Introduction
Coal seam-intercalated clay layers formed during burial diagenesis of pyroclastic rocks in a swampy lacustrine environment in San Miguel Mine, Atascosa, Texas, USA (Senkayi et al., Reference Senkayi, Ming, Dixon and Hosner1987). Argillaceous units in the Miocene coal fields (Seyitömer, Tunçbilek, Soma, and Harmancık) have intercalated tuffs in northwest–west Anatolia (Çelik et al., Reference Çelik, Karayiğit, Oskay, Kayseri Özer, Christanis, Hower and Querol2021; Erkoyun et al., Reference Erkoyun, Kadir, Külah and Huggett2017, Reference Erkoyun, Kadir and Huggett2019; Karayiğit et al., Reference Karayiğit, Littke, Querol, Jones, Oskay and Christanis2017; Oskay et al., Reference Oskay, Bechtel and Karayiğit2019). Smectitic bands were formed generally from altered volcanic ash layers having rhyolitic to dacitic composition in the Sydney Basin, Australia (Rivas-Sanchez et al., Reference Rivas-Sanchez, Alva-Valdivia, Arenas-Alatorre, Urrutia-Fucugauchi, Ruiz-Sandoval, Roberts and Loughnan1989), in Songzao, China (Zhao et al., Reference Zhao, Ward, French and Graham2012), in western Washington, USA (Bohor & Triplehorn, Reference Bohor and Triplehorn1993), and Alaska, USA (Reinink-Smith, Reference Reinink-Smith1990). The alteration of tuffs to smectite was due possibly to the occurrence of Mg and Ca which were not flushed away fully in a closed system during diagenesis (Bohor & Triplehorn, Reference Bohor and Triplehorn1993). Furthermore, detrital smectite in the fluvial-lacustrine units (organic-rich shale, mudstone, and sandstone) associated with early diagenetic coal seams had a terrigenous origin in Spain (Sáez et al., Reference Sáez, Inglès, Cabrera and de las Heras2003) and Bulgaria (Yossifova et al., Reference Yossifova, Dimitrova and Ivanova2018).
Detrital smectite is abundant in terrigenous fluvial sediments such as organic-rich shale, sandstone, mudstone, and, locally, in tuff in the Seyitömer coal deposit (Erkoyun et al., Reference Erkoyun, Kadir, Külah and Huggett2017). In addition, the authigenic formation of the tonstein kaolin in the Tunçbilek coal deposit was from volcanic ash during diagenesis and was determined in detail (Erkoyun et al., Reference Erkoyun, Kadir and Huggett2019). No detailed study of the mode of formation of smectite in the Seyitömer and Tunçbilek coal deposits in western Anatolia has been reported.
The Orhaneli and Keles coal mines are the most important source of raw materials for heat energy, electricity, and mechanical energies in western Anatolia and have reserves of 102 million tons (Şengüler, Reference Şengüler2004). The Neogene fluvial-lacustrine sediments include pyroclastics in the Orhaneli-Keles basins.
The Orhaneli and Keles coal mines have been studied previously for the purpose of coal prospecting (Bayraktar & Altınay, Reference Bayraktar and Altιnay1985; Bilgin et al., Reference Bilgin, Aksoy and Dost1988; Çetin, Reference Çetin1985; Yiğitel et al., Reference Yiğitel, Altιnay and Özcan1989); the studies were restricted to petrographic, mineralogical, and sedimentological characterization of coal (Çelik et al., Reference Çelik, Karayiğit, Oskay, Kayseri Özer, Christanis, Hower and Querol2021). Prior to the current study, no detailed information was available concerning the mineralogy, micromorphology and geochemistry, palynology, and formation conditions of the smectite associated with intercalated argillaceous sediments and volcanogenic units in coal seams in the Orhaneli and Keles coal mines. Thus, the purpose of the current study was to fill this gap and unravel the mineralogical and geochemical evolutionary stages of the lacustrine deposits and the origin of the smectites. These results have also helped to understand the fluvial deposition, the paleoclimatic conditions, and the diagenetic environment for the precipitation of smectitic claystone which intercalates the coal seams in a fault-controlled system and the local influence of hydrothermal fluid.
Geological Setting
Basement rocks of the study area comprise Mesozoic metamorphics and Upper Cretaceous ophiolitic mélange (Fig. 1). The Mesozoic metamorphic rocks are Triassic mica-schist, phyllite, metabasite, and marbles; and are overlain conformably by Triassic–Cretaceous marbles and locally recrystallized limestones (Okay et al., Reference Okay, Harris and Kelley1998, Reference Okay, Nobble and Tekin2011). The Mesozoic metamorphic rocks are overlain tectonically by an Upper Cretaceous ophiolitic mélange which consists of ultramafic rocks, basalt, meta-clastics, and marbles (Sarıfakıoğlu et al., Reference Sarιfakιoğlu, Özen and Winchester2009; Şengüler, Reference Şengüler2004). The basement units were intruded by Eocene Büyükorhan, Topuk, Göynükbelen, and Gürgenyayla granitoids (Başol, Reference Başol2009). During the Miocene, the region was influenced by an extensional tectonic regime, dominated by normal faulting, which led to the occurrence of numerous basins controlled by a ~ N–S-trending graben system (Selim et al., Reference Selim, Tüysüz and Barka2006; Şengüler, Reference Şengüler2004). These depression basins were filled by Miocene–Pliocene lacustrine sediments (Şengüler, Reference Şengüler2004). The Orhaneli and Keles areas represent two different basins, which are separated by a threshold (horst) of basement rocks which occurred in the same tectonic system (Şengüler, Reference Şengüler2004). In these basins, Miocene–Pliocene continental sediments overlie unconformably the basement rocks and Eocene granitoids (Fig. 2). Miocene sediments are composed of conglomerate, sandstone, mudstone, argillaceous sediments, marl, and organic-rich shale with coal seams, tuff/tuffite, and limestone deposited in a lacustrine environment. Lacustrine sediments have lateral and vertical transitions with Miocene volcanic and volcaniclastic units, showing an andesitic to dacitic composition. Miocene formations are overlain conformably by Pliocene continental sediments and volcano-sedimentary units. The Pliocene is represented by limestones, marls, tuff/tuffite, and has a total thickness of ~ 300 m in the Miocene units in the Keles area (Figs. 1 and 2; Şengüler, Reference Şengüler2004). All the aforementioned units are overlain unconformably by Quaternary alluvium.

Fig. 1 Location and geological maps of the coal-bearing Orhaneli-Gümüşpınar and Keles-Harmanalan basins and sample locations (Konak, Reference Konak2002; Özaksoy et al., Reference Özaksoy, Elmacι, Özalp, Kara and Duman2018)
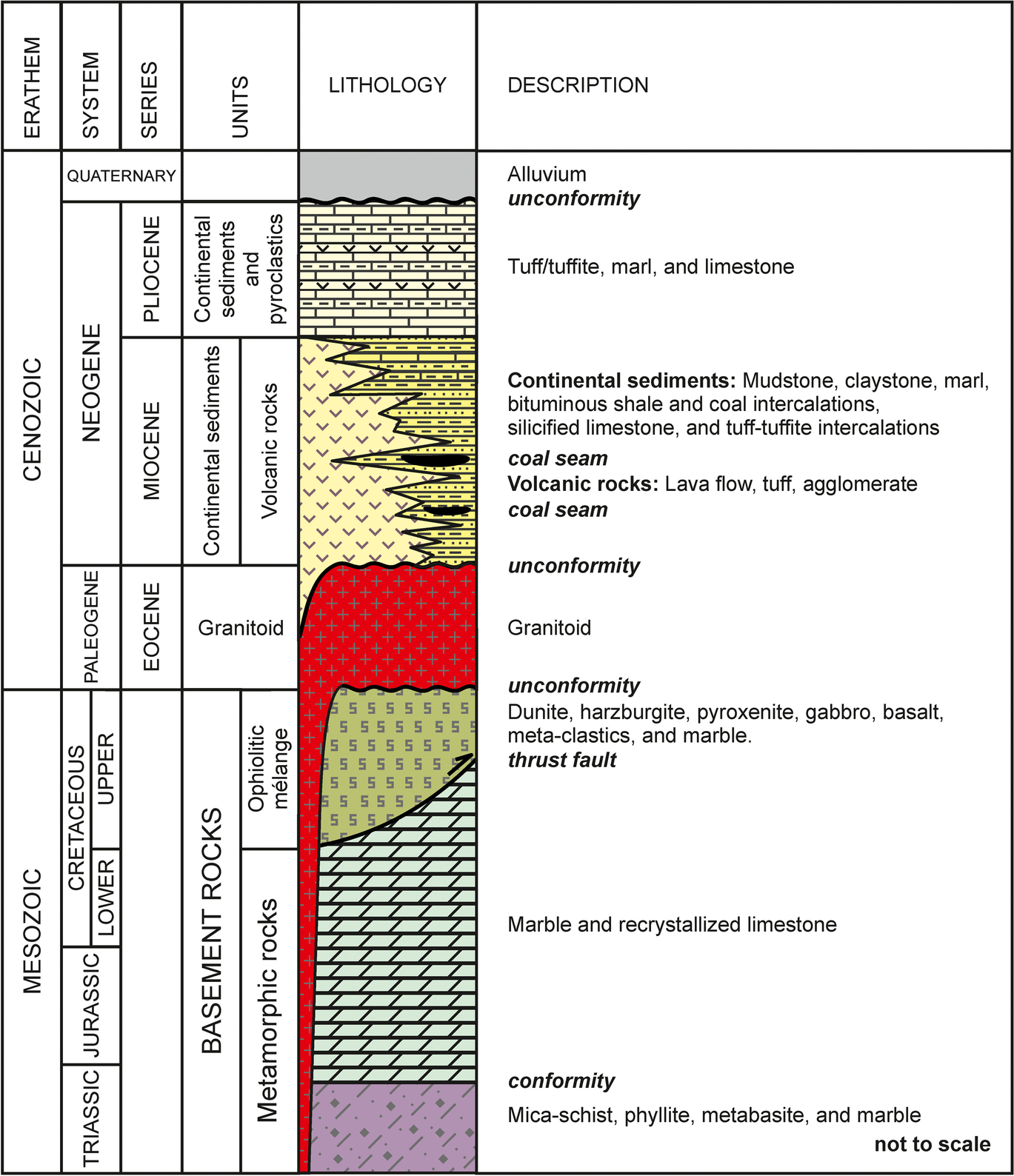
Fig. 2 Stratigraphic section of the study area (modified from Okay et al., Reference Okay, Harris and Kelley1998)
Description of Coal-bearing Sections
Orhaneli-Gümüşpınar Region
A6 section
This section begins with greenish-gray colored, locally coarse sand-fine gravel bearing argillaceous sediment and continues upward with a dark brown, fractured, and friable coal seam (Figs. 3 and 4a). The coal seam in this section is 8 m thick and contains 15 cm-thick layers of marl. The coal seam is overlain by a 40 m-thick, greenish-gray, plastic, conchoidally fractured, argillaceous sediment which contains pyrite crystals (Fig. 4b) and 1–2 m-thick dark brown, organic-rich shale levels. The middle part of this section is intercalated with 1 m thick conglomerate and 4 m thick sandstone, respectively. This section continues upward with light brown, thick-bedded (~ 1 m) mudstone, and beige tuff that is composed of biotite and quartz crystals, and pumice fragments. This section is overlain by beige, 10 m thick tuff that is intercalated with gray, thin-bedded (20–30 cm) marl.
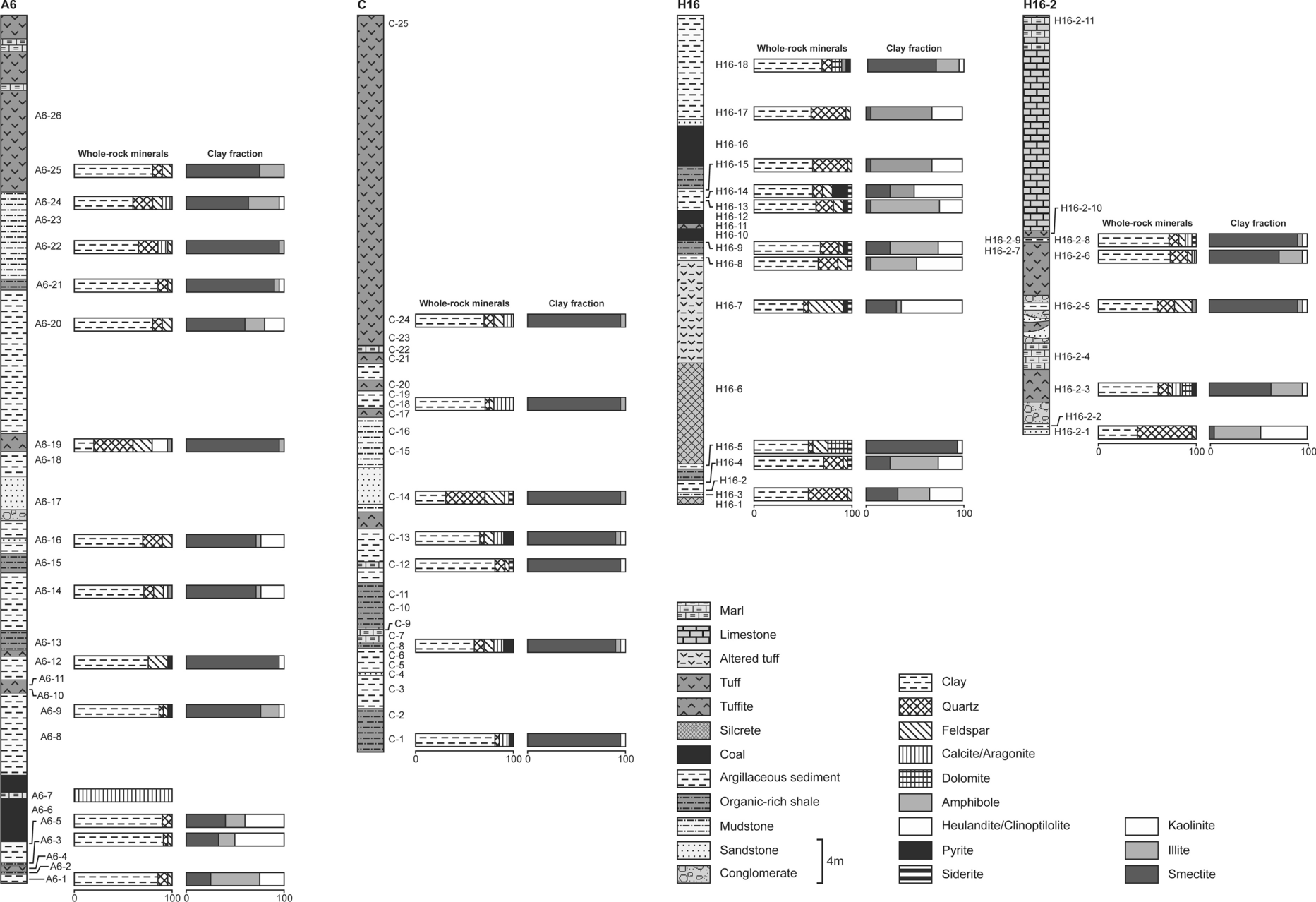
Fig. 3 Graphical log of the Orhaneli-Gümüşpınar (A-6 and C) and Keles-Harmanalan (H16 and H16-2) basins, and the abundance of clay fraction and whole-rock minerals based on XRD results
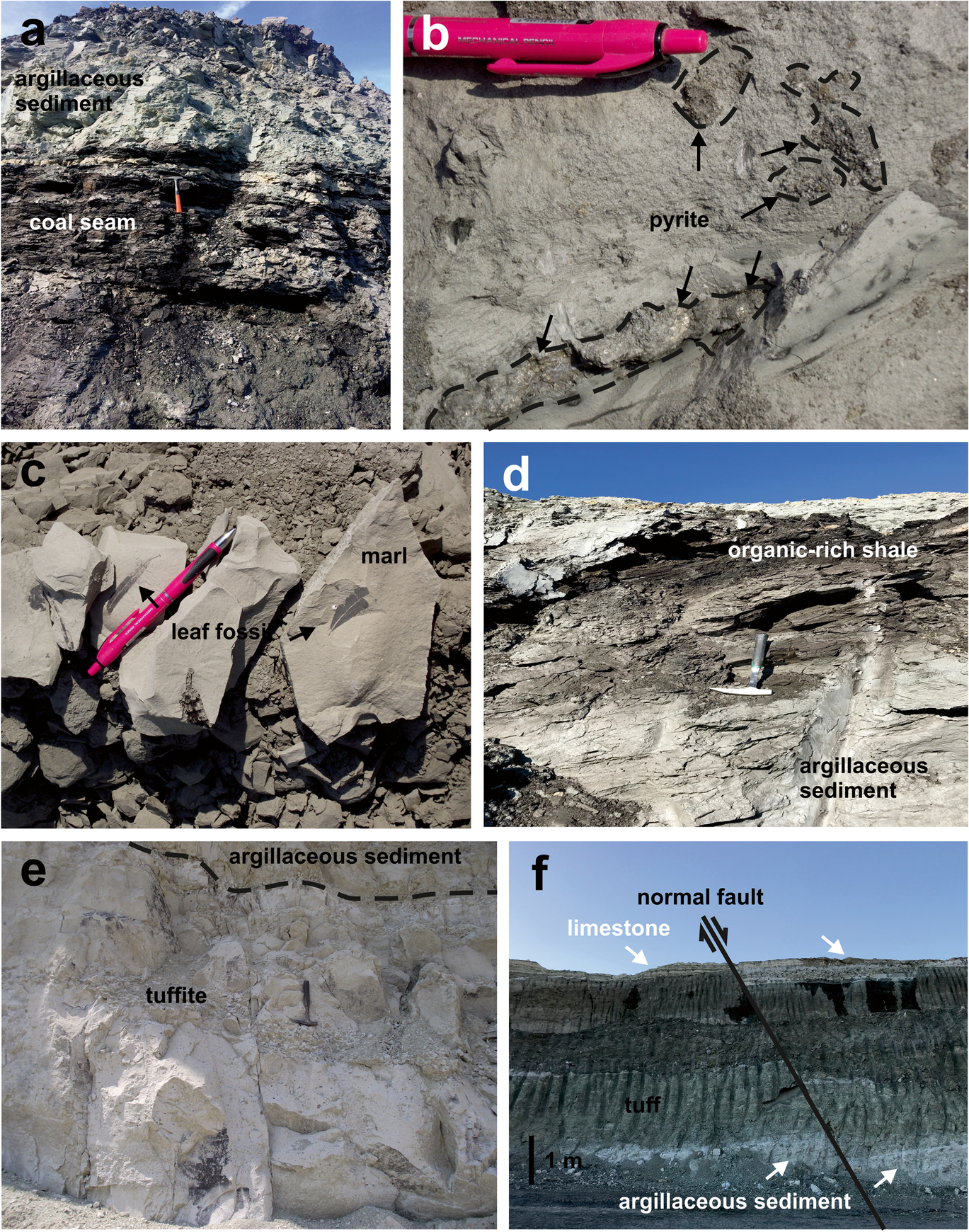
Fig. 4 Field photographs: a Geometrical relationship between coal seams and argillaceous sediment in the A6 section; b pyrite within the organic-rich shale in the A6 section; c leaf fossils in the marl in the C section; d argillaceous sediment and organic-rich shale in the H16 section; e geometrical relationship between tuffite and argillaceous sediment in the H16-2 section; f tuff/tuffite, argillaceous sediment, marl, and fault in H16-2 section
C section
The lowermost part of this section is composed of dark brown, 4 m-thick, organic-rich shale, and continues upward with 16 m-thick, argillaceous sediments and organic-rich shale levels (Fig. 3). The argillaceous sediments are exposed in two levels, generally showing a gray color, a plastic character, and are thin-bedded (20–30 cm). Dark brown 4 m-thick, organic-rich shale occurs between the two levels of argillaceous sediment. This section continues upward with volcano-sedimentary units (tuff, tuffite), mudstone, and argillaceous sediment levels intercalating sandstone. The tuff is observed in two levels. The tuffite is gray in color, bearing rounded to subrounded lithic fragments, and observed in two levels and both are 1–2 m thick. The mudstone shows a greenish-beige color and conchoidal fracture and is rich in organic material. The sandstone is gray in color, is thin-bedded and is 4 m thick. These units continue upward with gray marl (50 cm) and beige, thick-bedded tuff (2–3 m) with a total thickness of 30 m. The marl contains leaf fossils (Fig. 4c).
Keles-Harmanalan Region
H16 section
This section begins with a 12 m thick, gray, hard silica level intercalated with mudstone (1.5 m thick) and dark gray, plastic, muscovite-bearing argillaceous sediments (2 m thick), and beige organic-rich shale (Fig. 3). The silica level is overlain by a 10 m thick tuff. The section continues upward with an organic-rich shale that intercalated two coal seams 2 m thick, dark gray argillaceous sediment; and light brown organic-rich shale (2 m thick) (Fig. 4d). The uppermost part of this section consists of a 50 cm-thick sandstone and is covered by a 10 m thick, greenish-gray argillaceous sediment.
H16-2 section
The H16-2 section begins with gray, thin-bedded (10–15 cm) sandstone and continues upward with 50 cm-thick, brown argillaceous sediments and a 2 m-thick conglomerate (Fig. 3). Gray, friable, and mica-bearing tuffite overlies the conglomerate (Fig. 4e). The section continues upward with a 2 m-thick limestone and fluvial sediments consisting of cross-bedded conglomerate, sandstone, and tuffite and has a total thickness of 4 m. Fluvial sediments are overlain by beige, conchoidally fractured, thick-bedded argillaceous sediment (50 cm), 7 m-thick Fe-oxidized and altered tuff at the upper levels in addition, 20 m-thick, white, thin-bedded, (15–20 cm) limestone intercalates marl in the uppermost part (total thickness of 6.5 m) (Fig. 4f).
Materials and Methods
Sample Collection
To identify the lateral and vertical distribution of smectite in the Neogene Orhaneli-Gümüşpınar and Keles-Harmanalan coal mines, 70 samples were collected throughout the measured sections referred to as A6, C, H16, and H16-2 (Fig. 3).
Analytical Methods
The mineralogy was determined using optical microscopy, X-ray powder diffraction (XRD), and scanning electron microscopy – energy dispersive X-ray analysis (SEM–EDX). The rhyolitic tuff and andesitic tuffite, sandstone, organic-rich sandstone, and organic-rich shale samples were examined under a polarizing microscope (Nikon-LV 100Pol, Nikon Corporation, Tokyo, Japan). The powder XRD analysis of the parent rocks (tuff/tuffite, organic-rich shale, mudstone, sandstone), marl, and argillaceous sediments was carried out at the General Directorate of Mineral Research and Exploration of Turkey using a Rigaku D/Max–2200 instrument (Ultima PC, Tokyo, Japan). The XRD analyses were performed using CuKα radiation with a scanning speed of 1°2θ per min. Randomly oriented mounts of powdered whole-rock samples were scanned to determine the bulk mineralogy of each sample. Separation of the clay fraction was undertaken following the removal of free iron oxides and organic matter using the sodium dithionite-citrate procedure and hydrogen peroxide (30%) treatment, respectively (Kunze & Dixon, Reference Kunze, Dixon and Klute1986). Carbonate was removed using acetic acid with sodium acetate buffer of pH 5 (Jackson, Reference Jackson1956). The treated samples for clay mineralogical analysis (< 2 µm) were prepared by separating the clay fraction using sedimentation and centrifuging the suspension after an overnight dispersion in distilled water using a Hettich 32A centrifuge (Andreas Hettich GmbH and Co. KG, Tuttlingen, Germany). The clay particles were dispersed using ultrasonic vibration for ~ 15 min. Oriented specimens of the < 2 µm fractions were prepared from each sample using the following procedure: drying with air, solvating with ethylene glycol at 60°C for 2 h, and heated at 550°C for 2 h. Semi-quantitative abundances of rock-forming minerals were obtained using Brindley’s (Reference Brindley, Brindley and Brown1980) external standard method. The relative abundances of clay-mineral fractions were determined using their basal reflections and the mineral intensity factors of Moore and Reynolds (Reference Moore and Reynolds1989).
The SEM–EDX studies were performed at the Eskişehir Osmangazi University (Turkey) using a Hitachi-Regulus 8230 FE–SEM instrument (Hitachi High-Tech, Tokyo, Japan) and an ULTIM EXTREME detector (Oxford Instruments, Abingdon, UK). Clay-dominated bulk specimens were prepared for SEM–EDX analysis by adhering the fresh broken surface of each rock sample onto an aluminum sample holder with double-sided tape. The samples were then coated with a thin film (~ 3 nm) of gold/palladium using a Leica EM ACE600 (Leica Microsystems, Wetzlar, Germany).
A total of seven organic-rich shales and their intercalated coal seams were prepared according to standard palynological methods (Durand & Nicaise, Reference Durand, Nicaise and Durand1980; Tissot & Welte, Reference Tissot and Welte1984) at the Turkish Petroleum Corporation (TPAO) Research Center. Relative abundances of the palynomorphs have been calculated based on at least 200 counts for each sample. Abbreviations used for different relative abundances and the corresponding number of counts are as follows: Super Abundant (S): >100 specimens (50% or more), abundant (A): between 20 and 100 specimens (10–50%), common (C): between 6 and 20 specimens (3–10%), rare (R): between 2 and 5 specimens (1–3%), present (P): only 1 specimen (0.5%). Digital images of palynomorphs were captured using a Leica DC200 digital camera system.
Chemical analyses of 23 organic-rich shale, argillaceous sediments, mudstone, sandstone, marl, and their tuff/tuffite parent rock samples were performed at the Bureau Veritas Mineral Laboratories (Vancouver, Canada) using a PerkinElmer Elan 9000 instrument (PerkinElmer, Inc., Waltham, Massachusetts, USA) inductively coupled plasma–atomic emission spectroscopy (ICP–AES) for major and trace elements and inductively coupled plasma–mass spectrometry (ICP–MS) for rare-earth elements (REE) and a Spectro (Spectro Analytical Instruments Inc., Mahwah, New Jersey, USA) XLAB–2000 PEDX–ray fluorescence spectrometer (PEDXRF). The detection limits for the analyses were between 0.01 and 0.1 wt.% for major elements, 0.1 and 5 ppm for trace elements, and 0.01 to 0.5 ppm for REE. The Eu values are correct from ICP–MS laboratories because the Ba/Eu ratio <1000 in organic-rich shale, argillaceous sediments, mudstone, sandstone, marl, and tuff/tuffite samples (except H16-14 and C-14) (Loges et al., Reference Loges, Wagner, Barth, Bau, Göb and Markl2012).
The chemical index of weathering (CIW) of organic-rich shale, argillaceous sediments, mudstone, sandstone, marl, and tuff/tuffite whole-rock samples was calculated using the following equation (Harnois, Reference Harnois1988):

where CaO* = The CaO content incorporated in the silicate fraction.
Nine smectite and illite clay fractions were purified and analyzed for the H and O stable isotopes in the Cornell Isotope Laboratory at Cornell University, New York. Separation was undertaken after the removal of free iron oxides and organic matter using the sodium dithionite-citrate procedure and hydrogen peroxide (30%) treatments as described by Kunze and Dixon (Reference Kunze, Dixon and Klute1986). The isotope corrections were performed using a two-point normalization (regression) based on international standards (IAEA CO–1 and IAEA CO–8) for δ18O and CH–7 and benzoic acid for δD. The analyses were performed using a Thermo Delta V (Thermo Fisher Scientific, Waltham, MA, USA) isotope ratio mass spectrometer interfaced with a temperature-conversion elemental analyzer. The delta values for 2H and 18O were measured against the primary reference scale of Clayton and Mayeda (Reference Clayton and Mayeda1963). The data are reported in standard delta notation as per mil deviations from V-SMOW (Vienna Standard Mean Ocean Water). The standard deviation for internal standard benzoic acid for δ18O is 1.01% and for δD is 1.24%.
The δ34S was determined on five pyrite and chalcopyrite samples which were selected by handpicking under a binocular microscope. Stable-isotope analysis (δ34S) was conducted at the University of Arizona Department of Geosciences using an MAT 261–8 mass spectrometer [Thermo Fisher (Finnigan Corporation), Bremen, Germany]. The results of δ34S were referenced to V-CDT (Vienna Canyon Diablo Troilite).
δ34S was measured on SO2 gas in a continuous-flow gas-ratio mass spectrometer (ThermoQuest Finnigan Delta PlusXL, Tucson, Arizona, USA). The samples were combusted at 1030°C with O2 and V2O5 using an elemental analyzer (Costech, Tucson, Arizona, USA) coupled to the mass spectrometer. Standardization was based on international standards OGS–1 and NBS123 (Hosono et al., Reference Hosono, Lorphensriand, Onodera, Okawa, Nakano, Yamanaka, Tsujimura and Taniguchi2014), and several other sulfides and sulfate materials for sulfur that have been compared between laboratories. Calibration was linear in the range –10 to + 30%. Precision was estimated to be –0.15% or better (1 s) based on repeated internal standards.
Results
Petrography
The argillaceous volcano-sedimentary parent rocks of the coal mines were determined as rhyolitic tuff and andesitic tuffite, sandstone, organic-rich sandstone, and organic-rich shale. The rhyolitic tuff exhibits porphyritic and broken porcelain texture and is composed of partially altered and seriticized sanidine with Fe (oxyhydr)oxide character, a small amount of plagioclase (oligoclase), partially to completely argillized muscovite, and partially oxidized biotite and quartz in an argillized and carbonaceous groundmass (Fig. 5a). Muscovite and biotite crystals are bent. Quartz crystals have an irregular morphology and sharp edges. Volcanic glass and irregular pumice materials are abundant in rhyolitic tuff (Fig. 5a). Andesitic tuffite is composed of partially argillized plagioclase, a small amount of sanidine and pyroxene, partially-to-completely oxidized biotite, metamorphic, ophiolitic, and quartzite rock fragments, cemented by argillized and Fe (oxyhydr)oxidized groundmass (Fig. 5b–c). The amount of tuff/tuffite increases toward the Orhaneli-Gümüşpınar region.
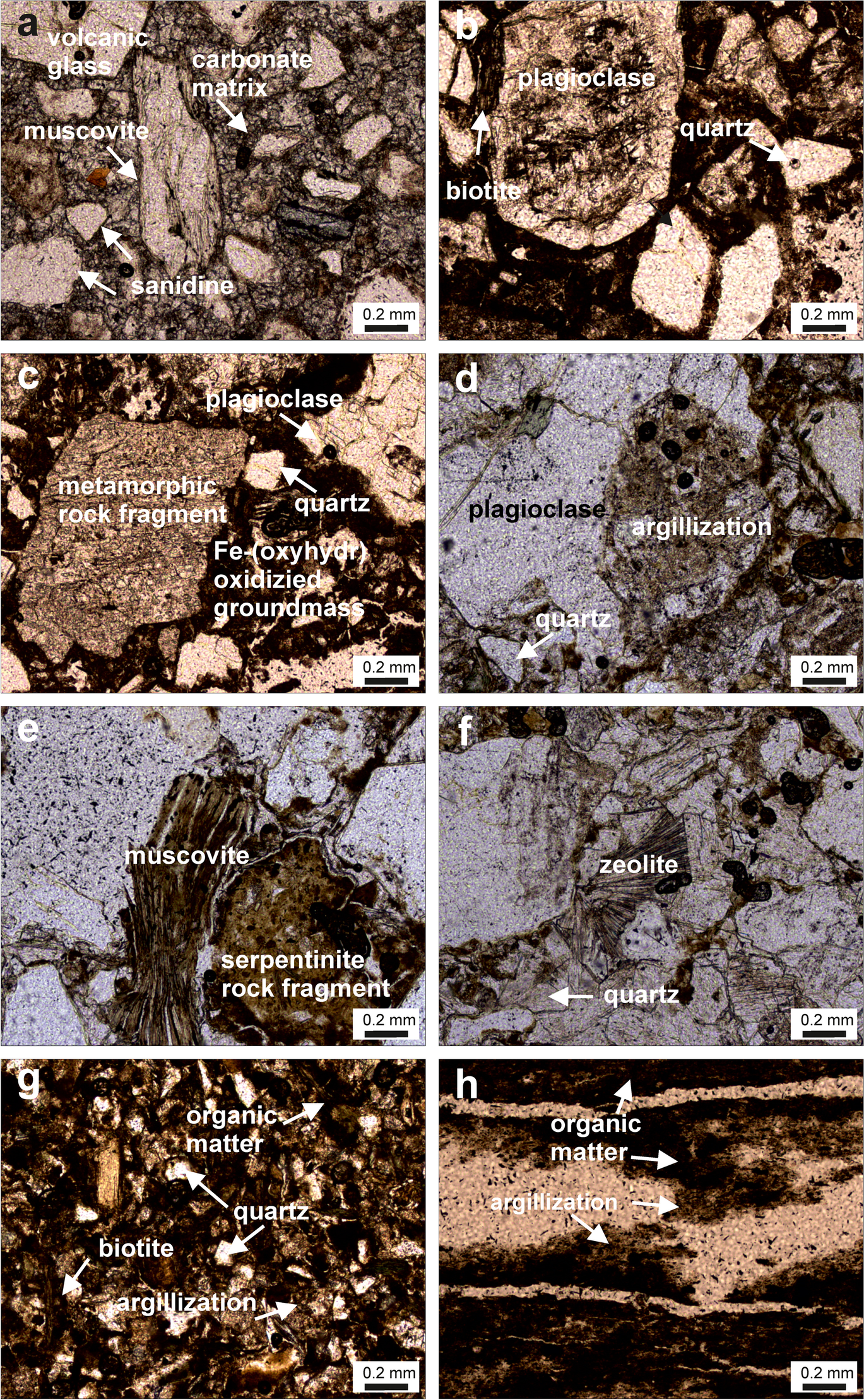
Fig. 5 Thin-section photomicrographs of the Orhaneli-Gümüşpınar and Keles-Harmanalan host rocks: a partially argillized and sericitizatied sanidine, argillized muscovite, and volcanic glass in argillized and carbonate groundmass of rhyolitic tuff (A6-25); b–c argillized plagioclase, quartz, pyroxene, biotite, and metamorphic rock fragment in Fe-(oxyhdr)oxidized groundmass of andesitic tuffite (C-20); d–f partially argillized bent muscovite, quartz, plagioclase, serpentinite rock fragments, and zeolite minerals in an argillized groundmass of sandstone (C-14); g chloritized bent biotite, anhedral quartz, and brownish-black organic matter in organic-rich sandstone (C-15); h argillized and seriticized brownish-black organic matter in thin layers parallel to laminate in organic-rich shale (A6-13)
Sandstone comprises monocrystalline and polycrystalline quartz, partially argillized bent muscovite, chloritized bent biotite, partially sericitized plagioclase, orthoclase, serpentinite rock fragments, and zeolite cemented by clay (Fig. 5d–f). Organic-rich sandstone was composed of abundant anhedral quartz, chloritized bent biotite, argillized feldspars, and brownish-black organic matter showing thin lamellae (Fig. 5g). Organic-rich shale consisted of argillized and sericitized brownish-black organic matter in a thin layer parallel to the lamination, cristobalite veins, and opaque minerals (Fig. 5h). The amount of fluvial sediments increased toward the Keles-Harmanalan region.
Mineralogical Composition
Orhaneli-Gümüşpınar region, A6 section
Smectite is a major constituent in clay-fraction and whole-rock samples, associated with small amounts of kaolinite and illite in the A6 section of the Orhaneli-Gümüşpınar region (Table 1 and Figs. 3 and 6). Abundant quartz, aragonite/calcite, feldspar, heulandite/clinoptilolite, and dolomite, are accompanied by accessory pyrite, siderite, and amphibole in organic-rich shale, argillaceous sediment, sandstone, tuff/tuffite, and mudstone. Smectite is found in tuff/tuffite, organic-rich shale, argillaceous sediment, sandstone, and mudstone, and in notable amounts in sandstone above and below the coal seams. Kaolinite occurs in organic-rich shale, and argillaceous sediments and illite is found in organic-rich shale, argillaceous sediments mudstone, and tuff.
Table 1 Mineralogical composition of samples from the Orhaneli-Gümüşpınar and Keles-Harmanalan coal mines. Sme: smectite, Ilt/Mca: illite/mica, Kln: kaolinite, Chl: chlorite, Hul/Cpt: heulandite/clinoptilolite, Py: pyrite, Fsp: feldspar, Qz: quartz, Amp: amphibole, Arg/Cal: aragonite/calcite, Dol: dolomite, Sid: siderite, acc: accessory, +: relative abundance of mineral (mineral name abbreviations after Whitney & Evans, Reference Whitney and Evans2010)
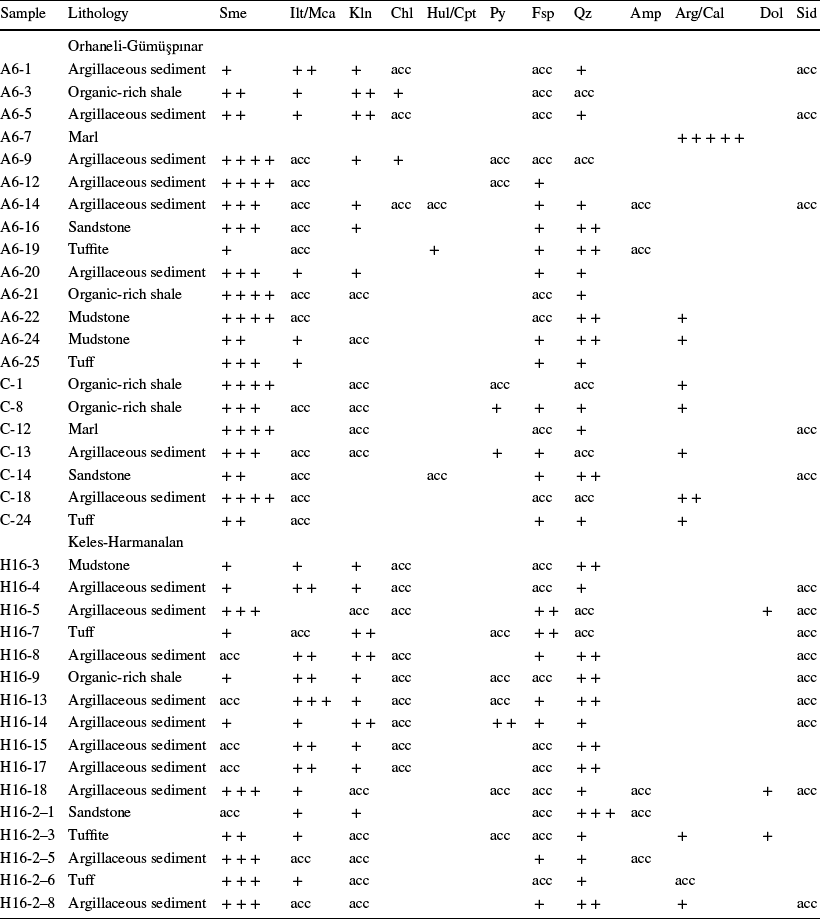
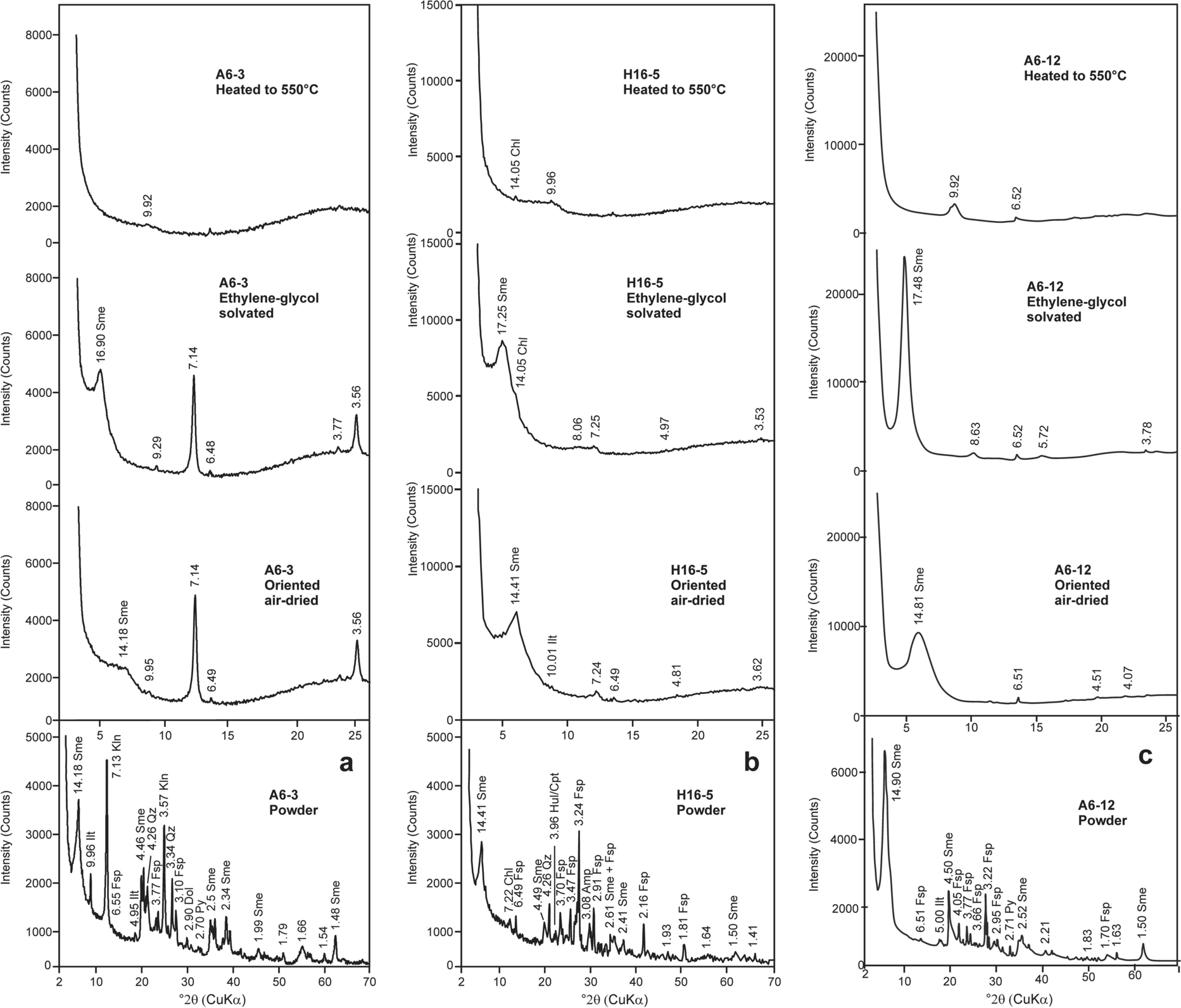
Fig. 6 XRD patterns of the bulk smectite: a – abundant, b – detrital, and c – authigenic sediment samples, oriented air-dried, ethylene glycol-solvated, and heated at 550ºC. Sme: smectite, Ilt: illite, Kln: kaolinite, Chl: chlorite, Hul/Cpt: heulandite/clinoptilolite, Qz: quartz, Fsp: feldspar, Amp: amphibole, Dol: dolomite, Py: pyrite
Orhaneli-Gümüşpınar region, C section
Smectite is the main clay mineral and it occurs in organic-rich shale, argillaceous sandstone, and tuff (Table 1 and Fig. 3). The intensity of smectite decreased toward the sandstone and tuff. The kaolinite and illite exist as accessory minerals and in smaller amounts than those in the A6 section. Feldspar, quartz, aragonite/calcite, pyrite, and siderite were detected in organic-rich shale, marl, and tuff.
Keles-Harmanalan region, H16 section
Abundant smectite and occasionally kaolinite and small amounts of illite were determined in the Keles-Harmanalan region (Table 1). The concentration of smectite in the H16 and H16-2 sections of the Keles-Harmanalan region was less than that observed in the Orhaneli-Gümüşpınar region (Fig. 3). Smectite, illite, and kaolinite were associated with quartz, feldspar, and accessory pyrite, siderite, and amphibole in mudstone, argillaceous sediments, tuff, and organic-rich shale (Fig. 6). Smectite was found predominantly in argillaceous sediments. Illite occurs in argillaceous sediments, and organic-rich shale, and its amount decreased in sandstone, and tuff/tuffite. Kaolinite was determined in tuff, argillaceous sediments, mudstone, and organic-rich shale.
Keles-Harmanalan region, H16-2 section
Smectite is the main clay mineral and occurs in tuff/tuffite and argillaceous sediments (Table 1). The amounts of kaolinite and illite decreased relative to the H16 section (Fig. 3). Aragonite/calcite and other non-clay minerals were detected in sandstone, tuff/tuffite and argillaceous sediments.
Smectite was identified by a broad peak at 14.18–14.90 Å which expanded to 16.90–17.48 Å following ethylene-glycol solvation; the peak collapsed to 9.92–9.96 Å following heating to 550°C (Fig. 6a–c). Kaolinite was determined by means of a narrow, sharp peak at 7.13 Å, and was not affected by ethylene–glycol solvation; the basal (001) peaks collapsed following heating to 550°C (Fig. 6a). Illite/mica was identified by peaks at 9.96 and 4.95–5.00 Å. Feldspar was determined by peaks at 3.10–3.24, 3.70, 3.77, 4.05, and 6.49–6.55 Å. Chlorite was determined by a peak at 14.05 Å following ethylene-glycol treatment and not affected by heating at 550°C (Fig. 6b). Heulandite/clinoptilolite and pyrite were determined by peaks at 3.96 and 2.70–2.71 Å, respectively.
The SEM studies were carried out on argillaceous sediments, organic-rich shale, mudstone, and sandstone intercalating coal seams in the Bursa-Orhaneli and Keles-Harmanalan coal mines. Smectite shows two types of morphologies: (1) platy and flaky smectite edging devitrified glass shards and highly resorbed feldspar – the latter shows bar-shaped, and subparallel comb structure in tuffaceous materials within the organic-rich shale (Fig. 7a–c); and (2) irregular flaky smectite is mostly coating the detrital volcanogenic materials or filling the pores in the fluvial sedimentary units such as mudstone and sandstone (Fig. 7d–h). Smectite crystals also edge fibrous crystals resembling illite formed via a dissolution–precipitation mechanism (Fig. 7e, f). Smectitic materials also enclose sphere-shaped opal-CT (Fig. 7g). The pores between the smectite crystals are filled locally by framboidal pyrite (Fig. 7h), aragonite crystals mostly showing radial morphology (Fig. 7i), and siderite crystals (Fig. 7j) and are associated with book-like, subparallel oriented, and anhedral kaolinite (Fig. 7k). Blocky euhedral heulandite/clinoptilolite crystals are associated with the small amount of smectite (Fig. 7l).
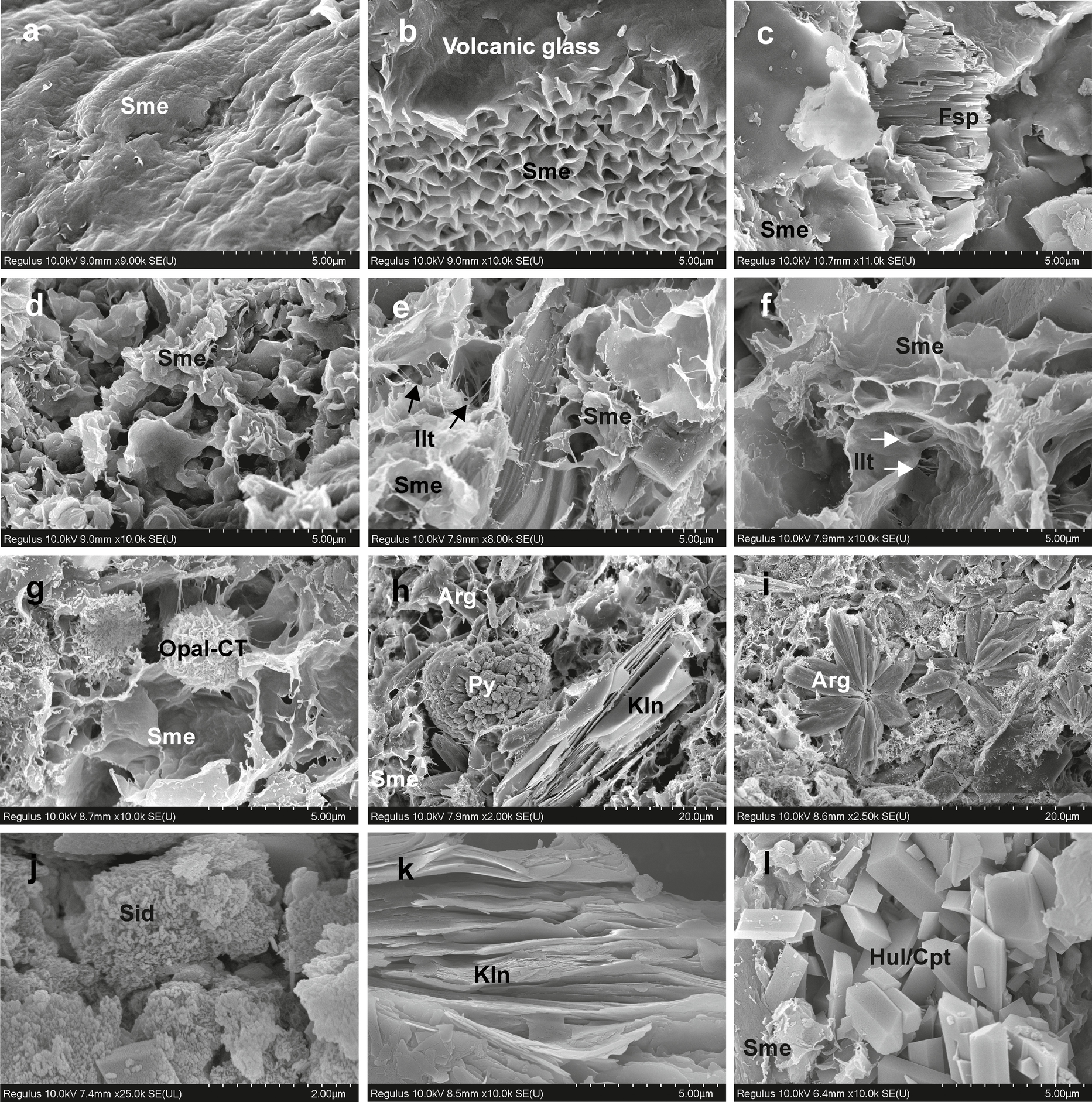
Fig. 7 SEM images of: a oriented platy smectite (A6-3); b smectite flakes edging devitrified volcanic glass (A6-3); c smectite flakes edging altered feldspar (A6-9); d–f flaky smectite locally edging illite coating and detrital materials (H16-5; A6-3; C-8); g spherical opal-CT between smectite flakes (C-8); h framboidal pyrite filling voids among smectite associated with platy kaolinite and aragonite (H6-14); i radial aragonite crystal in the void of smectite flakes (C-8); j siderite crystals in voids of smectite (H16-14); k close view of book-like kaolinite (A16-4); l blocky, euhedral heulandite/clinoptilolite crystals (C-14)
Palynology
The dominant terrestrial wood-type organic matter was determined in all of the samples except C-1; light-colored amorphous type organic matter and light-colored terrestrial (herbaceous) organic matter were identified (Fig. 8a).
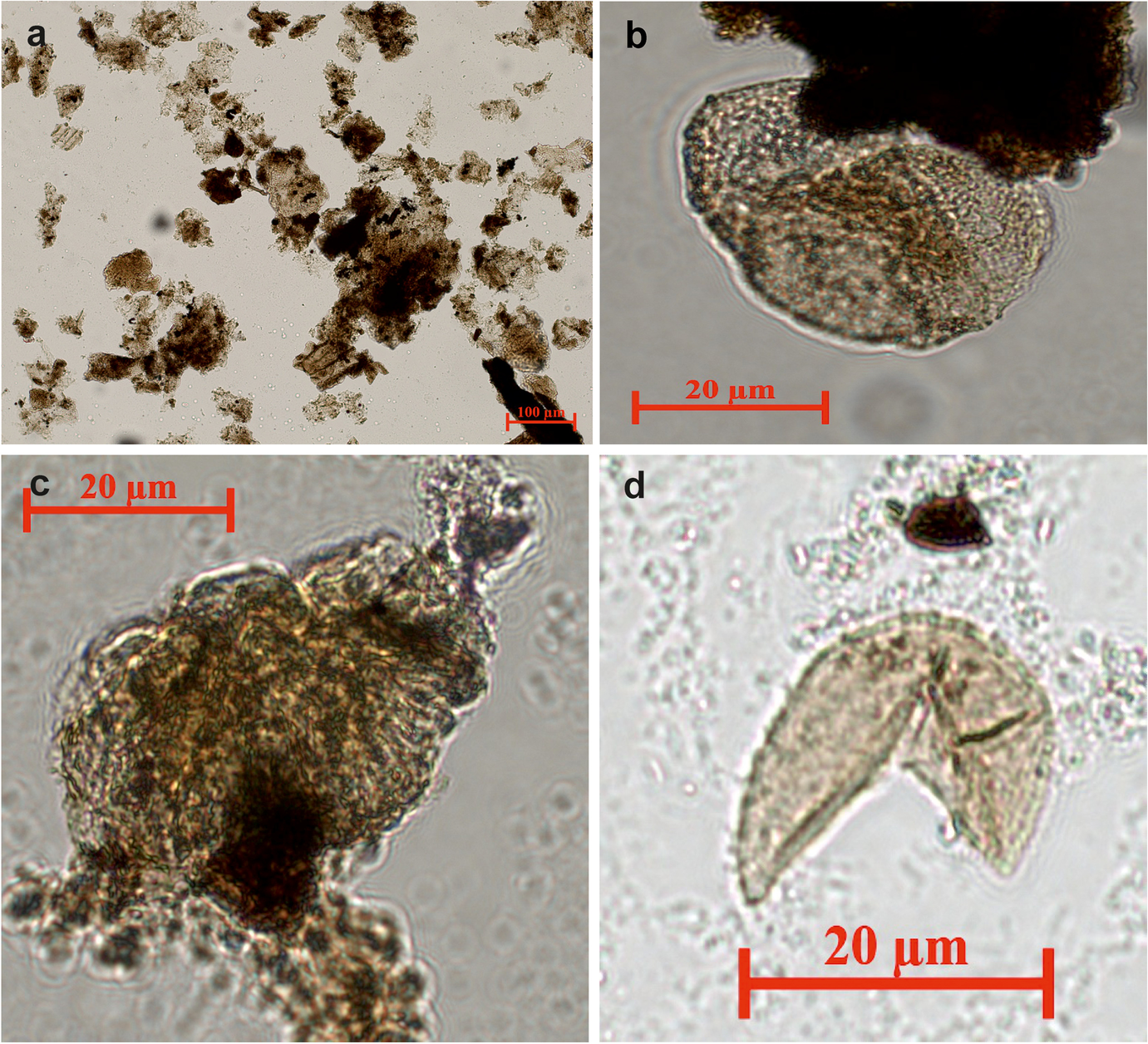
Fig. 8 Organic matter and pollen types from Orhaneli-Gümüşpınar and Keles-Harmanalan coal mines are: a terrestrial organic matter (C-1); b Bisaccat pollen (A6-7); c Botryococcus braunii (C-1); d Intratripollenites instructus (A6-3)
Palynological analysis showed that the samples are poor in organic palynomorph content. The common palynomorphs are Bisaccat pollen (6–20%) in sample A6-7 (Fig. 8b). Botryococcus braunii is determined at a weight percentage of 6–20% in sample C-1 (Fig. 8c). Rare (2–5%) Inaperturopollenites hiatus was found in sample A6-3 (Fig. 8d); Laevigatosporites sp., Leiotriletes adriennis, Subtriporopollenites simplex and Intratripollenites instructus were found in the A6, C, and H16 sections.
Chemical analyses
Major element oxides
The chemical analyses of representative organic-rich shale, argillaceous sediment, mudstone, sandstone, marl, and tuff/tuffite samples collected from the study area are presented in Table 2. The compositions of argillaceous sediments in the Orhaneli-Gümüşpınar and Keles-Harmanalan areas are characterized by small SiO2 (ave. 53.53 and 49.01 wt.%) and large Al2O3 (ave. 19.07 and 16.62 wt.%) values, respectively. Tuff sample (H16-7) is composed of SiO2 (51.70 wt.%) and significant amounts of Al2O3 (22.37 wt.%), Fe2O3 (4.97 wt.%), and MgO (1.20 wt.%). The Al2O3/TiO2 ratios range between 26.1 and 143.3 in Orhaneli-Gümüşpınar and between 23.7 and 69.8 in Keles-Harmanalan.
Table 2 Major- (wt.%) and trace-element (ppm) compositions of fresh and altered rocks from the study area (see Table 1 for the mineralogical compositions of the samples)

ƩREE = the sum of (La–Lu) + Y; ƩLREE = the sum of La–Nd; ƩMREE = the sum of (Sm–Ho); ƩHREE = the sum of (Er–Lu);
Eu/Eu* = EuN/ [(SmNx0.67) + (TbNx0.33)] (Bau & Dulski, Reference Bau and Dulski1996) and Ce/Ce* = 0.5LaN + 0.5PrN. (Dai et al., Reference Dai, Graham and Ward2016) LOI: loss on ignition at 1050°C
In the TiO2/Ni discrimination diagram (Fig. 9; Floyd et al., Reference Floyd, Winchester and Park1989) most of the argillaceous sediment samples fall mainly in the acidic magmatic rock portion, except for a few samples that fall into the sedimentary rock field.
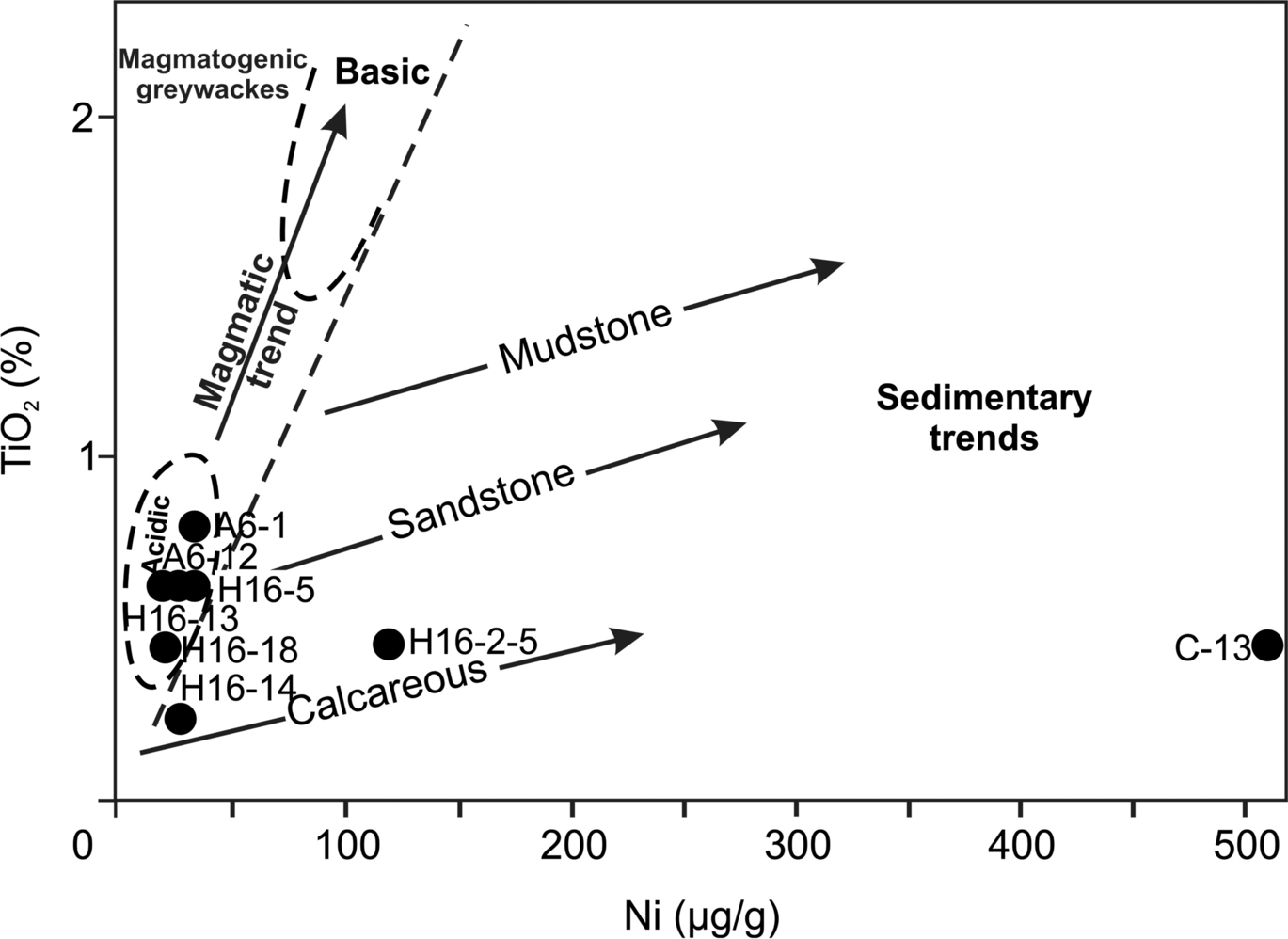
Fig. 9 Provenance diagram of Ni vs. TiO2 in the argillaceous sediment samples studied (after Floyd et al., Reference Floyd, Winchester and Park1989)
The plot of argillaceous sediments on the SiO2 vs. Al2O3 + K2O + Na2O diagram shows that clay minerals formed under arid and semi-arid conditions (Fig. 10; Suttner & Dutta, Reference Suttner and Dutta1986). The Al2O3 vs. TiO2 plot (r2 = 0.61) (Fig. 11a) exhibits a positive correlation and Al2O3 vs. CaO (r2 = 0.67) shows a negative correlation in the tuff/tuffite, marl, organic-rich shale, sandstone mudstone, and argillaceous sediment samples (Fig. 11b).
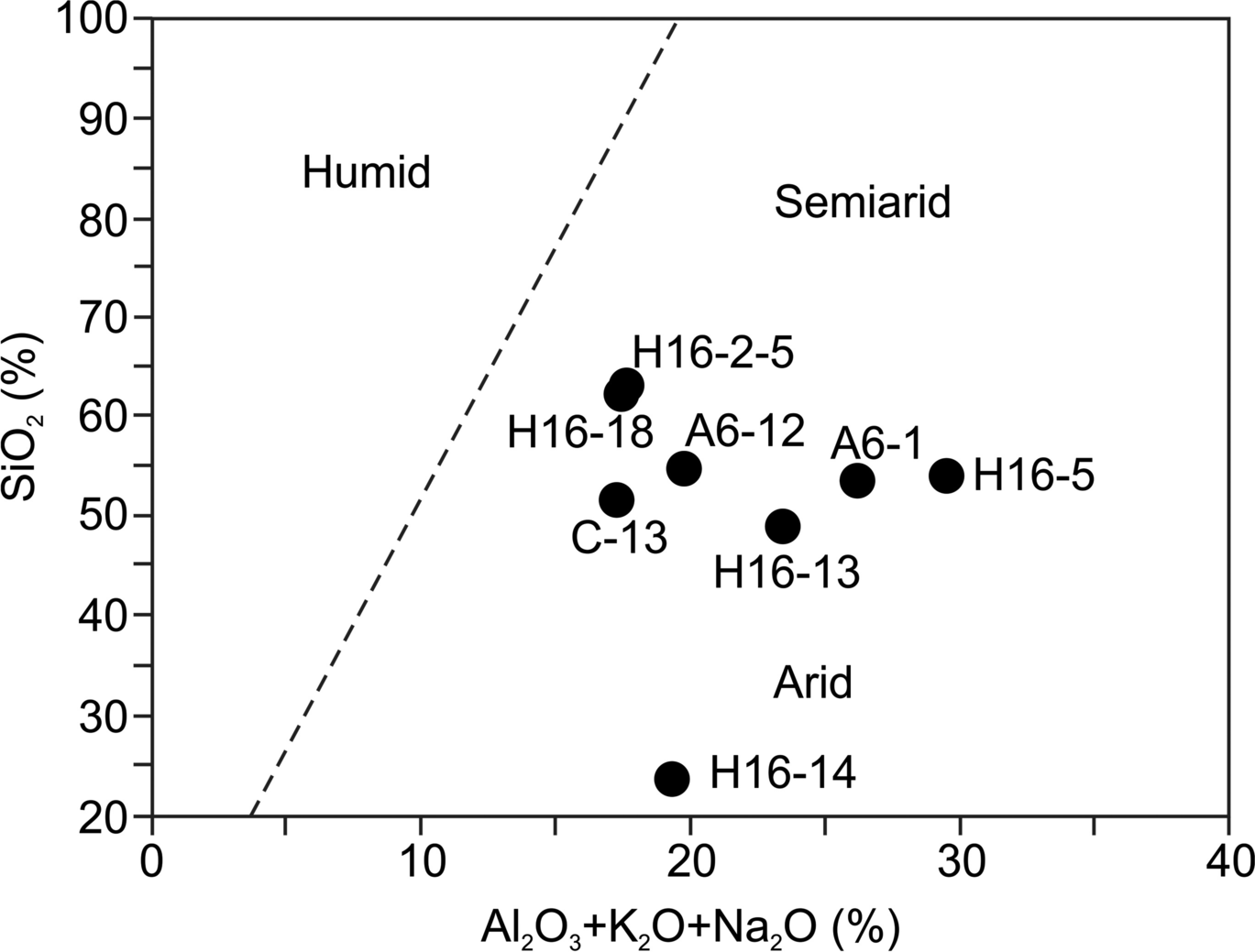
Fig. 10 Paleoclimate discrimination diagram of SiO2 vs. (Al2O3 + K2O + Na2O) in the argillaceous sediment samples studied (after Suttner & Dutta, Reference Suttner and Dutta1986)
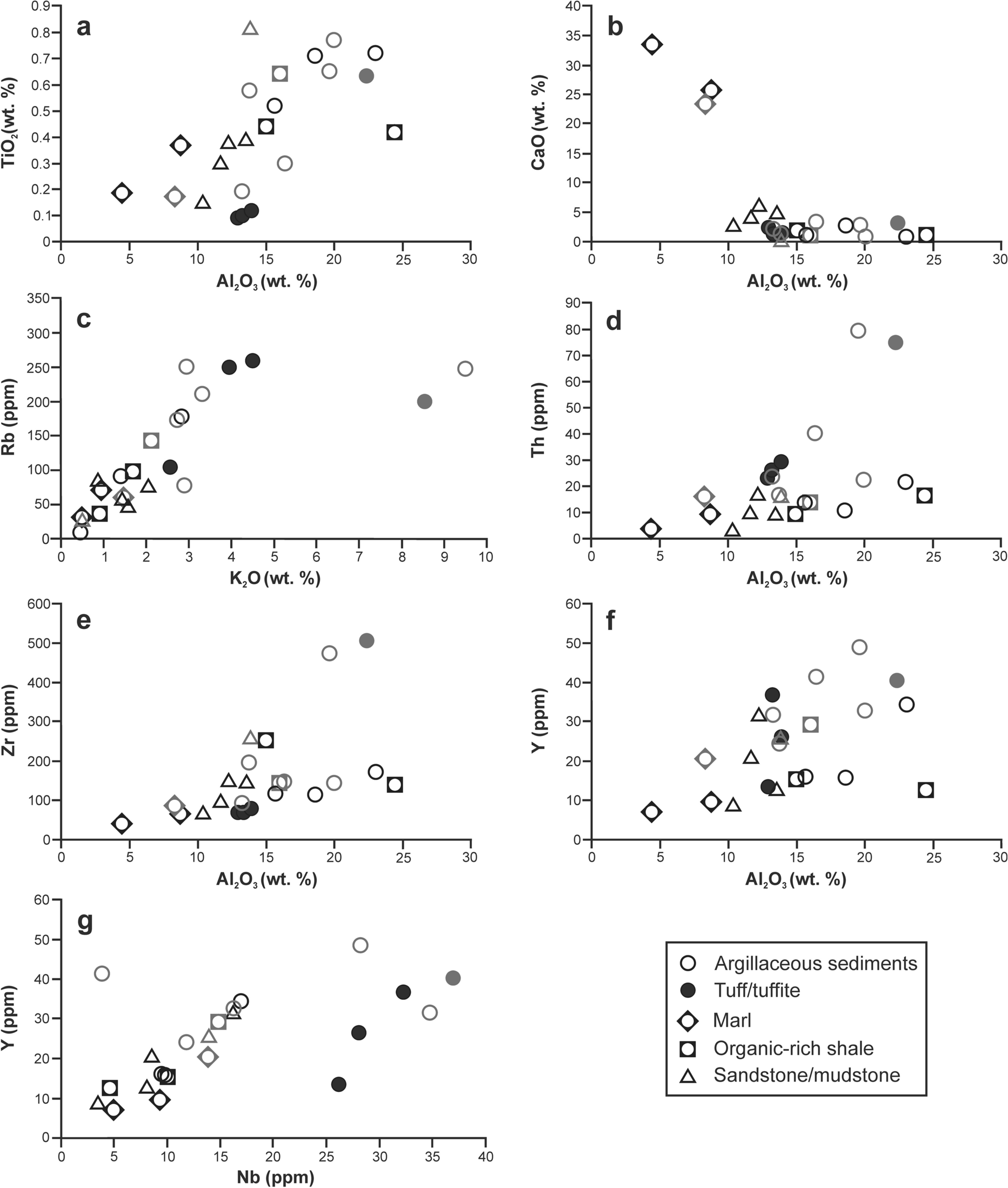
Fig. 11 Elemental variation diagrams for major oxides (wt.%) and trace elements (ppm) of the Orhaneli-Gümüşpınar and Keles-Harmanalan argillaceous sediment, tuff/tuffite, marl, organic-rich shale, and sandstone/mudstone samples: a Al2O3 vs. TiO2; b Al2O3 vs. CaO; c K2O vs. Rb; d Al2O3 vs. Th; e Al2O3 vs. Zr; f Al2O3 vs. Y; g Nb vs. Y. (The black and gray symbols represent samples from the Gümüşpınar and Keles areas, respectively)
The large iron oxide content (up to 8.69 and 18.86 wt.%) and the total S content (TOT/S) (up to 0.96 and 13.78 wt.%) are determined in argillaceous sediments of Orhaneli-Gümüşpınar and Keles-Harmanalan areas, respectively. The total carbon (TOT/C) values show max. 9.73 and 5.47 wt.% in marls, and max. 12.08 and 23.42 wt.% of organic-rich shale in the Orhaneli-Gümüşpınar and Keles-Harmanalan areas, respectively.
The chemical index of weathering (CIW) values of the parent rocks increase toward the argillaceous sediments (up to 92.59 except for organic-rich shale and 91.48 except mudstone) of the Orhaneli-Gümüşpınar and Keles-Harmanalan areas, respectively (Table 2).
Trace elements
Ba (up to 662 and 3406 ppm), Sr (up to 304.7 and 8223.5 ppm), Zr (up to 171.8 and 472.8 ppm), Co (up to 60.5 and 21.6 ppm), Cr (up to 513.2 and 1197.4 ppm), Ni (up to 548.2 and 117.9 ppm), Cu (up to 43.4 and 46.3 ppm), and Zn (up to 105 and 92 ppm) values in argillaceous sediments of the Orhaneli-Gümüşpınar and Keles-Harmanalan area, respectively, exhibit large values compared to those of the volcanogenic and terrigenous sediments (Table 2).
The ratios of Ni/Co (0.9–15.8), V/(V + Ni) (0.1–0.8), V/Cr (0.03–8.1), and Cu/Zn (0.1–0.9) were determined (Table 2). In terms of element correlations, K2O correlated positively with Rb (r2 = 0.74) (Fig. 11c). The plot of Al2O3 vs. Th (r2 = 0.50), Al2O3 vs. Zr (r2 = 0.55), Al2O3 vs. Y (r2 = 0.48), and Nb vs. Y (r2 = 0.60) also showed positive correlations (Fig. 11d–g).
Rare earth elements (REE)
Whole-rock REE abundances for the marl, parent rocks (tuff/tuffite, organic-rich shale, sandstone, mudstone), and argillaceous sediments were normalized to Upper Continental Crust (UCC) values (Fig. 12; Taylor & McLennan, Reference Taylor and McLennan1985). The average range of ΣREE values of the parent rocks and argillaceous sediments are 73.7–974.2 and 186.6–369.1 ppm, respectively (Fig. 13). The average ΣLREE and ΣHREE values of the parent rocks and argillaceous sediments are 56.71–869.4 and 148.51–300.72 ppm, 4.44–6.14 and 4.98–6.39 ppm, respectively (Table 2). The average light rare earth elements (LREE) exhibit slight enrichment relative to heavy rare earth elements (HREE) concentrations of the parent rocks and argillaceous sediments. The Eu anomaly (0.94–2.34 and 1.04–2.93) showed positive values in the Orhaneli-Gümüşpınar and Keles-Harmanalan areas, respectively (Table 2). The Ce value showed a negative anomaly (0.28–0.95 except for samples A6-16, A6-22, and A6-1) in Orhaneli-Gümüşpınar and positive anomalies (1.09–6.95 except samples H16-2–4, H16-9, and H16-2–5) in the Keles-Harmanalan area (Table 2). Rb and Nb show negative anomalies.
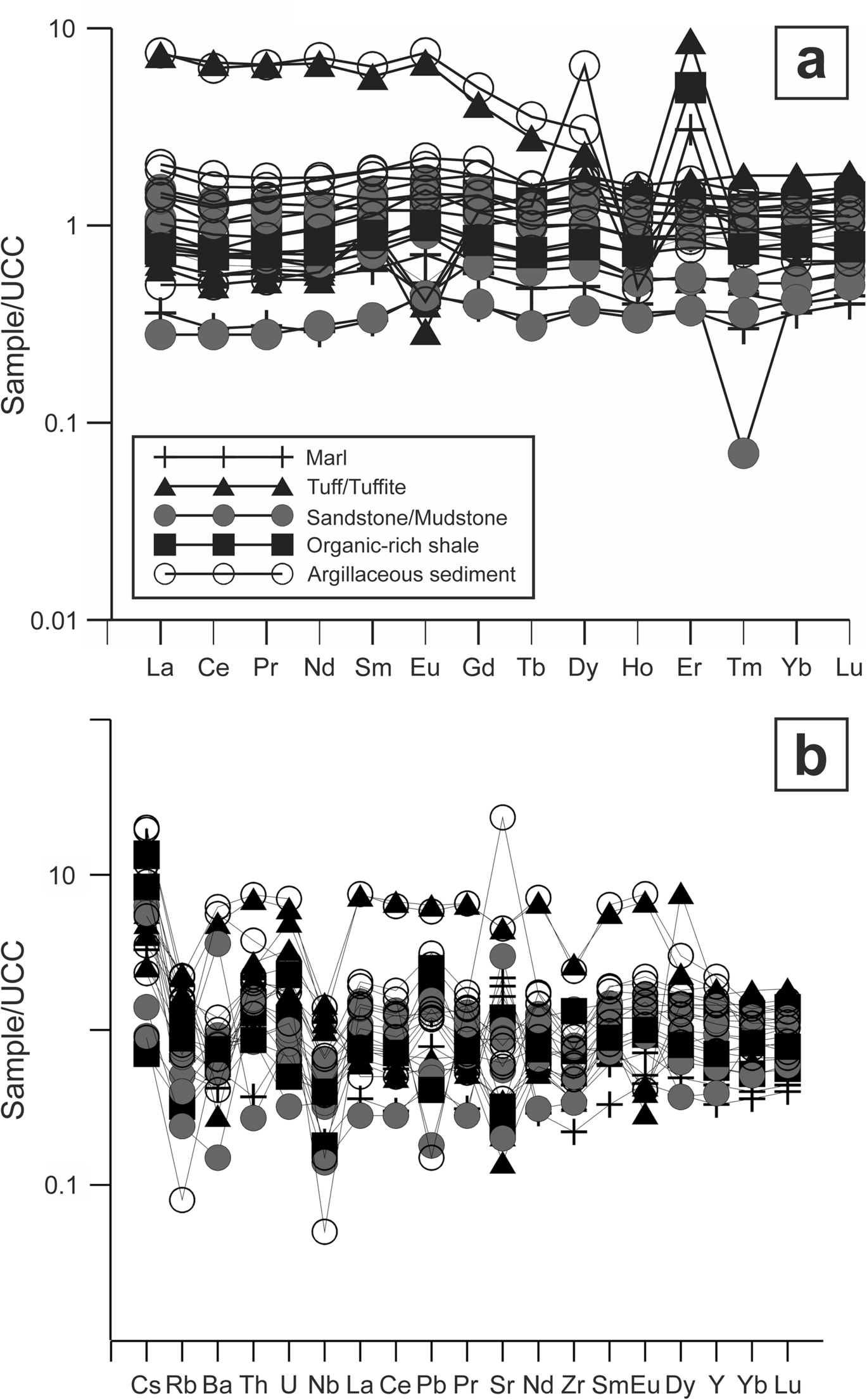
Fig. 12 UCC-normalized a REE and b trace element patterns of tuff/tuffite, marl, organic-rich shale, sandstone/mudstone, and argillaceous sediment samples from the Orhaneli-Gümüşpınar and Keles-Harmanalan region (Taylor & McLennan, Reference Taylor and McLennan1985)
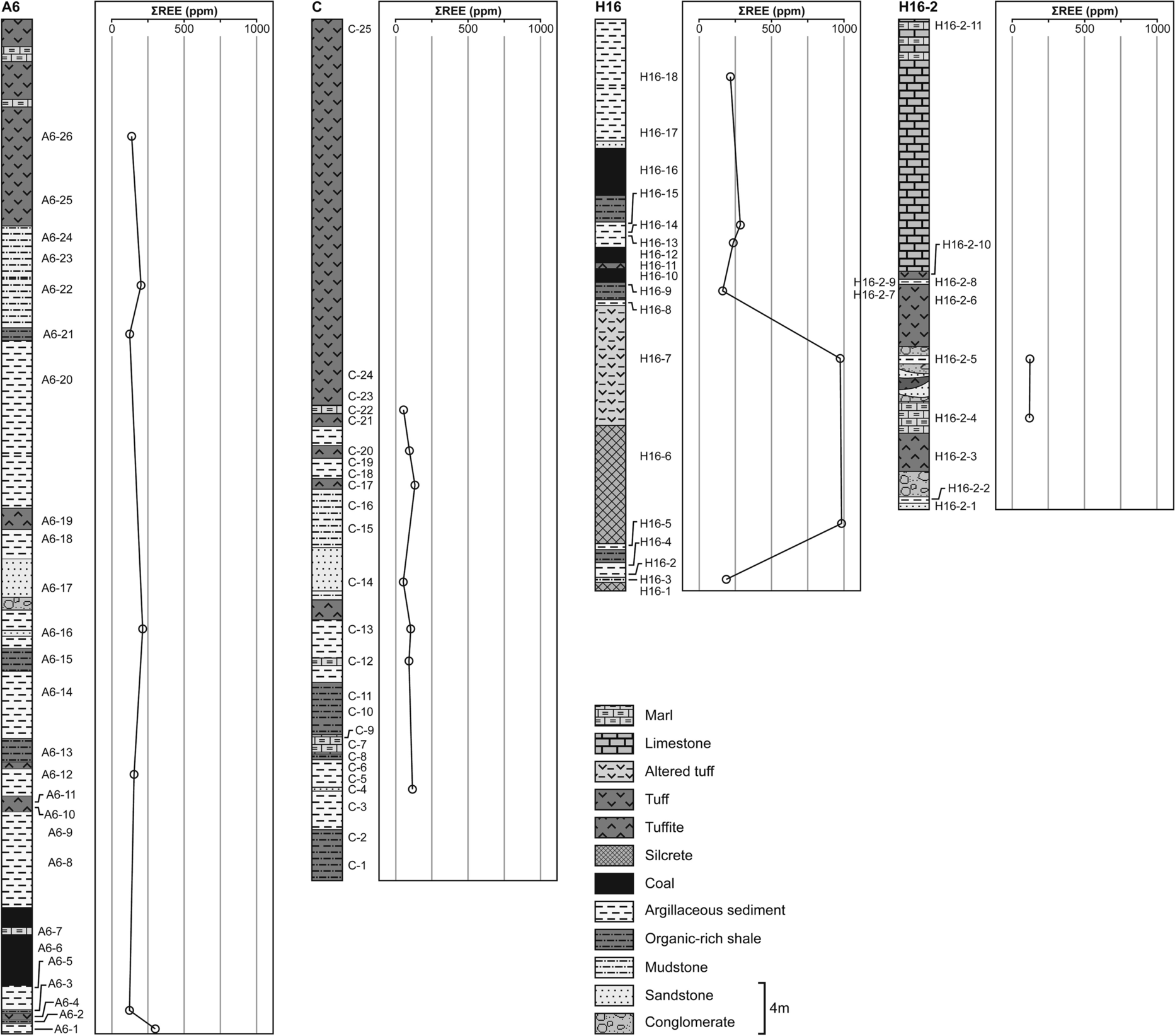
Fig. 13 Variation in ΣREE values in the graphical log of argillaceous sediments and their parent rocks
Smectite chemistry
The structural formula of montmorillonitic smectite was calculated from the chemical analysis of the clay fractions (Table 3) as follows:
Table 3 Chemical composition (wt.%) and structural formula of a purified smectite sample
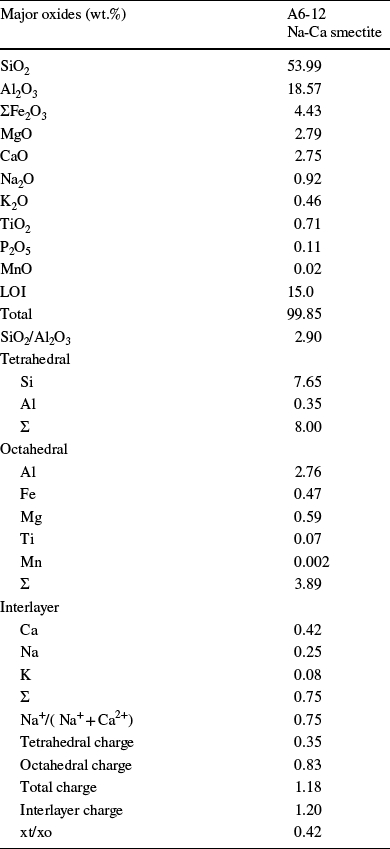

This formula was constructed by filling the tetrahedral sites with all of the Si and Al, and the octahedral cations included the remaining Al and Fe(III), Mg, Ti, and Mn2+. The SiO2/Al2O3 ratio is 2.90 compatible with montmorillonite (Christidis & Dunham, Reference Christidis and Dunham1997).
The tetrahedral/octahedral charge ratio (x t/x o) of smectites was 0.42, consistent with montmorillonite (Güven, Reference Güven and Bailey1988). The Na+/(Na+ + Ca2+) ratios of the smectite, 0.75, was determined as Na-Ca-smectites (Table 3; Takagi et al., Reference Takagi, Koh, Song, Itoh and Mogi2005).
Stable isotope geochemistry
The O- and H-isotopic compositions of the puri0fied smectite and illite samples from the study area range from 1.39 to 9.13‰ and –64.24 to –87.61‰, respectively (Table 4 and Fig. 14). The samples plot to the left side of the supergene–hypogene line and close to the magmatic water box. The correlation of δ18O with Fe2O3 in smectite shows a negative trend (Table 4).
Table 4 Oxygen-hydrogen-isotopic compositions of smectite and illite from the study area
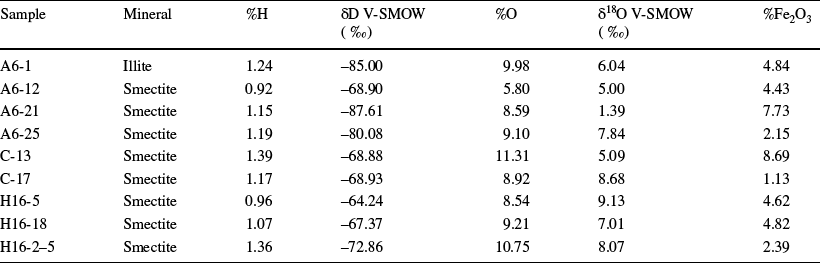
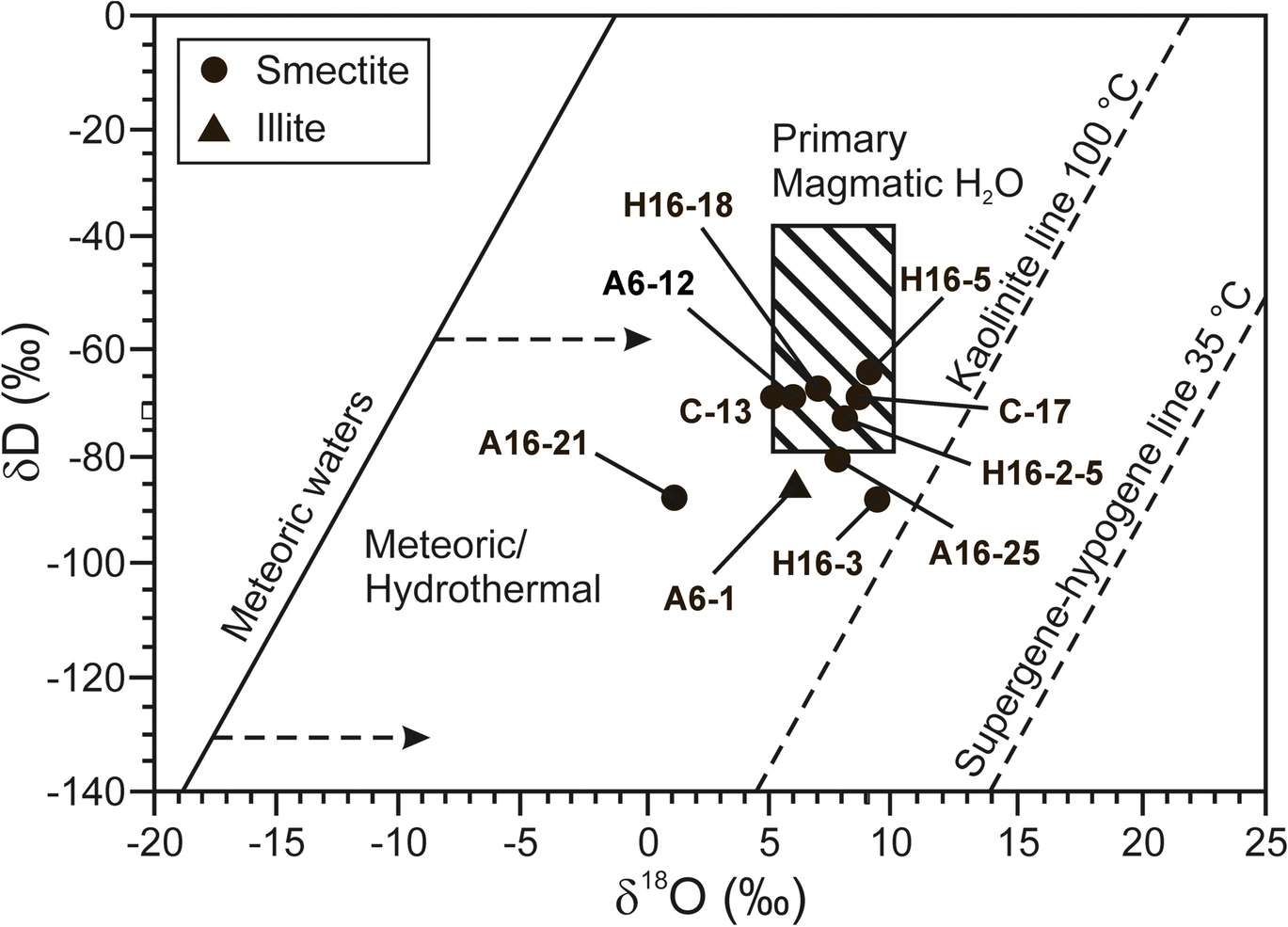
Fig. 14 δD vs. δ18O plot showing isotopic compositions of smectite and illite from the Orhaneli-Gümüşpınar and Keles-Harmanalan region (Sheppard, Reference Sheppard, Valley, Taylor and O'Neil1986). The supergene/hypogene kaolinite equilibrium line with meteoric water at 35°C is from Sheppard et al. (Reference Sheppard, Nielsen and Taylor1969). The kaolinite line in equilibrium with meteoric water at a temperature of 100°C is from Hayba et al. (Reference Hayba, Bethke, Heald and Faley1985). The meteoric water line is from Craig (Reference Craig1961)
The wide range of positive and negative δ34S of pyrite and chalcopyrite samples from Bursa-Orhaneli-Keles range from –19.2 to 15 and from –16.4 to –1.5‰, respectively (Table 5). Pyrite-chalcopyrite pairs yield isotope equilibrium temperatures of 104.2–152.1°C using the equations of Ohmoto and Rye (Reference Ohmoto, Rye and Barnes1979).
Table 5 δ34S composition of pyrite, and chalcopyrite samples from the study area
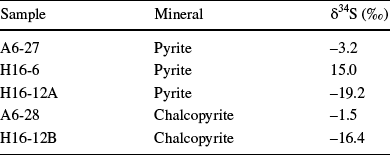

Discussion
Depositional Environment of Clays
The argillaceous sediments in the Bursa-Orhaneli and Keles-Harmanalan coal mines were developed in a swampy and lacustrine environment in an E–W-trending graben system (west Anatolia) controlled by an extensional tectonic regime associated with volcanism and geothermal activities (Şengüler, Reference Şengüler2004). The alteration of Triassic–Eocene metamorphic, ophiolitic, and igneous rocks (granitoid) of the basement by tectonic activity, alternation of warm and subtropical temperature, and rainfall regime favored the formation of clay-bearing detrital materials similar to those reported by Sáez et al. (Reference Sáez, Inglès, Cabrera and de las Heras2003).
In the first stage, the increase in the amount of terrigenous fluvial input toward the Keles-Harmanalan basin favored the deposition of detrital smectite in the organic-rich shale, mudstone, and sandstone (Figs. 3 and 15), and continued with an input of volcanic material upward in the sequence (Fig. 15). Consequently, in the second stage, the volcanic material was subjected to extensive alteration under diagenetic conditions (Fig. 15). Thus, smectite shows a detrital origin from terrigenous material and an authigenic origin in claystone which originated from the volcanic units.

Fig. 15 Simplified genetic model of the argillaceous fluvial-lacustrine sediments and volcanogenic material-intercalated coal seams in the Orhaneli-Gümüşpınar and Keles-Harmanalan basins
Paleoclimate and paleoenvironment
The enrichment of Bisaccat pollen in the Upper Coal Seam is indicative of a dry, warm to subtropical climate in a continental area (Wheeler & Götz, Reference Wheeler and Götz2017). The algal forms of Botryococcus braunii formed in fresh water and lakes in the study area (Sezgül Kayseri & Akgün, Reference Sezgül Kayseri and Akgün2008).
The presence of sporomorphs such as Intratripollenites instructus, Inaperturopollenites hiatus, and Subtriporopollenites simplex in the organic-rich shale suggested that the sediments were deposited during the Late Eocene to Middle Miocene period (Benda et al., Reference Benda, Innocenti, Mazzuoli, Radicati and Steffens1974; Nakoman, Reference Nakoman1968; Roche & Schuler, Reference Roche and Schuler1980). The plot of SiO2 vs. (Al2O3 + K2O + Na2O) on the paleoclimate discrimination diagram suggests arid and semi-arid paleoclimate conditions in the study area, similar to those reported for the Huainan Coal basin, China (Chen et al., Reference Chen, Liu, Wu and Sun2016).
Mineralogical proof from argillaceous sediment and parent-rock samples
The sharp-edged, irregularly-shaped, poorly-sorted quartz in thin sections from the study area suggest a pyroclastic origin, with monomineralic, well sorted, subrounded quartz having a terrigenous origin that was affected partially by the silica-rich hydrothermal fluid that favored the increase of quartz in sandstone, tuffite, and argillaceous sediments similar to the coal and tonsteins of Yunnan-China (Zhao et al., Reference Zhao, Dai, Graham and Wang2016). Furthermore, the metamorphic, ophiolitic, and quartzite rock fragments were the main source of detrital input. In addition, fracture-filling pyrite and chalcopyrite suggest the contribution of hydrothermal fluids. The presence of pyrite and chalcopyrite in coal seams, organic-rich shale, and marl exhibit the influence of sulfate-rich groundwater and/or hydrothermal fluids in a reducing environment based on S-isotope analysis similar to those reported by Jiang et al. (Reference Jiang, Qian and Zhou2016).
Examinations of the mineralogy and micromorphology showed that the relative increase in smectite and associated quartz, amphibole, and accessory chlorite, and the decrease in feldspar in argillaceous sediments, organic-rich shale, mudstone, and sandstone, compared to those in the volcanic units, and the significant degradation of quartz in detrital sediments suggest that smectite had a detrital origin in these units (Table 1 and Figs. 5 and 6a, b). In contrast, the close association of smectite with feldspar, the volcanic glass in a tuffaceous unit of the Bursa-Orhaneli and Keles-Harmanalan basins and the absence or rare presence of small amounts of detrital sediments such as quartz, amphibole, and chlorite suggest that smectite was formed authigenically (Table 1 and Figs. 5, 6c, and 7; Aldega et al., Reference Aldega, Cuadros, Laurora and Rossi2009; Leggo et al., Reference Leggo, Cocheme, Demant and Lee2001). The association of kaolinite with feldspar, biotite, and muscovite suggests that degradation of these precursor minerals resulted in the leaching of Na, Ca, and K through flushing by groundwater or hydrothermal fluid of organic-rich shale, mudstone, sandstone, and tuff/tuffite under an open acidic hydrologic system all of which favored the formation of kaolinite (Ece et al., Reference Ece, Ekinci, Schroeder, Crowe and Esenli2013). The SEM analyses showed that illite edging smectite in the organic-rich shale, sandstone, and tuff/tuffite suggested that the degradation of smectite favored the formation of illite via dissolution and precipitation under alkaline conditions during early diagenesis (Çiflikli et al., Reference Çifikli, Çiftçi and Bayhan2013; Hower et al., Reference Hower, Eslinger, Hower and Perry1976).
The presence of blocky, euhedral heulandite/clinoptilolite crystals in argillaceous sediments, sandstone, and tuff/tuffite suggest the contribution of volcanogenic materials and formation at pH < 11 conditions similar to those reported by Esenli et al. (Reference Esenli, Kadir and Ekinci Şans2019).
The presence of siderite in sandstone, mudstone, organic-rich shale, and argillaceous sediments indicated the reaction of carbon dioxide with iron-bearing minerals (biotite, pyroxene) under a reducing environment (Reinink-Smith, Reference Reinink-Smith1990).
Geochemical Evidence from Major Oxides and Trace Elements of the Argillaceous Sediment and Host-rock Samples
Argillaceous sediment and host-rock (rhyolitic tuff/andesitic tuffite) samples have average Al2O3/TiO2 values 29.34–33.24 and 133.5–35.50, respectively, indicating that the volcanic parent rocks had an acidic character in Orhaneli-Gümüşpınar and intermediate to acidic in the Keles-Harmanalan basin with a less continental fluvial source similar to those reported by Dai et al. (Reference Dai, Graham and Ward2016) and Shangguan et al. (Reference Shangguan, Zhuang, Li, Li, Querol, Liu, Moreno, Yuan, Yang and Pan2020). The TiO2/Ni diagram also suggests acidic volcanic rocks and a few sedimentary rocks as sources (Floyd et al., Reference Floyd, Winchester and Park1989; Noori et al., Reference Noori, Ghadimvand, Movahed and Yousefpour2016). The average CIW values for the argillaceous sediments in Orhaneli-Gümüşpınar (84.30) and Keles-Harmanalan (78.45) basins show a similar intensity of chemical weathering.
The authigenic smectite showed enrichment of Al2O3, Fe2O3, and MgO, and partial depletion of SiO2, Na2O, K2O, and CaO relative to the rhyolitic to andesitic tuff/tuffite (Table 2; A6-25, C-17, C-20, and H16-7) in the Orhaneli-Gümüşpınar and Keles-Harmanalan basins similar to those reported for smectites from Manisa-Osmancalı, Turkey and Milos Island, Greece (Christidis & Dunham, Reference Christidis and Dunham1993; Çiflikli et al., Reference Çifikli, Çiftçi and Bayhan2013). The positive correlation of K2O vs. Rb suggests the replacement of K by Rb in feldspars, biotite, and illite during alteration of tuff/tuffite, argillaceous sediment, sandstone/mudstone, organic-rich shale, and marl (Motoki et al., Reference Motoki, Sichel, Vargas, Melo and Motoki2015). The plots of Al2O3 vs. Zr and Th show similar immobile behavior during the alteration and indicate the presence of accessory zircon in the coal (Karayiğit et al., Reference Karayiğit, Littke, Querol, Jones, Oskay and Christanis2017). The positive correlation between Al2O3 and TiO2 may be related to the abundant detrital material such as sandstone, mudstone, organic-rich shale, and argillaceous sediment inputs similar to those reported by Akinyemi et al. (Reference Akinyemi, Adebayo, Ojo, Fadipe and Gitari2013). The positive correlation between Nb and Y shows that the leaching of volcanic ash by groundwater was similar to that reported by Crowley et al. (Reference Crowley, Stanton and Ryer1989).
The ratios of V/(V + Ni), Ni/Co, V/Cr, and Cu/Zn in both Orhaneli-Gümüşpınar and Keles-Harmanalan basins are indicative of paleoredox conditions (Table 2; Akinyemi et al., Reference Akinyemi, Adebayo, Ojo, Fadipe and Gitari2013; Hatch & Leventhal, Reference Hatch and Leventhal1992). These results are also consistent with the reported V/(V + Ni) ratios of < –0.46 and 0.54–0.82, V/Cr ratios of < –2 and > 2, Ni/Co ratios of < –5 and > –5, and a Cu/Zn ratio of < –1 indicate oxic, suboxic to anoxic conditions (Hallberg, Reference Hallberg1976; Jones & Manning, Reference Jones and Manning1994; Xiong et al., Reference Xiong, Liu and Liang2015). Furthermore, low U and high Th values are related to the devitrification of volcanic glass and alteration of feldspar and precipitation of montmorillonite in pyroclastic rocks during diagenesis (Zielinski, Reference Zielinski1983).
The high concentrations of Ba (up to 3406 ppm), Sr (up to 8223.5 ppm), and Zr (up to 504.8 ppm) in the argillaceous sediments and tuff/tuffite exhibit alteration of volcanic ashes similar to those of the Franklin coal zone in Washington (USA) (Brownfield et al., Reference Brownfield, Affolter, Cathcart, Johnson, Brownfield and Rice2005). The enrichment of Cr (up to 1197.4 ppm), Co (up to 60.5 ppm), and Ni (up to 548.2 ppm) in the sandstone and argillaceous sediments compared to the felsic tuff and organic-rich shale and carbonate (including marl) suggest a detrital contribution from the mafic–ultramafic source units in the study area similar to those reported by Zou et al. (Reference Zou, Tian and Li2016) and Zhao et al. (Reference Zhao, Niu, Xie, Zhang, Zhou and Arbuzov2020). The enhancement of Cr, Co, and Ni values may suggest that these elements are associated with the Fe-bearing smectitic clay fraction and Fe-bearing sulfide via substitution of Fe by Cr, Co, and Ni in the octahedral site of smectite (Kadir et al., Reference Kadir, Aydoğan, Elitok and Helvacι2015).
The enrichment of LREE relative to HREE demonstrated a terrigenous or tuffaceous origin similar to those of the Permian and Jurassic coals, China (Shangguan et al., Reference Shangguan, Zhuang, Li, Li, Querol, Liu, Moreno, Yuan, Yang and Pan2020). Enhancement of REE (max. 983.2 ppm) downward in the sequence of the deposit is related to the increase in smectitic clay fraction in the sediments (Fig. 13). Scott et al. (Reference Scott, Deonarine, Kolker, Adams and Holland2015) and Li and Zhou (Reference Li and Zhou2020) reported that REE are accommodated mostly in the structures of smectites and kaolinite, in addition to adsorption by the clay fraction.
The positive Eu anomaly in acidic tuff may be related to the accumulation of feldspar released from the magma similar to that reported by Wolff et al. (Reference Wolff, Forni, Ellis and Szymanowski2020). In addition, the positive Eu anomalies in shale and sandstone/mudstone may be related to the hydrothermal fluids at temperatures of > 200ºC contributing to the transformation from Eu3+ to Eu2+ under the reducing environment during the formation of the peat and an oxidized depositional environment, respectively (Dai et al., Reference Dai, Graham and Ward2016; Jin et al., Reference Jin, Feng, Teng, Nie, Cao, Hou, Liu, Miao, Zhao, Chen, Zhu and Zhou2020; Mastalerz et al., Reference Mastalerz, Drobniak, Eble, Ames and McLaughlin2020). The positive Ce anomaly in argillaceous sediments of the Keles-Harmanalan deposit indicates precipitation under an anoxic environment similar to argillites in the Huainan Coal Basin, China (Table 2 and Fig. 12; Chen et al., Reference Chen, Liu, Wu and Sun2016), Ce3+ is diluted along with the other REE from underlying organic matter, and Ce3+ oxidized to Ce4+ during in situ precipitation conditions (Taunton et al., Reference Taunton, Welch and Banfield2000). The negative Ce anomaly in samples from the Orhaneli-Gümüşpınar deposit indicates leaching from argillaceous sediments by groundwater or hydrothermal fluid during post-depositional processes (Table 2 and Fig. 12; Chen et al., Reference Chen, Liu, Wu and Sun2016).
Stable isotope
The δ18O and δD values for the smectite and illite plot left of the supergene-hypogene line, close to the magmatic water box, suggesting the effect of hydrothermal activity during alteration (Sheppard & Gilg, Reference Sheppard and Gilg1996). The narrow δ18O and δD values reflect clay minerals’ formed during hydrothermal alteration due to magmatic water mixing with surface water (Arslan et al., Reference Arslan, Abdioğlu and Kadir2010). This suggestion is also supported by the occurrence of the Sadağ thermal spring-water located 6 km west of the Orhaneli-Gümüşpınar coal mines which have reservoir temperatures up to 119°C based on a silica geothermometer (Erkut, Reference Erkut2016) and the Orhaneli-Yapköydere hydrothermal Ni mineralization located 6 km north of the deposit (Örgün, Reference Örgün1993).
The low O-isotopic compositions of smectite (ranging from 1.39 to 5.09‰) from the mudstone and the organic-rich shale relative to the high values (range of 7.01–9.13‰) from the volcanic units suggest that the smectite has a detrital and authigenic origin. Similar low O-isotopic values of smectite (2.2–7.2‰) were also determined in siltstone and tuffite units from the Seyitömer coal deposit in western Anatolia (Erkoyun et al., Reference Erkoyun, Kadir, Külah and Huggett2017). The enrichment of the δ18O value in volcanic units may be related to the authigenic formation of smectite by oxygen isotope exchange between the glass shards and water similar to what was reported by Cuadros et al. (Reference Cuadros, Caballero, Huertas, de Cisneros, Huertas and Linares1999).
The negative correlation of δ18O with Fe2O3 in smectite indicates loss of Fe from the volcanic glass by increasing hydration resulting in the change of oxygen isotope composition during alteration (Compton et al., Reference Compton, Conrad and Vennemann1999). The depletion of δD in clays shows that hydrogen isotope exchange with meteoric waters or fluid flow processes, post-formation, is associated with tectonism (Uysal, Reference Uysal2000).
The wide range of δ34S values for pyrite and chalcopyrite in coal and tuff units of the Bursa-Orhaneli coal mines suggests that more than one phase of pyrite and chalcopyrite occurred during different phases of coalification under the effect of hydrothermal fluids similar to those reported by Lefticariu (Reference Lefticariu2009). The similar wide range between negative to positive δ34S isotope values of pyrite was also found in the fault gouge, siltstone, marl, and tonstein units from the Tunçbilek coal deposit (Erkoyun et al., Reference Erkoyun, Kadir and Huggett2019). Furthermore, the calculated formation temperature of 104.2–152.1°C for pyrite and chalcopyrite suggested a hydrothermal or magmatic contribution. The negative δ34S values for pyrite and chalcopyrite show that kinetic fractionation between sulfate and sulfide is related to the high sulfate ratio supply downward compared with the bacterial sulfate reduction and formation of framboidal pyrite in the upper parts of coal sections (Smith & Batts, Reference Smith and Batts1974). In contrast, positive δ34S values of pyrite show that the ratio of bacterial sulfate reduction is greater than the ratio of downward sulfate supply toward the bottom of the coal sections in the Orhaneli-Gümüşpınar and Keles-Harmanalan deposits similar to those of the pyrite and chalcopyrite sulfur isotopes in siltstone and marl from the Seyitömer coal deposit (Erkoyun et al., Reference Erkoyun, Kadir, Külah and Huggett2017), the Garrick coal seam in Australia, and the Bakerstown coal bed, USA (Lyons et al., Reference Lyons, Whelan and Dulong1989).
Conclusions
The detrital smectite input is accompanied by quartz, amphibole, and chlorite in fluvial and lacustrine sediments such as organic-rich shale, sandstone, and mudstone. On the other hand, the degradation of feldspar and devitrification of volcanic glass in tuff in the Orhaneli-Gümüşpınar and Keles-Harmanalan coal mines resulted in enrichment of Al, Fe, and Mg, and depletion of Si, all of which favored the formation of authigenic smectite under alkaline conditions via dissolution–precipitation mechanisms during diagenesis.
The development of smectite and smectite-edged illite as cement between detrital feldspar, amphibole, and quartz, and the close linkage between smectite flakes with resorbed feldspar and devitrified volcanic glass shards confirm the detrital and authigenic origin of smectite.
The presence of Bisaccat pollen, Botryococcus braunii, Intratripollenites instructus, Inaperturopollenites hiatus, and Subtriporopollenites simplex in organic-rich shale suggests a dry, warm to subtropical climate in fresh water and lakes in Late Eocene-Middle Miocene.
The average Al2O3/TiO2 ratios and TiO2/Ni diagrams suggest acidic-intermediate and a few sedimentary rock sources. SiO2 vs. (Al2O3 + K2O + Na2O) and V/(V + Ni), V/Cr, Ni/Co, and Cu/Zn ratios suggest arid–semiarid and oxic, suboxic to anoxic conditions.
The enrichment of LREE relative to HREE demonstrated a terrigenous or tuffaceous origin/provenance for the argillaceous sediments. The high ΣREE, positive Eu and positive/negative Ce anomalies suggest the fractional crystallization of feldspar and the contribution of hydrothermal fluids under a reducing environment. The δ18O and δD values of the smectite and illite fractions were formed during mixing of magmatic and surface waters.
The negative δ34S values for pyrite and chalcopyrite imply the reduction of sulfate to sulfide by a hydrothermal or magmatic effect under a temperature range of 104.2–152.1°C. There is also bacterial reduction as shown by the occurrence of framboidal pyrite.
Acknowledgements
This present study was supported financially by the Scientific Research Projects Fund of Eskişehir Osmangazi University in the framework of Project 2014-368. The authors are grateful to the anonymous reviewers, to Associate Editor Chun Hui Zhou, to the Editor-in-Chief Joseph W. Stucki, and to the Managing Editor, Kevin Murphy, for their extremely careful and constructive reviews and suggestions that improved the quality of the paper significantly. The preliminary and advanced stages of this paper were presented at the Euroclay 2019 conference, held in Paris, France, and the 17th International Clay Conference held in İstanbul, Turkey, respectively.
Authors contributions
Hülya Erkoyun: Field studies, Determination of the analyzed data, Drafting of the manuscript.
Selahattin Kadir: Field studies, Determination of the analyzed data, Drafting of the manuscript.
Tacit Külah: Field studies, Determination of the analyzed data, Drafting of the manuscript.
Funding
This present study was supported financially by the Scientific Research Projects Fund of Eskişehir Osmangazi University in the framework of Project 2014–368.
Data Availability
All data are available in the five Tables and 15 Figures included here.
Code availability
Not applicable.
Declarations
Conflicts of interest/Competing interests
The authors declare that there is no conflict of interest or competing interests.
Ethics approval and consents to participate
Not applicable.