Iron deficiency is the most common micronutrient deficiency in the world and continues to present a major burden to health in both low- and high-resource settings(Reference McLean, Cogswell and Egli1). Iron deficiency anaemia, reported in over 1.2 billion people in 2016, is one of the top five leading causes of years lived with disability globally and the leading cause of years lived with disability in low- and middle-income countries(2). Given the critical role of iron in the functioning of all cells and organ systems, reducing the prevalence of iron deficiency and anaemia globally is considered an urgent priority by the WHO(3).
Iron stores become depleted if dietary iron intake and/or absorption is inadequate or physiological losses through blood are uncompensated for. Iron deficiency occurs when iron stores are insufficient to meet the needs of an individual; therefore, individuals with increased iron requirements are at the greatest risk. Iron requirements are at their highest during the first 1000 days of life. They increase almost 10-fold during pregnancy, increasing from 0⋅8 mg/d in the first trimester to about 7⋅5 mg/d in the third trimester(Reference Bothwell4). This means close to 1000 mg of iron must be acquired during the pregnancy to preserve maternal iron balance and support fetoplacental development(Reference Fisher and Nemeth5). As infancy and early childhood is characterised by rapid growth and development, iron requirements per kg of body weight are higher from 6 to 24 months of age compared to during any other period of life(Reference Domellof, Braegger and Campoy6). Failure to meet these increased requirements predisposes pregnant women, infants and young children to iron deficiency and iron deficiency anaemia.
The aim of the present paper is to provide an in-depth review of the current perspectives on iron deficiency in the first 1000 days of life, with a particular focus on the key determinants of iron status during this period. The lasting consequences for neurological development are discussed, while challenges in defining and diagnosing iron deficiency in pregnant women, infants and young children are identified. Finally, suggestions are made for prevention and screening strategies to help tackle this global public health issue.
Iron deficiency in the first 1000 days
The first 1000 days arguably represents the period of life with the greatest risk of iron deficiency. In Europe, the prevalence of iron deficiency during pregnancy ranges from 28 to 85 %, with the highest rates reported in women in their third trimester and in those unsupplemented(Reference Milman, Taylor and Merkel7). Up to a third of pregnant women have iron deficiency anaemia in Europe, with higher rates reported in low- and middle-income countries, ethnic minorities and pregnant adolescents(Reference McLean, Cogswell and Egli1,Reference Milman, Taylor and Merkel7,Reference Marvin-Dowle, Burley and Soltani8) . Rates of iron deficiency anaemia are typically <5 % amongst 6–24-month-old children, although iron deficiency and depleted iron stores have been reported in up to half of European children in this age group(Reference Eussen, Alles and Uijterschout9,Reference van der Merwe and Eussen10) .
Dietary determinants of iron status
Inadequate dietary iron intakes and/or poor iron absorption are considered significant risk factors for iron deficiency during pregnancy and early childhood. Current dietary recommendations for the first 1000 days are presented in Table 1, with much variability observed across agencies due to differing assumptions about the efficiency of iron absorption and utilisation in these population groups.
Table 1. Dietary reference values for iron (mg/d) during the first 1000 days of life*
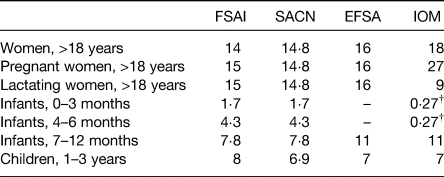
Important physiological adaptations in iron absorption and mobilisation occur during pregnancy, but women must still enter pregnancy with sufficiently large iron stores and consume a diet abundant in bioavailable iron during pregnancy to avoid iron deficiency(Reference Lynch, Pfeiffer and Georgieff11). However, inadequate dietary iron intakes and poor compliance with dietary guidelines are widely reported amongst pregnant women worldwide(Reference Harika, Faber and Samuel12,Reference Livock, Anderson and Lewis13) , with 60–100 % of pregnant women in Europe not meeting recommended intakes(Reference Milman14). To further compound this, many women begin pregnancy with already depleted iron stores as inadequate iron intakes are also common amongst women of reproductive age(Reference Milman, Taylor and Merkel7,Reference Milman15) .
The assumption is that healthy term infants are born with sufficient body iron stores to meet their requirements until they have doubled their birth weight, usually about 4–6 months of age(Reference Domellof16). As iron is transferred back from stores to the blood compartment to meet the infant's iron requirements, exclusive breastfeeding during this period, despite its low iron concentration, is sufficient to meet the needs of the infant(Reference Domellof, Braegger and Campoy6). It is only after this point that the infant becomes dependent on external dietary iron sources, as evidenced by the considerable increase in recommended intakes from 7 months onwards. Failure to incorporate sufficient iron-rich complementary foods into the diet and the early introduction and/or excessive intake of unmodified cow's milk are significant risk factors for iron deficiency in 6–24-month olds(Reference Thane, Walmsley and Bates17–Reference Agostoni, Decsi and Fewtrell19). Unfortunately, inadequate iron intakes are widely reported amongst infants and young children in Ireland(Reference McCarthy, Ní Chaoimh and Hourihane18,20) , the UK(21) and across Europe(Reference Eussen, Alles and Uijterschout9).
Non-dietary determinants of iron status
Even if dietary iron supply is adequate, there are several pregnancy-related and lifestyle factors that can compromise maternal–fetal iron supply in utero. Any disruption to maternal–fetal iron supply is especially detrimental to the developing fetus who is entirely dependent on maternal supply to meet its increased iron requirements for growth and development. Iron is actively transported from the mother to the fetus through the placenta(Reference Lynch, Pfeiffer and Georgieff11), with the iron-regulatory hormone, hepcidin, particularly critical at this time in controlling plasma iron concentrations and tissue iron distribution(Reference Ganz and Nemeth22). Maternal hepcidin concentrations are decreased in the second and third trimesters of healthy pregnancies to allow for an increased iron supply into maternal circulation to support fetal demand(Reference Fisher and Nemeth5,Reference Cao and O'Brien23) .
Disruption in maternal–fetal iron supply generally occurs through three key pathways: compromised maternal iron status, altered fetal iron delivery and/or demand or a reduction in fetal iron accretion. Critically, such disruption in iron supply to the fetus increases the risk of iron deficiency in the newborn infant, with 10–85 % iron deficiency reported in infants at birth, depending on the aetiology of the disruption(Reference Rao and Georgieff24). Infants born deficient are also at an increased risk of iron deficiency later in infancy and early childhood, as low iron stores at birth track into early childhood(Reference Hay, Refsum and Whitelaw25,Reference Georgieff, Wewerka and Nelson26) .
Compromised maternal iron status
Despite the earlier assumption that the fetus could accumulate enough iron independent of maternal iron status(Reference Cao and O'Brien23,Reference Rios, Lipschitz and Cook27,Reference Hussain, Gaafar and Laulicht28) , more recent evidence has emphasised the importance of maternal iron status to fetal and neonatal iron status. Infants born to mothers with iron deficiency with and without anaemia at delivery and/or mid-late gestation have lower umbilical cord ferritin concentrations at birth, indicative of poorer iron stores(Reference Hokama, Takenaka and Hirayama29–Reference Shao, Lou and Rao34). A maternal ferritin concentration of 12–13⋅6 μg/l has been suggested by some as the threshold below which fetal iron status is compromised(Reference Jaime-Perez, Herrera-Garza and Gomez-Almaguer33,Reference Shao, Lou and Rao34) . Maternal anaemia has also been shown to result in reduced neonatal Hb concentrations at birth in some cohorts(Reference Kumar, Rai and Basu35–Reference El-Farrash, Ismail and Nada37). Worryingly, this effect of maternal anaemia appears long-lasting(Reference Colomer, Colomer and Gutierrez38,Reference Kilbride, Baker and Parapia39) . Zhang and colleagues in China observed that maternal anaemia in the second trimester was associated with an increased risk of infant anaemia at both 5–7 and 11–13 months of ages(Reference Zhang, Jin and Liu40).
Disruption to fetal iron delivery and/or demand
Several pregnancy complications can result in a decrease in fetal iron delivery and/or an increase in fetal iron demand, thereby increasing the risk of iron deficiency in the newborn infant. Maternal hypertension, intrauterine growth restriction and gestational diabetes mellitus are characterised by intrauterine fetal hypoxia, which stimulates erythropoiesis and the production of Hb, thereby increasing fetal iron demand beyond the system's capacity(Reference Rao and Georgieff24). In pregnancies complicated by intrauterine growth restriction, approximately 10 % of all pregnancies, placental iron transport is also decreased due to uteroplacental vascular insufficiency, with reduced liver and brain iron concentrations observed in these infants(Reference Chockalingam, Murphy and Ophoven41,Reference Georgieff, Mills and Gordon42) . Similar findings are observed in infants of diabetic mothers; almost 65 % of these infants are born iron deficient with worrying evidence of brain iron depletion reported(Reference Petry, Eaton and Wobken43,Reference Siddappa, Georgieff and Wewerka44) .
In addition to these clinical complications, there are common lifestyle factors that can further disrupt maternal–fetal iron supply. Maternal smoking during pregnancy can induce fetal hypoxia, resulting in reduced cord ferritin concentrations at birth(Reference Hay, Refsum and Whitelaw25,Reference Sweet, Savage and Tubman32,Reference Chelchowska and Laskowska-Klita45,Reference McCarthy, Kenny and Hourihane46) . Although widely acknowledged as a risk to maternal and infant health(Reference Poston, Harthoorn and Van Der Beek47), only recently has maternal obesity both prior to and during pregnancy emerged as a considerable risk factor for iron deficiency. Maternal obesity is associated with poorer iron status, particularly low ferritin concentrations, in both pregnant women(Reference Jones, Zhao and Jiang48–Reference Flores-Quijano, Montalvo-Velarde and Vital-Reyes51) and their newborn infants(Reference McCarthy, Kenny and Hourihane46,Reference Jones, Zhao and Jiang48,Reference Dao, Sen and Iyer52–Reference Campbell, Tamayo-Ortiz and Cantoral54) . Although micronutrient deficiencies often coexist with obesity, termed the ‘double burden’ of malnutrition, the effect of maternal obesity on iron status is thought to be due to reduced iron absorption rather than just reduced dietary iron intakes(Reference Menzie, Yanoff and Denkinger55,Reference Charnley, Newson and Weeks56) . The low-grade, chronic inflammation associated with obesity is thought to result in an over-expression of hepcidin, inhibiting intestinal iron absorption and iron stores mobilisation, thereby reducing maternal–fetal iron supply(Reference Dao, Sen and Iyer52). However, further investigation into this mechanism is required, as some(Reference Jones, Zhao and Jiang48,Reference Garcia-Valdes, Campoy and Hayes49,Reference Flores-Quijano, Montalvo-Velarde and Vital-Reyes51,Reference Dao, Sen and Iyer52) but not all studies(Reference Flynn, Begum and White50,Reference Koenig, Klikuszowian and O'Brien57,Reference Jones, Shi and Lambrecht58) have observed elevated hepcidin and inflammatory marker concentrations in obese pregnant women. Additionally, a potential BMI threshold above which upregulation of hepcidin is induced has been suggested by some investigators recently(Reference Flynn, Begum and White50,Reference Jones, Shi and Lambrecht58,Reference Dosch, Guslits and Weber59) .
Reduction in iron accretion
The majority of fetal iron accretion occurs in the third trimester of pregnancy, therefore infants born premature miss out on this critical period of accretion(Reference Cao and O'Brien23,Reference Rao and Georgieff60) . Preterm infants have lower total body iron content, Hb and ferritin concentrations compared to term infants(Reference Halliday, Lappin and McClure61,Reference Lackmann, Schnieder and Bohner62) . Worryingly, this means that up to 50 % of preterm infants are either born iron deficient or will develop deficiency very early in infancy(Reference Berglund, Westrup and Domellof63–Reference Amin, Orlando and Eddins66). In addition to the impact of preterm birth itself, preterm infants have very high iron requirements given their high rate of postnatal growth and an earlier onset of erythropoiesis. They can also experience significant iron loss through uncompensated phlebotomy blood losses(Reference Rao and Georgieff60,Reference Lundstrom, Siimes and Dallman67,Reference Domellof and Georgieff68) . Similarly, low birthweight infants are born with low iron stores(Reference Domellof, Braegger and Campoy6,Reference Mukhopadhyay, Yadav and Kishore69) . In particular, extremely low birthweight infants of <1000 g can be in negative iron balance within the first month of life if an appropriate external iron source is not provided(Reference Shaw70). Timely, appropriate iron supplementation is, therefore, of the utmost importance to this vulnerable cohort, although much variability still exists with respect to iron dosing, duration of supplementation and delivery method in practice(Reference McCarthy, Dempsey and Kiely71).
Interestingly, although widely unacknowledged, obstetric mode of delivery can have a significant influence on the accretion of iron in the infant. Infants born by caesarean section have lower Hb, haematocrit and erythrocyte concentrations in peripheral and cord blood when compared to infants delivered vaginally(Reference Zhou, Li and Zhu72). In our own prospective maternal–infant cohort in Ireland, infants delivered by caesarean section were twice as likely to be iron deficient at birth in comparison with those delivered vaginally(Reference McCarthy, Kenny and Hourihane46). This effect is thought to be due to a shorter placental transfusion period because of immediate cord clamping and a weaker placental transfusion force, all reducing the transfer of iron to the infant through the umbilical cord at delivery(Reference Yao, Hirvensalo and Lind73,Reference Pisacane74) . Rates of deliveries by caesarean section have increased dramatically worldwide, with rates of 26–33 % reported in Ireland and the UK(Reference Boerma, Ronsmans and Melesse75,76) .
Neurological consequences of iron deficiency during the first 1000 days
The rate of growth and development of the brain is amongst the highest during the first 1000 days, making this period critical for immediate brain function but also for laying the foundations for later brain function(Reference Georgieff, Ramel and Cusick77). Fig. 1 illustrates the key milestones and processes that occur in brain development throughout the lifespan, with the importance of the early-life period particularly evident.
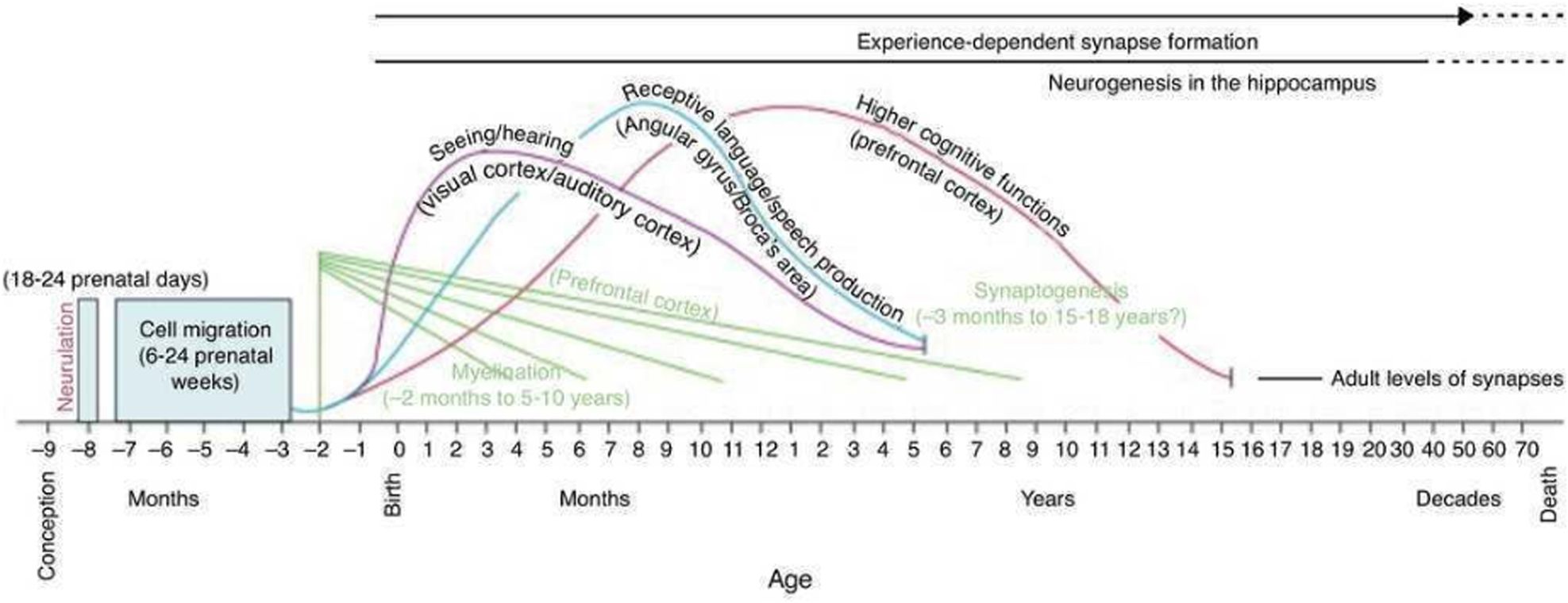
Fig. 1. Developmental milestones in human brain development. Copyright © 2001 by American Psychological Association. Reproduced with permission from Thompson and Nelson(Reference Thompson and Nelson138).
Iron deficiency during pregnancy and early-life has many health consequences for both the mother and her child, but the long-lasting neurological consequences are, perhaps, the most concerning. Consistent mechanistic evidence has shown that iron plays a key role in the fundamental neuronal processes of myelination and neurotransmitter and energy metabolism(Reference Lynch, Pfeiffer and Georgieff11). Iron deficiency can, therefore, disrupt these processes, resulting in adverse neurological consequences that often remain long after correction of the deficiency itself. Excellent reviews of the neurobiological effects of iron deficiency are provided elsewhere(Reference Lynch, Pfeiffer and Georgieff11,Reference Lozoff and Georgieff78,Reference Georgieff79) , with the focus of this review on the observational evidence underpinning the association between iron deficiency and brain development in early life.
The impact of maternal iron status on neonatal iron status has been discussed, but it can also present an immediate threat to fetal brain development. Monk and colleagues observed that low maternal iron intakes in the third trimester were associated with altered neonatal brain structure, particularly of the cortical grey matter(Reference Monk, Georgieff and Xu80). Using health and population register data from Sweden, the offspring of women diagnosed with anaemia in the first and/or second trimester of pregnancy were at an increased risk of developing neurological disorders such as autism spectrum disorder and attention-deficit/hyperactivity disorder(Reference Wiegersma, Dalman and Lee81). The significant variability in study design can make it difficult to interpret studies in this field, but a 2019 systematic review by Janbek et al. did conclude that maternal iron status during pregnancy may be associated with offspring cognition, academic achievement and behaviour(Reference Janbek, Sarki and Specht82). Since then, significantly higher scores in working memory and executive function at 7 years of age were observed in children born to mothers that had ferritin concentrations >12 μg/l in the first trimester in a large birth cohort in Spain(Reference Arija, Hernández-Martínez and Tous83).
The long-lasting consequences of postnatal iron deficiency, particularly from 6 to 24 months of age, are widely reported and acknowledged, with poorer cognition, intelligence, motor function and behaviour commonly observed(Reference Georgieff79,Reference Lozoff, Smith and Kaciroti84) . To date, little consideration has been given to the consequences of iron deficiency in the neonatal period. Neurophysiological disturbances are observed within 24–48 h of birth in infants born iron deficient (frequently defined as cord ferritin <70–76 μg/l), with abnormalities in the auditory system often reported(Reference Choudhury, Amin and Agarwal85,Reference Amin, Orlando and Wang86) . Neonatal iron deficiency is also associated with poorer recognition memory at 15 days old(Reference Siddappa, Georgieff and Wewerka44), poorer motor outcomes at 9 months(Reference Santos, Angulo-Barroso and Li87) and poorer language ability, fine motor skills and tractability at 5 years(Reference Tamura, Goldenberg and Hou88). We recently identified lasting behavioural consequences of iron deficiency at birth in our prospective, low-risk maternal–infant cohort, with this effect most apparent in the children born to mothers with obesity or delivered by caesarean section(Reference McCarthy, Murray and Hourihane89). This is concerning as we know early social-emotional development is considered an important determinant of future educational attainment, career and earning potential and overall quality of life(Reference Grantham-McGregor, Cheung and Cueto90).
Challenges in the diagnosis of iron deficiency
In contrast to other nutrients, there is no single biomarker that can truly assess the iron status of an individual or population. Iron status should be considered as a spectrum, moving from the early stage of depleted iron stores to iron deficiency to the final stage of iron deficiency anaemia. A wide range of biomarkers that reflect storage, transport, supply and functional iron are available to assess the different stages of iron status as outlined in Fig. 2. Additional indicators including hepcidin and reticulocyte Hb content are currently under investigation as potentially useful biomarkers in some populations(Reference Lynch, Pfeiffer and Georgieff11,Reference Pfeiffer and Looker91) . However, there are limitations to each biomarker, given that they are frequently confounded by other factors, particularly inflammation or lack specificity and/or sensitivity for iron. Difficulties in standardisation and harmonisation across different labs also present significant challenges to interpretation(Reference Hoofnagle92).
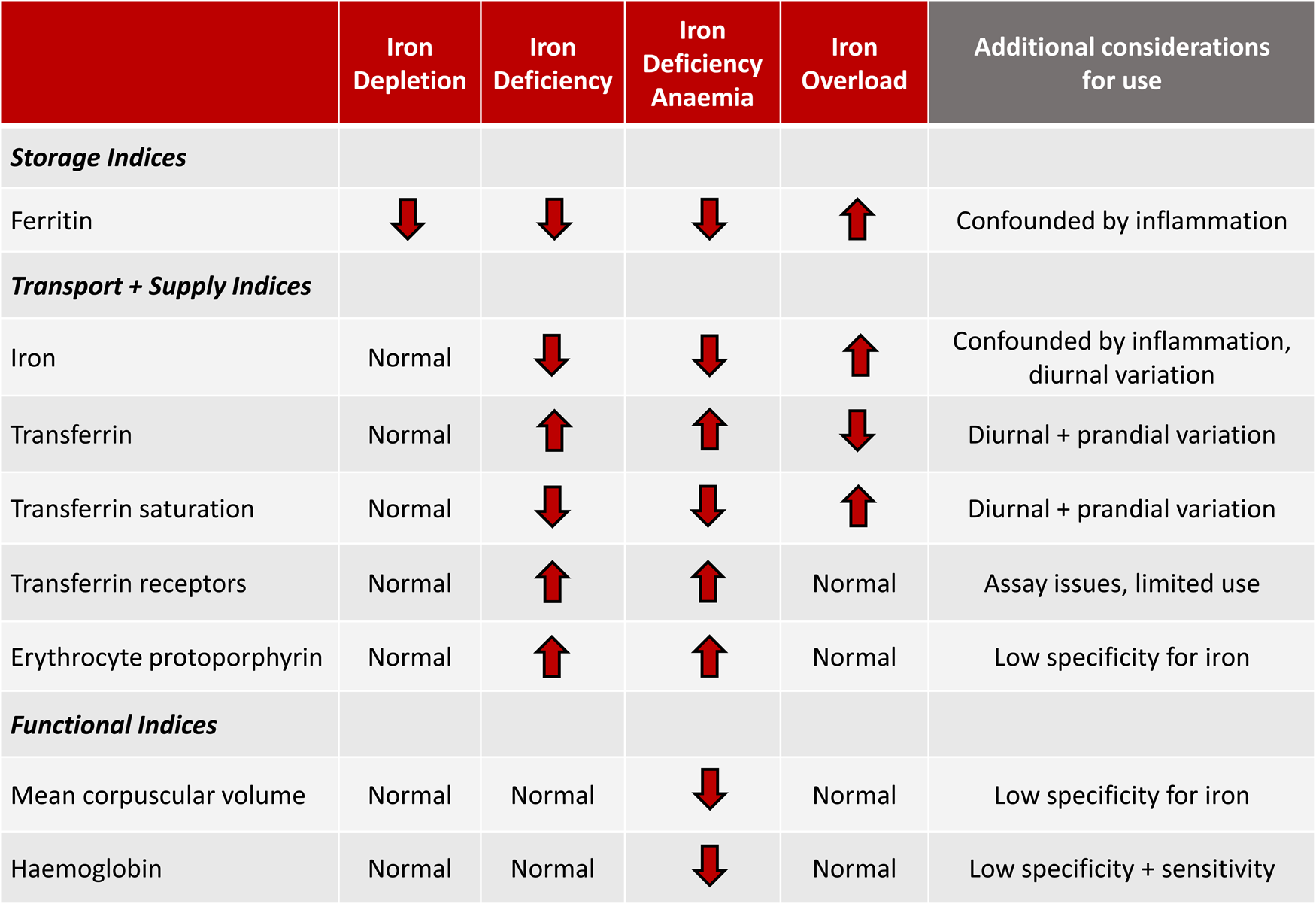
Fig. 2. Relationship of storage, transport, supply and functional iron indices to the spectrum of iron status. Modified from McCarthy and Kiely(Reference McCarthy and Kiely139).
The diagnosis of iron deficiency is further complicated in pregnant women, infants and young children, as serious knowledge gaps remain as to the most appropriate biomarkers and thresholds for this stage of life. Hb, a marker of functional iron is routinely employed in practice, but this is perhaps given the ease with which it can be measured with a point-of-care test. The over-reliance on Hb, particularly in this population is a major concern, as iron is prioritised to the erythrocytes for erythropoiesis above all other organs. The liver, heart, skeletal muscle and critically, the brain will all become iron deficient prior to any disturbances in Hb concentrations(Reference Petry, Eaton and Wobken43,Reference Georgieff93) .
Secondly, the thresholds applied to each biomarker are often not specific to this population and are not related to any relevant health outcomes. Currently used thresholds for many biomarkers are either extrapolated from other populations and do not account for the unique physiological adaptations in iron homoeostasis that occur during pregnancy and early infancy or are solely based on the distribution of a marker in a given population(Reference Brannon, Stover and Taylor94). This means many thresholds currently used are completely arbitrary and certainly not related to any meaningful health outcomes in this high-risk population. The huge variability and lack of consistency in the current use of thresholds, even amongst international agencies, further complicates matters.
Although much debate continues as to the most appropriate biomarkers and thresholds(Reference Daru, Allotey and Peña-Rosas95–Reference Domellof, Dewey and Lonnerdal97), health professionals, clinicians and researchers should aim to assess iron status using a battery of biomarkers, but at a very minimum, using both ferritin (with an inflammatory marker as it is an acute phase reactant) and Hb(98). The WHO recommend ferritin thresholds of 12 μg/l for children <5 years and 15 μg/l for everybody else, including pregnant women, with thresholds of 110 g/l for children <5 years and pregnant women for Hb(98,99) . Ferritin continues to be considered an important indicator of the earliest stage of iron deficiency, although some investigators have suggested adjustments to the thresholds applied in infants and young children(Reference McCarthy, Kiely and Hannon100,Reference Abdullah, Birken and Maguire101) . Research is also ongoing into novel biomarkers that may provide more sensitive indicators of impending brain dysfunction due to iron deficiency in infants and young children(Reference Rao, Ennis and Lubach102–Reference Marell, Blohowiak and Evans104).
Strategies to combat iron deficiency in the first 1000 days
Although the first 1000 days of life represents the period of greatest risk for iron deficiency, it also represents the period of greatest opportunity to tackle this global public health issue. Many of the risk factors outlined in the present paper are modifiable and thus preventable, whereas the impact of those that are not preventable could certainly be lessened through early identification. Interventions targeting the fetal and early-life period represent the best opportunity to prevent iron deficiency and its lasting consequences for health. Although several intervention targets could be considered, in this review, we have suggested three key targets that we feel are the most achievable and meaningful.
Target 1: improvements in nutrition and health status of women prior to conception
Many of the risk factors for iron deficiency in the first 1000 days are maternal or pregnancy-related. Therefore, interventions targeting the mother should be considered as one of the best ways to prevent iron deficiency in infancy and early childhood. As a starting point, poor micronutrient status and obesity are the major challenges that need to be addressed by any such interventions.
To combat the widespread issue of iron deficiency, iron supplementation is commonly used, as daily supplementation has been shown to reduce the prevalence of iron deficiency and iron deficiency anaemia in pregnant women at term(Reference Pena-Rosas, De-Regil and Garcia-Casal105). However, the positive effect of supplementation during pregnancy outside of this, for neonatal iron status or health outcomes remains very much unclear(Reference Domellof, Braegger and Campoy6,Reference Pena-Rosas, De-Regil and Garcia-Casal105) . Moreover, compliance with supplementation strategies is often poor, particularly in low- and middle-income countries and untargeted supplementation can be dangerous(Reference Wessling-Resnick106). Taking all of these into consideration, it is likely that starting supplementation during pregnancy is too late to influence long-term health outcomes in the offspring, so strategies to improve nutrient intakes and status in girls and women prior to conception are more pertinent.
Changes in BMI require an even earlier intervention than that required to improve the nutritional status of women prior to pregnancy. Lifestyle and behavioural interventions amongst pregnant women with overweight and obesity have been shown to improve dietary intakes and physical activity levels(Reference Ainscough, O'Brien and Lindsay107–Reference Poston, Bell and Croker109). However, for the most part, such interventions have not resulted in improved clinical outcomes in the mothers or their offspring(Reference Poston, Bell and Croker109,Reference Louise, Poprzeczny and Deussen110) . A life course approach has been suggested as a better alternative, whereby the prevention of obesity prior to conception is recommended, with a focus on a healthy weight status beginning in adolescence and right through the childbearing years(Reference Hanson, Barker and Dodd111,Reference Barker, Dombrowski and Colbourn112) . An integrated approach is required to achieve this, composed of community-based awareness initiatives and education programmes targeting adolescent girls, women of reproductive age, women and couples planning a pregnancy and those not planning but still able to conceive.
Target 2: consistent, widespread employment of delayed clamping of the umbilical cord
After birth, placental transfusion continues with a net transfer of blood, along with erythrocytes, stem cells and plasma through the placenta to the newborn infant(Reference Andersson and Mercer113). Clamping of the umbilical cord stops this transfer, with varying practices in the timing of cord clamping reported.
Delayed clamping of the umbilical cord, considered by many to be 1–3 min after birth or after cord pulsations stop, will allow for a greater placental transfusion than if the cord was clamped immediately. This increased placental transfusion results in increased Hb, haematocrit and ferritin concentrations after birth in both term(Reference Hutton and Hassan114,Reference McDonald, Middleton and Dowswell115) and preterm infants(Reference Ranjit, Nesargi and Rao116–Reference Ultee, van der Deure and Swart118). These benefits are long-lasting with improved iron stores and a decreased risk of iron deficiency observed throughout infancy, up to 8–12 months of age(Reference Andersson, Hellstrom-Westas and Andersson119–Reference Mercer, Erickson-Owens and Deoni122). An increased risk of jaundice requiring phototherapy in infants receiving delayed cord clamping has been suggested as a potential risk of this practice(Reference McDonald, Middleton and Dowswell115), but a recent review by Andersson and Mercer stresses that this conclusion is exaggerated and not evidence based(Reference Andersson and Mercer113). Furthermore, improved neurological outcomes have been observed following delayed cord clamping, with increased brain myelination at 4 months and improved fine motor and social development at 4 years reported(Reference Mercer, Erickson-Owens and Deoni122,Reference Andersson, Lindquist and Lindgren123) .
Delayed cord clamping, albeit with varying definitions about timing, is recommended for all term neonates, regardless of the mode of delivery, by multiple professional bodies worldwide(124–126). The WHO also recommend delayed cord clamping for preterm infants, where possible(124), although this can be difficult given the complicated nature of many preterm deliveries. As preterm infants are especially vulnerable to iron deficiency, efforts are now being made to allow for the incorporation of delayed cord clamping into the stabilisation procedures of these infants in the delivery room(Reference Knol, Brouwer and Vernooij127). Despite consistent evidence to support the benefits of delayed cord clamping and recommendations from professional bodies, the practice of delayed cord clamping is not widespread or even consistent within countries and regions(Reference Winter, Macfarlane and Deneux-Tharaux128,Reference Devin and Larkin129) .
Target 3: development of appropriate screening strategies to enable early detection
When secondary to preterm birth and some pregnancy complications, prevention of iron deficiency may not always be feasible. Therefore, strategies targeting both prevention but also screening are needed to reduce the risk of iron deficiency and its lasting health consequences. Screening during the first 1000 days will allow for the early detection of iron deficiency, thereby enabling prompt and targeted treatment to prevent its associated neurological consequences.
Current screening strategies to tackle the issue are either non-existent, too limited or totally inappropriate to protect the developing brain. There are currently no screening strategies for the early detection of iron deficiency in pregnant women, infants or young children in Ireland. Some assessment of iron status is undertaken in pregnant women and hospitalised preterm or low birth weight infants, but this frequently relies on Hb concentrations to indicate the need for further investigation and tests. The American Academy of Pediatrics recommend universal screening of infants at 12 months of age using Hb concentrations(Reference Baker and Greer130). In 2015, the US Preventive Services Task Force concluded that there was insufficient evidence to assess the benefits and harms of screening for iron deficiency anaemia in pregnant women and children aged 6–24 months(Reference Siu131,Reference Siu132) . In contrast, the recent UK guidelines on the management of iron deficiency in pregnancy outline that Hb should be routinely measured about 28 weeks' of gestation and followed up with an assessment of ferritin concentrations, if anaemia detected(Reference Pavord, Daru and Prasannan133).
Future screening strategies need to be appropriately timed, incorporate the most relevant and meaningful biomarkers and identify those at the highest risk. Many questions remain as to the most appropriate biomarkers for use in this population group, but a move away from relying solely on Hb to screen for risk is warranted. However, this does require further development of other biomarkers and better education as to why the use of Hb for such purposes does not protect the developing brain. Perhaps, screening tools that identify individuals as high-risk based on their own and their mother's clinical history and past exposures/risks are a stepping stone towards the development of a much-needed screening programme. Without such a screening programme, iron deficiency and its long-lasting neurological consequences will continue to threaten those most vulnerable.
Conclusions
The first 1000 days of life represents the period of greatest risk for iron deficiency and its long-lasting neurological consequences. Inadequate dietary intakes prior to and during pregnancy can be compounded by several pregnancy-related and lifestyle factors that disturb maternal–fetal iron supply in utero. Unfortunately, this means that many of the commonly held assumptions during this period, particularly pertaining to women and newborn infants having sufficient iron stores to meet their increased requirements do not always hold true. To further complicate matters, serious questions remain as to the most appropriate biomarkers and thresholds for the diagnosis of deficiency in this population, with re-evaluation of the diagnostic criteria necessary. There continues to be a lack of research into this area, with trimester-specific ferritin thresholds during pregnancy one area that needs urgent attention to enhance our ability to identify the women at most risk.
The lasting neurological consequences of iron deficiency represent a real cost and burden to individuals, but also wider society. Therefore, the earlier we can protect the developing brain from the consequences of suboptimal iron, the better it is for our society's long-term health and prosperity. To do so, a dual approach encompassing both prevention and screening strategies must be adopted. Prevention strategies need to focus on improving the health and nutritional status of young women, prior to ever becoming pregnant, while delayed cord clamping should be considered a priority in the obstetric field. Better screening strategies, incorporating screening tools and point-of-care tests, are needed, to facilitate the early detection and identification of those at the greatest risk. These targets need to be achieved to protect both maternal health and the developing brain.
Financial Support
E. K. M. holds a Health Research Board of Ireland Applying Research into Policy and Practice Fellowship (Iron deficiency assessment for protection of the newborn brain (ARPP-25 2020-008)).
Conflict of Interest
None.
Authorship
The authors had sole responsibility for all aspects of preparation of this paper.