until a best fit is achieved encompassing the observed variability. The two lines of descent bound a set of models with intermediate DSr values. The results show that the MFC modelling could be considered satisfactory for explaining the petrogenesis of QMZD rocks, even if the calculated values of degree of fractionation are quite high, about 58 % and 60 % for the two lines of descent. The lines of descent calculated using values of the r parameter smaller than 0.5 would require an unrealistically high degree of fractionation (> 0.9). The same procedure has been applied for 143Nd/144Nd (Fig. 8b) and the results show that the MFC process cannot encompass the observed variability, and that using r < 0.5 values produces even worse results.

Figure 8. (87Sr/86Sr)20Ma v. Sr (a), (143Nd/144Nd)20Ma v. Nd (b), and Cr v. Rb (c) diagrams showing the best fit of two MFC lines needed to encompass the variability of composition of the QMZD rocks. Solid square – mafic end-member, average composition of minettes/leucominettes from Veliki Majdan. Star – felsic end member, average composition of GDS rocks. Open square with cross – average composition of minettes, leucominettes, and lamproites. Open square with circle – average composition of lamproites. All mafic magmas from Prelević et al. (Reference Prelević, Foley, Cvetković and Romer2004). Tick marks are reported at 10 % intervals of crystal fractionation; r – rate of mixing over rate of fractional crystallization; D – bulk partition coefficient. Other symbols as in Figure 3. (d) Values of felsic/mafic magma ratios ρ for each QMZD sample and selected elements as calculated using equation 6 from Aitcheson & Forrest (Reference Aitcheson and Forrest1994) for MFC process whose parameters are reported in the text. Solid segment – variation of ρ for Cr, Rb, Sr, Zr, and Nb; dashed segment – variation of ρ for the previous elements and La, Nd, Tb, and Th; solid triangle – Ba; solid circle – Y; solid square – Yb.
It is known that a clear dichotomy exists in the geochemical behaviour of isotopes and trace elements during magma interaction processes, due to sharp differences in diffusion rates. Namely, the isotope diffusion is more than one order of magnitude faster than the trace element diffusion (e.g. Waight, Maas & Nicholls, Reference Waight, Maas and Nicholls2001; Christofides et al. Reference Christofides, Perugini, Koroneos, Soldatos, Poli, Eleftheriadis, Del Moro and Neiva2007). We therefore tried to model the MFC process using trace elements alone. We modelled the lines of descent for 11 elements using Cr contents as the differentiation index. In Figure 8c the plot of Cr versus Rb is reported as an example, and in Table A2 (online Appendix at http://www.cambridge.org/journals/geo) the modelled bulk partition coefficients for all elements are reported. We also calculated the felsic/mafic ratio for each QMZD rock (parameter ρ from Aitcheson & Forrest, Reference Aitcheson and Forrest1994, equation 6; Table A2), and we report ρ values for QMZD rocks in Figure 8d. The ρ values for each rock should be the same for all the elements if the modelling is correct. Although the ρ values are fairly constant for some elements, they are extremely variable for Ba, Y and Yb. This indicates that the MFC process cannot reproduce the variability of QMZD rocks even if we use only trace element concentrations in modelling. It could be suggested that using different mafic and felsic magmas the MFC model could give better results. The compositions of some different possible mafic magmas are reported in Figure 8a–c. It is evident that the modelled results would be even worse for 143Nd/144Nd, due to the higher values of elemental Nd in these mafic magmas, and the same holds for trace elements. For instance, adopting mafic magmas with Cr contents higher than those from the minette/leucominette basic end-member would produce even higher calculated degrees of fractionation. On the other hand, TMG is the only other candidate in the area for representing the composition of the felsic end-member. However, 87Sr/86Sr initial ratios and Sr contents must decrease in the model in order to encompass the compositional variability of QMZD rocks and this is not possible using TMG compositions (De Paolo, Reference De Paolo1981). In conclusion, isotope and trace element geochemistry suggests that MFC is not a likely process for generation of QMZD rocks. This is corroborated by the lack of MME and by the absence of disequilibrium textures in minerals (e.g. Chen, Chen & Jahn, Reference Chen, Chen and Jahn2009).
Given that the QMZD rocks plot between minettes/leucominettes and GDS rocks on many variation diagrams, a simple two end-member mixing process could be proposed. Actually, Prelević et al. (Reference Prelević, Foley, Cvetković and Romer2004) argued that dacites (similar to QMZD in composition) are petrogenetically related to the minette/leucominette rocks, and that the leucominettes are produced by two end-member mixing between a lamproite melt and dacites, although little is said about the genesis of the dacites (e.g. fig. 12 from Prelević et al. Reference Prelević, Foley, Cvetković and Romer2004). It is worth noting that Prelević et al. (Reference Prelević, Foley, Cvetković and Romer2004) found many disequilibrium textures but only in the leucominettes and not in the dacites. Furthermore, they did not find MME in either the leucominettes or the dacites, and they found dacite-derived mineral phases in the leucominettes, but no leucominette-derived mineral phases were found in the dacites.
In order to constrain the simple two end-member mixing process, we applied the binary mixing test of Fourcade & Allègre (Reference Fourcade and Allègre1981). We used the average GDS composition to represent the acid magma composition (Ca), the average composition of minettes/leucominettes to represent the basic magma (Cb), and the average composition of QMZD rocks (Cqmzd) to represent the mixed magma. The plot (Cb − Ca) versus (Cqmzd − Ca) should give a straight line with its slope ranging between 0 and 1 and representing the mass proportions of Ca in the mixture. The fitted line (not shown) calculated using all the elements reported in Tables 2 and 3 has a slope of 0.76 with a R2 = 0.82. These results seem reasonable at first glance, but we need to consider the so-called ‘King Kong effect’ which often occurs when elements with strongly different concentrations are used (Makridakis, Wheelwright & Hyndman, Reference Makridakis, Wheelwright and Hyndman1998, chapter 5). Namely, extreme observations can influence linear correlation between variables masking a deceiving correlation, and acting such as goodness of parameters (e.g. sum of the squared deviations) can be misleading. To overcome this effect we plot the slopes of each element against elements; if correlation is correct all the elements should have the same slope, and this is not the case, as shown in Figure 9a. Moreover, some slopes are outside the permitted values (0–1). It could be argued that using the average composition for QMZD rocks as reflecting the composition of hybrid magma, the correlation could be lost. Therefore, we performed calculations using the composition of every single QMZD rock as reflecting the composition of hybrid magmas, and we report minimum and maximum slope values in Figure 9a. It is evident that all the elements cannot be taken into account for each QMZD sample as the result of a two end-member magma mixing process.

Figure 9. (a) Binary mixing test of Fourcade & Allègre (Reference Fourcade and Allègre1981) for elements reported in Tables 2 and 3 using: the average composition of GDS rocks as acid magma composition, the average composition of minettes/leucominettes as reflecting the basic magma composition, and the average QMZD rocks to represent the mixed magma. Value of each closed square represents the mass proportions of acid magma in the mixture for each element. Dark grey field represents minimum and maximum of mass proportions of acid magma in each QMZD rock, considered as a mixture, for each element. Light grey rectangle is the field of permitted values for the proportions of acid magma according to Fourcade & Allègre (Reference Fourcade and Allègre1981). Selected major element oxide (b) and trace element ratios (c, d) v. Zr. In the insets, companion plots v. 1/Zr are reported. Data for dacites, and minettes/leucominettes are from Prelević et al. (Reference Prelević, Foley, Cvetković and Romer2004).
We plotted major and trace element ratios versus Zr concentrations following figure 12 of Prelević et al. (Reference Prelević, Foley, Cvetković and Romer2004), and some plots are reported in Figure 9b–d. Some elements plot very close to simple binary mixing hyperbolae (e.g. SiO2, Fig. 9b) and their companion plots, against 1/Zr, plot close to the line, indicating that such elements can be modelled by a magma-mixing process (Langmuir et al. Reference Langmuir, Vocke, Hanson and Hart1978). Plots of other elements (e.g. La and Yb, Fig. 9c, d) differ from simple binary mixing hyperbolae and lines, indicating that they cannot be modelled by two end-member mixing. We calculated the correlation coefficients (R2) for all the elements for the companion plots. SiO2 and Al2O3 perfectly follow two end-member mixing trends (0.997 and 0.995), whereas Sr and Rb do not (0.84 and 0.56). LREE plots are strongly scattered (R2 = 0.60) in comparison to HREE (R2 = 0.87).
We believe that such differences in element behaviour give a clue to the genesis of QMZD. Because these differences cannot be due to specific fractionating mineral assemblages (see above), it can be supposed that they resulted from different diffusion coefficients. As general rule, major elements have the lowest diffusion coefficients (~ 10−13 cm2/sec; e.g. Liang, Richter & Watson, Reference Liang, Richter and Watson1996), whereas Rb and Sr have the highest diffusion coefficient (~ 1.7*10−7 cm2/sec at 1500 MPa; Nakamura & Kushiro, Reference Nakamura and Kushiro1998). In addition, LREE have higher diffusion coefficients (La: 1.1*10−8 cm2/sec at 1500 MPa; Nakamura & Kushiro, Reference Nakamura and Kushiro1998) than HREE (Lu: 7*10−8 cm2/sec at 1500 MPa; Nakamura & Kushiro, Reference Nakamura and Kushiro1998). These findings imply that diffusion played an important role in the petrogenesis of QMZD, and that diffusion was probably controlled by specific conditions during magma interaction processes. When a felsic magmatic mass overlies a mafic one, the two magmatic masses tend to remain separated at the very beginning, because of the density contrast, but this does not preclude chemical exchanges taking place. As stated by Snyder & Tait (Reference Snyder and Tait1998) and Christofides et al. (Reference Christofides, Perugini, Koroneos, Soldatos, Poli, Eleftheriadis, Del Moro and Neiva2007), a convection–diffusion process could influence the concentration of isotopes and elements having the highest diffusivity. 87Sr/86Sr and 143Nd/144Nd have the highest diffusion coefficients (e.g. Lesher, Reference Lesher1990), whereas these coefficients are progressively lower for trace and major elements, the latter displaying the lowest diffusivity (e.g. Waight, Maas & Nicholls, Reference Waight, Maas and Nicholls2001). The reason for this behaviour lies in the fact that diffusion of major elements is controlled mostly by diffusivity of Si and Al, which are network-forming cations. This means that these elements may diffuse only by restructuring the melt, which is difficult to achieve. On the other hand, diffusion of isotopes and trace elements does not require the melt restructuring and, therefore, the mobility of these elements in the melt is much higher. Quartz monzonite/quartz monzodiorite rocks have geochemical characteristics such that major element contents, particularly SiO2 and Al2O3, perfectly follow two end-member mixing trends (Fig. 9b), whereas trace element (Fig. 9c, d) contents and isotope ratios (Fig. 7) show a lot of scatter. During magma interaction processes, diffusion is further enhanced by convection in each of the two magmas caused by their thermal differences. This process of convection–diffusion may thus change the chemical composition in the felsic magma even in the overt absence of mixing.
During such processes, thermal and rheological contrasts between the mafic magmatic mass and the overlying felsic one are progressively smoothed. These new conditions change the nature of interaction in which magma mixing becomes more important and higher efficiency of mixing is enabled (e.g. Sparks & Marshall, Reference Sparks and Marshall1986; Poli, Tommasini & Halliday, Reference Poli, Tommasini and Halliday1996).
The above discussion suggests a scenario for the formation of QMZD in which a felsic magmatic mass having composition similar to GDS rocks was overlying a mafic magmatic body similar in composition to minettes/leucominettes. At the beginning, a convection–diffusion process influenced the elements of the highest diffusivity. In such a way, the GDS magma was driven to change the concentrations of Sr, Rb and REE, but not the contents of major elements. When the contrasts in composition between the two magmas decreased, a simple two end-member mixing occurred, causing a change of the concentrations of all the elements. In this way the elements not affected by the convection–diffusion process followed a clear two end-member mixing trend (Fig. 9b), whereas those modified by this process have scattered trends (Fig. 9c, d). The lack of mafic microgranular enclaves and disequilibrium textures in mineral phases in the produced melts (QMZD and dacites) is consistent with the proposed scenario. Namely, the mafic magma acted only as a chemical contaminant during the convection–diffusion process, whereas an almost complete two end-member mixing excluded the possibility of disequilibrium textures being produced or physical dispersion of magmas into each other. However, the process itself does not completely preclude having a few larger mafic microgranular enclaves, such as that found in the southern part of the pluton.
The scenario outlined above is different from many interpretations of mixing processes (e.g. Bateman, Reference Bateman1995; Karsli et al. Reference Karsli, Chen, Aydin and Sen2007). The explanations could be found in the fact that this convection–diffusion process followed by two end-member magma mixing occurred at pressures higher than 600 MPa, as suggested by an amphibole geobarometer and by the presence of weak thermal metamorphism effects at the emplacement level of QMZD. From 30 Ma to 15 Ma, basaltic (sensu lato) magmatism took place in the intermediate/lower crust (e.g. Cvetković et al. Reference Cvetković, Prelević, Downes, Jovanovic, Vaselli and Pécskay2004; Prelević et al. Reference Prelević, Foley, Romer, Cvetković and Downes2005) causing an increase of isotherms in the area and providing conditions for partial melting of intermediate and lower crust (see below; e.g Annen, Blundy & Sparks, Reference Annen, Blundy and Sparks2006). Perugini & Poli (Reference Perugini and Poli2005) showed that the initial stages of magma interaction, when the mafic magma enters the felsic magma chamber, are governed by viscous fingering dynamics. The morphology of interfaces between mafic and felsic magma depends on relative viscosity. At low viscosity ratios, the interface is roughly plain, preventing fountain-like interactions and effective mixing. At high viscosity ratios, finger-like patterns are generated and they usually enhance mixing processes between the low-viscosity mafic magma and the high-viscosity felsic magma. The viscosity ratio for the two interacting magmas in the proposed model has been calculated assuming different crystal percentages and pressure of 2000 MPa (Shaw, Reference Shaw1972; Bottinga & Weil, Reference Bottinga and Weill1972). Under the worst conditions, that is, with a crystal-free mafic magma and 50 % crystallized felsic magma, the viscosity ratio is only about 3 × 102. On the other hand, the high temperature in the area would depress nucleation and crystallization of magmas, and would increase temperature in the felsic magma, so the viscosity ratios would be even lower, prohibiting extensive magma mixing (see fig. 4 of Perugini & Poli, Reference Perugini and Poli2005). This supports the hypothesis that initial hybrid QMZD magmas may have resulted mainly from convection–diffusion rather than magma-mixing processes, whereas the latter processes subsequently became more important.
Once the QMZD magmas formed, they ascended and were emplaced, producing a weak contact metamorphism. They started to crystallize at about 500 MPa, and continued to ascend up to 300–400 MPa according to pressures reported in Figure 3b. Cognate ME started to be generated at about 500 MPa with maximum formation at about 400 MPa and 200–300 MPa (Fig. 3b). The nearly continuous pressure of formation of QMZD and ME was caused by uplift and erosion during the opening of the Pannonian Basin. The age of these processes can be constrained by data reported in Table 1. Quartz monzonite/quartz monzodiorite magmas were emplaced earlier than c. 21 Ma, and a minimum age of this emplacement is obtained on hornblende. The final crystallization of QMZD magmas probably occurred earlier than c. 17 Ma, assuming that the K/Ar age data on biotites represent the cooling age. The above considerations suggest that a time span between the emplacement and final crystallization of QMZD was about 4 Ma. During this time the QMZD body was continuously ascending. Assuming blocking temperatures for 40K–39Ar of amphibole and biotite of 500–600 °C and 300–350 °C, respectively, the pluton cooled at a rate of about 40–75 °C/Ma. Such a rate is consistent with calculated rates from various plutons (e.g. Pitcher, Reference Pitcher1997, chapter 12; Long, Castellana & Sial, Reference Long, Castellana and Sial2005).
4.b. GDS
The Stražanica granodiorite/quartz monzonite rocks show silica contents ranging between 67.5 and 70.5 wt % and are characterized by the least crustal-like Sr–Nd isotopic signature among all the Mt Cer rocks. This indicates that they cannot be products of low-pressure processes of evolution starting from any of the less evolved magmas present in the area. Besides, the above discussed origin of QMZD implies that GDS originated by partial melting of intermediate/lower crust. The relatively low Sr and high Nd isotopic ratios strongly narrow the possibility that GDS rocks originated by partial melting of upper crustal rocks, unless a very young upper crust is involved, for which there is no geological evidence (e.g. Cvetković et al. Reference Cvetković, Poli, Christofides, Koroneos, Pecskay, Resimic-Saric and Eric2007). In addition, GDS are mainly metaluminous or slightly peraluminous rocks, and this excludes an Al-rich crust as a potential source. In Figure 10 the composition of GDS rocks is plotted along with data of melt compositions obtained by melting experiments of various crustal protoliths. The graph shows that GDS samples are compositionally similar to the melts derived from metabasalts and amphibolites, and depart from those produced by melting of greywackes and pelites.

Figure 10. ASI/10–CaO–5*K2O (molar), and ASI/10–CaO–MgO/SiO2 (molar) triangular diagrams displaying the composition of the GDS rocks compared with experimental data of melt compositions from different crustal protoliths at 1500 MPa. References for the various sources are reported in online Appendix at http://journals.cambridge.org/geo.
Melts with major-element compositions similar to GDS rocks can be produced by fluid-absent partial melting of metabasaltic rocks under a wide variety of conditions (e.g. Clemens, Reference Clemens, Brown and Rushmer2006). Many authors have studied pressure and temperature ranges, and residual assemblage of partially melted amphibolites and coexisting residual phases (e.g. Sen & Dunn, Reference Sen and Dunn1994; Rapp & Watson, Reference Rapp and Watson1995; Clemens, Yearron & Stevens, Reference Clemens, Yearron and Stevens2006). Garnet can be considered stable at P ≥ 1.5 GPa, and its stability is mostly temperature-independent, whereas the amount of garnet in the restite is controlled by both temperature and presence of plagioclase in the protolith. At pressures P ≥ 1.5 GPa (garnet is present), the modal amphibole/clinopyroxene ratio in the residuum mainly depends on temperature, chemical composition, and mineral assemblage of the protolith.
Fractionated HREE (Fig. 5), and low absolute concentrations of Yb and Y in GDS rocks (Table 2) strongly suggest that garnet was present as a residual phase in the source of GDS magmas, and this implies pressures higher than 1.5 GPa. Relatively high Ba/Th ratios (~ 115; Fig. 6) are not consistent with the only presence of residual amphibole in the source, due to the preferential retention of Ba in amphibole. Hence, both amphibole and clinopyroxene must have been present as residual phases. High contents of Sr and weak or no Eu-anomaly indicate that plagioclase was not an important restitic phase. Consequently, a residual assemblage composed of amphibole + clinopyroxene + garnet ± plagioclase was likely left behind during the partial melting process that generated GDS magmas. The contents of other trace elements provide some additional constraints on the residuum mineralogy. The presence of negative Ta–Nb, P and Ti anomalies (Fig. 6), for instance, is consistent with the presence of residual rutile and apatite.
To evaluate whether partial melting of an amphibolitic source leaving behind such a residuum is a likely explanation for the petrogenesis of GDS, trace element modelling has been applied. We used subduction-modified lower crust having the average composition of more mafic (Mg no. > 60) continental andesites (Kelemen et al. Reference Kelemen, Hanghøj, Greene, Heinrich, Karl and Rudnick2007) to represent the composition of the lower crust in the northern Dinarides (Table 5). Given that the source mineral assemblage is difficult to envisage, we used the following formulation for a non-modal batch melting model (Rollinson, Reference Rollinson1993):

where C 0 and Cl are the concentrations of a given trace element in the source and melt, respectively, Dr is the bulk partition coefficient of the residual solid, and F is the weight fraction of melt produced.
Table 5. Range of trace element abundances in GDS rocks and source used in the partial melting model

*Data for source are from Kelemen et al. (Reference Kelemen, Hanghøj, Greene, Heinrich, Karl and Rudnick2007). Bulk partition coefficients used to model GDS rocks. Residuum assemblages calculated using linear programming (Wright & Doherty, Reference Wright and Doherty1970) minimizing the sum of squares of residuals as a constraint (SSR). Mineral abbreviations are after Kretz (Reference Kretz1983).
In Figure 11 the best model is shown as a spider diagram normalized to the source for degree of melting of 0.3. There is a remarkable match for all the elements between modelled melt and GDS. Modelled bulk partition coefficients and percentages of residual minerals are given in Table 5. The percentage of residual minerals has been calculated by linear programming, minimizing the sum of squares of residuals as a constraint (SSR; e.g. Wright & Doherty, Reference Wright and Doherty1970) starting from the bulk partition coefficient modelled and using literature partition coefficients (Table 5; GERM data set, http://earthref.org/GERM/main.htm).

Figure 11. Source-normalized spider diagram of minimum and maximum of reported elements in the GDS rocks from Mt Cer, together with the modelled melt after 0.3 degree of melting of the Arc Andesite source reported in Table 5.
Degree of melting (F = 0.3) is consistent with the supposed presence of amphibole and clinopyroxene in the residuum (e.g. Rapp & Watson, Reference Rapp and Watson1995). The presence of mica is necessary to account for Rb and Ba contents. A subduction-modified lower crust has higher Rb and Ba abundances relative to a MORB-like source (e.g. GERM, http://earthref.org/GERM/main.htm), and this is a further indication of the presence of residual mica. On the other hand, Rapp & Watson (Reference Rapp and Watson1995) argued that biotite is present at 800 MPa and 1000 °C for the most potassium-rich source (N°1 alkali rich basalt, K2O = 0.82 in Rapp & Watson, Reference Rapp and Watson1995). The source used for the present model contains double that value; it is thus reasonable to assume that mica could be a residual phase even at higher pressures. The presence of small amounts of plagioclase is consistent if melting temperature is below 1025 °C at 1600 MPa (Rapp & Watson, Reference Rapp and Watson1995). On the basis of the above discussion, it can be concluded that partial melting of lower crust having an average composition of mafic continental andesites can produce magmas which are geochemically similar to GDS. It is noteworthy that the presence of small amounts of plagioclase accounts also for the peculiar behaviour of Sr in QMZD (Fig. 4d). The Sr contents in melts are controlled by plagioclase, and because this phase is not strongly enriched in the restite, Sr contents in the produced GDS melt are quite high.
4.c. TMG
Two-mica granites are the most evolved rocks of the Mt Cer pluton and have a peraluminous character. In terms of mineral chemistry and major and trace element geochemistry, they are similar to the two-mica rocks from Mt Bukulja pluton, but the Mt Cer TMG have higher initial 87Sr/86Sr and lower 143Nd/144Nd isotope ratios. In Figure 12 the composition of the two-mica granites from the Mt Cer and Mt Bukulja plutons are plotted together with the composition of basement rocks. The latter were considered by Cvetković et al. (Reference Cvetković, Poli, Christofides, Koroneos, Pecskay, Resimic-Saric and Eric2007) as the contaminant in an AFC model of the evolution of the Mt Bukulja TMG. It is clear that Mt Cer TMG plot in continuity with Mt Bukulja TMG rocks, suggesting a similar evolution. Such a hypothesis has been tested using the approach of Cvetković et al. (Reference Cvetković, Poli, Christofides, Koroneos, Pecskay, Resimic-Saric and Eric2007) for a set of trace elements and the modelled bulk partition coefficients (Fig. 12). Two evolution lines are used for encompassing the TMG variability, at a constant value of r = 0.4 (De Paolo, Reference De Paolo1981). Fractionating assemblages have been calculated by linear programming, minimizing the sum of squares of residuals as a constraint (SSR; e.g. Wright & Doherty, Reference Wright and Doherty1970), starting from the bulk partition coefficient modelled and using literature partition coefficients (GERM dataset, http://earthref.org/GERM/main.htm). Calculated fractionating assemblages are similar for the two models AFC1 and AFC2, and are roughly dominated by quartz (~ 40 %), plagioclase (~ 35 %), K–feldspar (~ 8 %) and biotite (~ 13 %). In comparison to the AFC model for TMG rocks from the Mt Bukulja pluton, a greater amount of feldspars is necessary to reproduce the observed geochemical trends. This is in agreement with lower mean contents of Sr in the Mt Cer TMG (100 ppm) relative to Mt Bukulja TMG (150 ppm). In addition, the increase of feldspar in the fractionating assemblage is inferred by the high Eu anomaly in the Mt Cer TMG rocks (Fig. 6). The amount of accessory minerals (apatite 1.3 %, titanite 0.25 % and zircon 0.15 %) is more or less similar for the Mt Bukulja and Mt Cer models, although more zircon and a very small amount of allanite (0.05 %) are necessary to reproduce the geochemistry of Mt Cer TMG. These slight differences may have resulted from local changes in the magma chamber and in the contaminant.

Figure 12. (87Sr/86Sr)20Ma v. (143Nd/144Nd)20Ma showing the two AFC models (AFC 1 and AFC 2) needed to encompass the variability of composition of the Mt Cer TMG. Modelled bulk partition coefficients D for Sr and Nd, and for a series of trace elements for the two models are also given. Parental end-member and contaminant are the average composition of most primitive TMG rocks and sample BK136 from the metamorphic basement, respectively. Both end-members are from the Mt Bukulja pluton (Cvetković et al. Reference Cvetković, Poli, Christofides, Koroneos, Pecskay, Resimic-Saric and Eric2007). Tick marks are reported at 10 % intervals of crystal fractionation.
5. Geodynamic constraints
The Mt Cer pluton, along with the adjacent Mt Bukulja pluton, has a specific geotectonic position. Both plutons are situated at the junction between the southern Pannonian Basin and northern Dinarides. The foregoing discussion has shown that the Mt Cer pluton consists of I- and S- type intrusions that show differences in age and composition and thereby reflect important differences in their source, evolution and geodynamic conditions.
The age of the Mt Cer pluton is not completely constrained, but there is evidence that the complete consolidation of QMZD and TMG occurred not later than 17 Ma and 16 Ma, respectively, whereas that of GDS is similar to QMZD at c. 17 Ma. Therefore, it is very likely that the Mt Cer pluton is genetically unrelated to widespread Oligocene granitoid bodies which can be, with some gaps, traced from the Periadriatic line southeastwards (Fig. 1; e.g. Laubscher, Reference Laubscher1983; Elias, Reference Elias1998; Rosenberg, Reference Rosenberg2004). These plutons in Serbia stretch roughly NW–SE, and follow a belt of elongated Oligocene lacustrine depositional systems. These lacustrine basins are interpreted as having resulted after stabilization of the Dinaride orogen and during dextral strike-slip wrenching tectonics (e.g. Brunn, Reference Brunn1960; Marović et al. Reference Marović, Krstić, Stanić, Cvetković and Petrović1999, Reference Marović, Mihailović, Đoković, Gerzina and Toljić2001). Studies of basaltic and potassic/ultrapotassic rocks from the central Balkan Peninsula (Cvetković et al. Reference Cvetković, Prelević, Downes, Jovanovic, Vaselli and Pécskay2004; Prelević et al. Reference Prelević, Foley, Romer, Cvetković and Downes2005) imply a major role for the post-collisional orogenic collapse of the Dinarides, and such an interpretation can likely be valid for the origin of temporally and spatially related granitoid rocks. Similar processes have already been recognized in other orogenic systems (e.g. England & Houseman, Reference England and Houseman1985; Ratschbacher et al. Reference Ratschbacher, Frisch, Neubauer, Schmid and Neugebauer1989) where overburden gives rise to convective thinning, lithosphere instabilities and post-collisional collapse.
The Mt Cer composite pluton has an age range similar to that shown by granitoids occurring mostly along the northern Dinarides/southern Pannonian realm (e.g. Cvetković et al. Reference Cvetković, Poli, Christofides, Koroneos, Pecskay, Resimic-Saric and Eric2007; Fodor et al. Reference Fodor, Gerdes, Dunkl, Koroknai, Pécskay, Trajanova, Horváth, Vrabec, Jelen, Balogh and Frisch2008). During the Palaeogene to Neogene tectonic regime, dominated by orogen-parallel wrenching, the final formation of a double vergent Dinaride–Carpathian–Balkan orogen occurred. The subsequent tectonic style was generally related to the formation of the Pannonian Basin. This tectonic event was combined with the Neogene indentation of the Apulian microplate which formed nap-stacking in the External Dinarides (Karamata, Reference Karamata, Robertson and Mountrakis2006; Schmid et al. Reference Schmid, Bernoulli, Fügenschuh, Matenco, Schefer, Schuster, Tischler and Ustaszewski2008; Robertson, Karamata & Sarić, Reference Robertson, Karamata and Sarić2009). This is contemporaneous with, and most probably tectonically related to, the continuation of dextral orogen-parallel movements along the major axis of the Balkan Peninsula and to the extension in the Pannonian area.
Ilić & Neubauer (Reference Ilić and Neubauer2005) performed a palaeostress analysis in the central Dinarides, some 200 km south of Belgrade. They reported mainly NW-trending Neogene normal faults, which indicate Early/Middle Miocene NE–SW extension related to syn-rift extension in the Pannonian basin. This suggests that the events genetically related to the Pannonian extension can be traced southwards deep in the Dinarides and that they are more apparent along their northern margin.
Our study revealed that the composite Mt Cer pluton originated after complex and multistage magmatic events involving melting of intermediate–lower crustal protoliths and evolution through convection–diffusion, magma mixing and AFC processes. It is suggested that the origin and evolution of QMZD and GDS occurred at the base of the continental crust. The present-day thickness of the continental crust across the Vardar suture is estimated at about 30 km (Aljinović, Reference Aljinović1987). However, straddling the Oligocene–Miocene boundary the continental crust must have been relatively thicker, reaching ~ 50 km, corresponding to pressures as high as 1700 MPa, having been subsequently reduced during the Dinaride wrenching and even more during the Middle Miocene Pannonian extension. Partial melting at such depths could have produced GDS-like magma that interacted with underlying minette-type magma by a convection–diffusion process. The peraluminous character and the AFC-dominated evolution of TMG imply a progressively more important role of higher levels of continental crust in the succession of these tectonomagmatic events. A significant age difference between QMZD, which started to crystallize deep in the crust, and TMG which shows sharp intrusive contacts with the former, indicates that the origin and evolution of the composite Mt Cer pluton is related to an increase of regional heat flow. This is consistent with a transition from a dextral transpression–transtension, at the beginning of Miocene times, to a normal fault-dominated tectonic style, which occurred around 4–5 Ma later.
Schematic cross-sections of the mantle–crust transition along the southern margin of the Pannonian Basin in northern Serbia during Tertiary times are shown in Figure 13. Underplating of mantle-derived magmas with high-K calc-alkaline to ultrapotassic affinities started at c. 35 Ma (Fig. 13a) and continued up to the end of Oligocene and beginning of Miocene times (e.g. Prelević et al. Reference Prelević, Foley, Romer, Cvetković and Downes2005). The underplating produced high heat flow in the lower crust that was able to induce partial melting of amphibolitic/andesitic lower crust and production of GDS-like magmas. According to our model, such magmas were underlain by minette/leucominette-like magmas (Fig. 13b). This contact first led to a convection–diffusion process influencing elements of high diffusivity, such as Sr, Rb, La and Th, but not the major elements. When the contrasts in physical parameters between the two magmas decreased, a simple two end-member mixing occurred, producing QMZD-like magmas (Fig. 13c). Such magmas were emplaced at crustal levels corresponding to about 500 MPa, where they started to crystallize producing weak thermal metamorphism (Fig. 13d). Simultaneously, some cognate mafic enclaves were formed. The Pannonian extension caused erosion events in the marginal areas, with the consequence that QMZD was uplifted to 300–400 MPa, where it continued to crystallize and finally solidified (Fig. 13e). In the meantime, a predominance of cognate enclaves formed and batches of GDS-like magma were emplaced at the same crustal level, causing strong contact-metamorphic effects (Fig. 13e). Further opening of the Pannonian Basin caused decompressional partial melting of intermediate crust, which resulted in the genesis of the least evolved TMG magmas, which successively experienced AFC processes (Fig. 13e). Two-mica granite-like magmas were emplaced as a magmatic body, such as the Mt Bukulja pluton, and as veins and dykes intruding the main QMZD body, in the case of Mt Cer pluton. These magmatic intrusions were emplaced at about the 300–400 MPa level, producing strong contact metamorphic effects in the case of the Mt Bukulja pluton (Fig. 13e), whereas the present day outcrop level resulted from subsequent erosion processes (Fig. 13f).

Figure 13. Schematic cross-sections of the mantle–crust transition along the southern margin of the Pannonian Basin in northern Serbia during Tertiary times, showing episodes of underplating, partial melting, plutonism and extension (see text for details). Not to scale. Crust thickness is at least 50 km up to 20 Ma, at the onset of the opening of the Pannonia basin. Present crust thickness in the area is about 30 km.
Acknowledgements
We have dedicated this paper to late Prof. Vera Knežević-Đorđević, whose nearly four decades of research contributed greatly to the understanding of the origin of Serbian granites. We are grateful to N. Bonev, B. Bonin, two anonymous referees and editor D. Pyle for critical reviews and helpful comments, which greatly improved the manuscript. The authors thank Dejan Prelević and Diego Perugini for critical reading of early versions of the paper. We are very grateful to R. A. Creaser, Department of Earth and Atmospheric Sciences, University of Alberta, Canada, for Sr and Nd isotope analyses. This study was supported by a Scientific Exchange Programme between the Aristotle University of Thessaloniki and University of Belgrade and by the Project 146013 of the Serbian Ministry of Science and Technological Development (VC). Research was also financially supported by Italian MIUR and University of Perugia funds to GP. A grant to GC from European Commission TMR Programme – Geochemical facility was used for REE analyses in the Department of Earth Sciences, University of Bristol (UK).