African Buffalo and Diseases, and the Villain is…
All iconic wildlife species trigger an array of positive and negative feelings, beliefs and values in humans. The lion is the king of the savanna, powerful and dangerous but benevolent towards his subjects. The African buffalo, on the other hand, is aggressive, naughty and treacherous. These perceptions do not only draw their strength from the ferocious opponent that the buffalo represents when hunted. They also are deeply rooted in the perceived relationship between the buffalo and infectious diseases, and the negative representations associated with the buffalo as a villainous animal spreading pathogens to livestock. In Africa, and perhaps even beyond, no other species crystallizes so many of perceptions about the health risks that need to be controlled to produce livestock. Managing buffalo populations, by culling them, physically disconnecting them from livestock and protecting livestock from their diseases, has been one of the priorities of colonial and post-colonial veterinary services since their creation at the end of the nineteenth century. This section of the book aims to review the current state of knowledge on the relationship between the buffalo and infectious diseases, and then to reflect on old paradigms and opportunities provided by new knowledge and global contexts.
To do so, we first needed to present recent data and synthetize knowledge about the role of buffalo in infectious diseases in Africa. The buffalo is a species that co-evolved with African pathogens and their vectors, developing resistance and tolerance mechanisms that has allowed it to survive across the continent in different habitat types. However, it is far from clear that the buffalo is a maintenance host for all or many endemic pathogens. Gaps in knowledge still exist despite the massive amount of work that has been done on the species. With regard to European and Asian pathogens that have been imported into Africa since the beginning of the colonial era, buffalo have once again proved to be a quite resistant species. The one exception was the deadly and now extinct rinderpest, which hammered buffalo populations in Africa. The picture that emerges from this section is that compared to exotic cattle breeds, the buffalo is a quite robust species well adapted to the African terrain. As a result, in the current intensive livestock production systems promoted in Africa, the buffalo needs to be separated from livestock because of its maintenance of some diseases detrimental for livestock production. Does the same hold for extensive subsistence farming systems?
The last decades have seen the emergence of a relatively larger number of behavioural studies looking at the buffalo/cattle interface in relation to pathogen ecology. These studies have been concentrated in southern and eastern Africa and are largely absent in other regions. They require interdisciplinary collaboration bridging ecology, spatial epidemiology and social sciences, among others. The replication of these studies in different ecosystems has indicated that buffalo tend to avoid cattle, leading to very few observations of direct contacts (only the odd story of a buffalo bull hanging around a cattle herd, but probably not a common event). Buffalo tend to use similar grazing and water sources because cattle are penned (‘corralled’, ‘kraaled’) at night, adapting their behaviour to the contexts of wildlife/livestock interfaces. This means that potential interspecies transmission is more likely to involve pathogens that are indirectly transmitted (e.g. through the environment or vectors). This new science of the wildlife/livestock interface still needs some development. More data-heavy modelling will help the testing of management options for these interfaces, such as manipulating key resources (e.g. water, grazing) or strategically controlling the disease in cattle (e.g. seasonal vaccination protecting cattle when contacts with buffalo are the highest). The integration of non-invasive tools and genomics should considerably impact the understanding of these buffalo/cattle interfaces.
The entanglement of the buffalo in grievances because it was thought (unjustifiedly) to cause sanitary issues had the advantages of concentrating a huge amount of disease ecology work on the species. Today, the buffalo belongs to the top five species in the world studied for in vivo coinfection. Its gregarious social organization and phylogenetic proximity with cattle, with whom they share a large part of their pathogen burden, makes the buffalo a formidable subject to investigate the little-known interactions of important viruses, mycoplasmas, other bacteria and parasites in coinfected individuals and populations. Interesting coinfection properties emerge from these studies that emphasize potential cooperation or competition between pathogens through the intermediary of the host immune systems and the relevance of the infection history of the host to determine the community of pathogens that it harbours. The knowledge and hypotheses produced by these studies can inform not only the management of buffalo and buffalo/cattle interfaces (e.g. shall we manage pathogens in buffalo populations or not), but also the nascent field of pathogen community ecology.
After weighing and sifting through the evidence, it would appear that the African buffalo is probably not the health villain invented by colonial administrations. Its coexistence with Western livestock production systems is probably impossible and has been fought against during the twentieth century. But what have been the costs and benefits for African societies? Is the species that is well-adapted to the African environment the problem, or is it the imported breed that needs to be kept under unsustainable conditions to stay productive? The twenty-first century has started with the prospect of massive global changes for the century to come that will threaten societies and their productive systems. There is a chance that Africans and African societies could emerge stronger from this challenging era, and the buffalo could be a flagship of this transformation.
Introduction
Infections in free-ranging African wildlife with pathogens normally associated with wild animals or domestic livestock can arbitrarily be assigned to four or possibly even five categories, as follows.
Wildlife-maintained infections: African indigenous infections without disease expression in sylvatic hosts, such as South African Territory (SAT) types of foot and mouth disease (FMD), African theileriosis, African swine fever, African horse sickness, bovine malignant catarrh fever and African trypanosomiasis.
Alien, exotic or foreign animal infections: some of the best examples of this category are certain pathogens historically alien to sub-Saharan Africa that were probably introduced onto the African continent with the importation of domestic livestock from Eurasia during the colonial era. Indigenous African free-ranging mammals, within similar taxonomic groupings to these domesticated hosts, are generally immunologically naïve to these foreign pathogens, and may suffer significant morbidity and mortality when exposed to these disease agents. Examples include rinderpest (may it rest in peace), bovine tuberculosis, bovine brucellosis, peste des petits ruminants (PPR), bovine papillomatosis and canine distemper, which may impact both wildlife and domestic species.
Multi-species infections: these infections usually occur on most continents and may affect both wildlife and domestic livestock. Transmission can thus occur in both directions, although certain regional dominant role players have been identified. These infections are generally cyclical in nature, and the epidemic cycles appear to be related to population densities of one or more host species, as well as climatic factors. Uniquely, these infections generally have a fatal outcome in both wildlife and domestic livestock and are frequently zoonotic. Some of the these diseases, such as anthrax and rabies, have been documented in African buffalo (Syncerus caffer).
Newly emerging or recently detected infections: these infections have been detected only recently, such as encephalomyocarditis in elephants (Loxodonta africana), arthropod-borne flavi- and bunyaviruses in several wildlife species, parafilariasis in African buffalo and feline immunodeficiency virus infection in wild felids. They also include infections that have relatively recently crossed the species barrier, such as canine distemper in lions (Panthera leo) and bovine papillomatosis in giraffe (Giraffa camelopardalis), zebra (Equus zebra), sable antelope (Hippotragus niger) and buffalo.
Finally, truly novel diseases such as spongiform encephalopathies seen in cervids in North America and more recently in Norway and Sweden, but not yet seen in African wildlife.
Infectious Diseases Impacting African Buffalo
Rinderpest
Rinderpest, the great cattle plague pandemic of 1889–1905, was introduced with infected cattle from southern Asia to feed troops on military expeditions around the Horn of Africa (Hutcheon, Reference Hutcheon1902). Much has been written about the massive die-offs of both indigenous cattle, wild ruminants and suids across most regions of Africa, and the massive social, political and economic repercussions resulting from the huge livestock losses (Mack, Reference Mack1970). The livelihoods of nomadic pastoralists were devastated. Countless wild artiodactyls also perished, with buffalo, tragelaphs (spiral horned antelope), wildebeest, hippotragines and wild suids being the most severely affected (Kock, Reference Kock, Barret, Pastoret and Taylor2006). Being social ruminants that occur in large herds, buffalo were massively affected by this close-contact transmitted morbillivirus, and in many cases, only small relict populations survived in remote pockets or were entirely extirpated from their former ranges (Stevenson-Hamilton, Reference Stevenson-Hamilton1957). Impacts were also seen on some keystone species, including migrating East African wildebeest (Connochaetes taurinus). This disease had major impacts on the scale of migration and the habitat, with resultant transformation in vegetation types and distribution (Holdo et al., Reference Holdo, Sinclair and Dobson2009). This disease may have contributed to certain wildlife distribution anomalies, such as the formation of isolated metapopulations of species such as sable antelope, roan antelope (Hippotragus equinus), greater kudu (Tragelaphus strepsiceros) and nyala (Tragelaphus angasii). Rinderpest was declared officially eradicated in 2011, the first animal disease to be so (FAO report, 2011).
Bovine Tuberculosis
Bovine tuberculosis (bTB) in wildlife in sub-Saharan Africa is probably a foreign animal disease introduced into southern Africa with colonial cattle breeds imported from Europe during the eighteenth and nineteenth centuries. The first report of bTB in wildlife in southern Africa was in greater kudu and common duiker (Silvicapra grimmia) in the eastern Cape Province of South Africa (Paine and Martinaglia, Reference Paine and Martinaglia1929), and then again in greater kudu in the same region in 1940 (Thorburn and Thomas, 1940). This region apparently had a high prevalence of bTB in cattle at that time. The first report of bTB in African buffalo came from the Queen Elizabeth National Park (NP) in Uganda (Guilbride et al., Reference Guilbride, Rollinson, McAnulty, Alley and Wells1963), and confirmed in 1965 (Thurlbeck et al., Reference Thurlbeck, Butas, Mankiewicz and Laws1965). The disease spilled over into common warthogs (Phacochoerus africanus) and has persisted to this day (Woodford, Reference Woodford1982a, Reference Woodford1982b). BTB was also discovered in free-ranging Kafue lechwe (Kobus leche kafuensis) in Zambia in 1972 and 1977 (Gallagher et al., Reference Gallagher, Macadam, Sayer and van Lavieren1972; Clancey, Reference Clancey1977), and confirmed later (Munyeme et al., Reference Munyeme, Muma and Siamudaala2010). Subsequent screening of buffalo from the Greater Kafue NP yielded nothing (Munang’andu et al., Reference Munang’andu, Siamudaala and Matandiko2011). In South Africa, the disease was first detected in buffalo in the Hluhluwe/Imfolosi Park in Kwazulu-Natal Province in the 1980s, and in Kruger (KNP) in 1990 (Bengis et al., Reference Bengis, Kriek and Keet1996). It appears as though these wildlife populations became infected by contact with infected cattle. The outbreak in Kruger followed recorded outbreaks of bTB in cattle on farms south of the Crocodile River (southern boundary of KNP) during the early 1960s, and again in the 1980s (State veterinary reports). During these periods, outbreaks of buffalo-associated theileriosis were also reported in cattle on these farms, indicating that buffalo and cattle had shared range and vector ticks. Subsequently in Kruger, the disease spread through all of the buffalo herds from the extreme south to the far north of the park, and eventually into Zimbabwe (Gonarezhou National Park), over a period of 19 years (de Garine-Wichatitsky et al., Reference de Garine-Wichatitsky, Caron and Gomo2010; Figure 9.1). Systematic sampling of buffalo herds in the different regions of the KNP between 2003 and 2011 demonstrated a gradient of the prevalence of infection, with a 35 per cent prevalence in the south where the disease first entered, 20 per cent in the central district and 3 per cent in the far north. Spillover infection also has been detected in 15 other species. Only buffalo and lechwe appear to have become true sylvatic maintenance hosts of bTB, while greater kudu and warthog appear to have maintenance host potential, if their population densities are high enough. The long-term effects of this chronic progressive disease on African wildlife host populations at sustained high prevalence rates are unknown. Initially there was concern that bTB may negatively affect the population dynamics and structure of buffalo herds and lion prides in KNP. To date, however, no negative impact has been detected at the population level in Kruger, illustrating the resilience of these species. However, due to the veterinary control measures imposed, this disease has definitely had an impact on translocation of wildlife out of what are now endemic bTB areas in South Africa. The disease has also been reported in buffalo, warthogs, Uganda kob (Kobus kob thomasi) and olive baboon (Papio anubis) in Uganda, and in buffalo, Masai giraffe (Giraffa camelopardalis tippelskirchi) and topi (Damaliscus lunatus jimela) in the greater Serengeti Ecosystem and in Kenya (Meunier et al., Reference Meunier, Sebulime, Whiteand and Kock2017). These are unfenced systems and the prevalence of disease appears to be relatively stable (Meunier, Reference Meunier2017), although no intensive sampling has been reported. In Ethiopia, although bTB is endemic in cattle, no infection was detected in a small sample of buffalo from Omo/Mago NP, also an open system.

Figure 9.1 Advanced pulmonary tuberculosis in African buffalo.
Brucellosis
In the African wildlife context, only Brucella abortus bovis and B. melitensis have been recorded in wildlife (Simpson et al., Reference Simpson, Thompson and Saegerman2021). Both these organisms have zoonotic potential, although no cases of human infection from buffalo have been reported.
Bovine brucellosis caused by B. abortus bovis is a foreign chronic animal disease thought to have been introduced into sub-Saharan Africa by the importation of European cattle breeds by colonial settlers during the eighteenth and nineteenth centuries. This chronic disease has also crossed the interface with wildlife, and is now endemic in wildlife in several countries. In sub-Saharan Africa, Brucella sero-positivity has been demonstrated in bovids, 12 antelope species, four carnivore species, baboons, black and white rhinoceros, hippopotamus (Hippopotamus amphibius) and zebras. Of all of these, only African buffalo and possibly Kafue lechwe appear to be reservoir species that are able to maintain infection in the absence of infected cattle (Simpson et al., Reference Simpson, Thompson and Saegerman2021).
In KNP, Brucella sero-positivity has been detected in most buffalo herds (De Vos and van Niekerk, Reference De Vos and van Niekerk1969), and at sero-prevalence rates of up to 23 per cent (Herr and Marshall, Reference Herr and Marshall1981). Similar to the disease in cattle, experimental infection with brucellosis causes late-term abortions in buffalo heifers, as well as in buffalo cows during the first pregnancy after infection (Gradwell et al., Reference Gradwell, Schutte, Van Niekerk and Roux1977). In addition, it was observed that calves born through the following pregnancy tend to be weak and poor survivors. Brucellosis in buffalo may also affect synovial structures causing arthritic conditions such as carpal hygromata, and may cause severe lameness (Figure 9.2). This has been observed in both eastern and southern African buffalo populations (Kock, personal communication). In Zimbabwe, clinical disease also has been reported in eland and waterbuck (Condy and Vickers, Reference Condy and Vickers1972). Brucellosis caused by B. melitensis has only been detected in farmed sable antelope on several Eastern Cape wildlife ranches (Glover et al., Reference Glover, Macfarlane and Bengis2020).

Figure 9.2 Brucella Carpel Hygroma in African buffalo.
Anthrax
Anthrax is historically one of the oldest documented diseases, and the life cycle of the causative bacterium, Bacillus anthracis, has both biotic and abiotic components. The abiotic component is the resistant dormant spore phase, which occurs in regions with predominantly alkaline soils and high calcium and moisture content. These spores can survive almost indefinitely in this dormant state in the soil. The biotic component is the exponential amplification phase, which takes place within the mammalian body, and appears to be the essential reproductive phase (De Vos and Turnbull, Reference De Vos, Turnbull, Coetzer and Tustin2004), although germination and amplification of anthrax bacteria in certain soil-dwelling Amoebas has been reported (Dey et al., Reference Dey, Hoffman and Glomski2012). Anthrax outbreaks have been documented in most domestic species in the absence of a wildlife link. Similarly, localized to extensive outbreaks have occurred in various wildlife populations with no livestock link. Large-scale outbreaks may cross the interface, especially where livestock and wildlife share ranges and resources (Mukarati et al., Reference Mukarati, Matope and de Garine-Wichatitsky2020). With regard to buffalo, anthrax has been reported in buffalo populations in KNP, in Gonarezhou NP in Zimbabwe, in the Okavango ecosystem in Botswana, in the Caprivi system in Namibia and in Luangwa valley NP in Zambia. In East Africa, anthrax has been recorded in Uganda, Tanzania, Kenya and Ethiopia. In Kenya, an anthrax outbreak in 2015 at Lake Nakuru NP resulted in the death of 745 out of the 4500 buffalo in the park, with a species-specific mortality rate of 17 per cent (Muturi et al., Reference Muturi, Gachohi and Mwatondo2018). An analysis of temporal and spatial distribution of anthrax outbreaks among Kenya wildlife revealed that out of the 51 outbreaks, 23.5 per cent involved buffalo (Gachohi et al., Reference Gachohi, Gakuya and Lekolool2019).
In Mago NP in Ethiopia, anthrax outbreaks originating from cattle and sheep from nearby pastoralists killed more than 1617 (in 1999) and 563 (in 2000) wild animals from 21 species, including buffalo. About 20 people, including one scout, from the area who handled or ate the carcasses, including one scout, were infected and developed severe skin lesions, but recovered after treatment (Shiferaw et al., Reference Shiferaw, Abditcho, Gopilo and Laurenson2002).
In a review of seven documented anthrax outbreaks in KNP between 1960 and 2010, buffalo featured as the second most common species affected in an analysis of the carcass counts. Greater kudu were the most frequently affected species in these Kruger outbreaks (Bengis, personal communication; Figure 9.3).
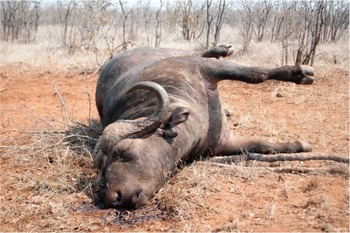
Figure 9.3 Anthrax in African buffalo. Note blood coming from nose and generalized putrefactive swelling.
In Africa, human anthrax is generally contracted when people handle or consume carcasses of infected livestock or of any wildlife that have succumbed during an outbreak, and this would include buffalo in light of the susceptibility of this species as mentioned.
Buffalo appear to become infected mainly by ingestion of contaminated water, contaminated grazing or by mechanical transmission by biting flies. Buffalo are exquisitely sensitive to the exotoxins produced during the exponential replication phase of the anthrax organisms, and unlike other ruminants, will often die before developing substantial bacteremia. This makes it important for laboratories to microscopically scan buffalo diagnostic blood smears thoroughly if this disease is suspected. The post-mortem signs commonly seen with buffalo that have died of anthrax include sudden death, tarry black blood that does not clot exuding from the natural orifices and swollen carcasses from rapid putrefaction. Suspected anthrax carcasses should not be opened.
Other Bacterial Diseases
There are several other bacterial diseases that have been sporadically reported in African buffalo. Localized bacterial abscesses caused by a variety of organisms do occur, usually as a result of bacterial infection of penetrating wounds. With the exception of anthrax, bacterial infections that cause systemic disease have rarely been reported in free-ranging buffalo. Those that have been detected include haemorrhagic septicaemia caused by Pasteurella multocida and bacterial entero-colitis caused by entero-pathogenic Escherichia coli or Salmonella spp. (Mitchell et al., Reference Mitchell, Steyl and Woodburn2021). In addition, malignant oedema/gas gangrene, caused by toxigenic Clostridia, have been sporadically reported following blunt traumatic injury and bruising.
Buffalo and Other Wildlife-Maintained Infections
In this discussion, it is important to realize that only a few wildlife species have been identified and positively implicated in the maintenance and transmission of these diseases. These include the African savanna buffalo, wild suids including warthogs and bush pigs, brindled wildebeest, black wildebeest (Connochaetes gnou), bushbuck, the various species of zebra and possibly greater kudu.
Foot-and-Mouth Disease
In the case of FMD in sub-Saharan Africa, cattle-derived imported Eurasian serotypes A and O coexist with African serotypes SAT1, 2 and 3 (SAT for South African Territory). The pivotal role played by the African buffalo as a sylvatic maintenance host of the SAT group of FMD viruses was identified in the late 1960s, and initial findings in various buffalo populations were published by Condy (Reference Condy1971), Hedger (Reference Hedger1972) and Falconer and Child (Reference Falconer and Child1975). This was followed by further publications on the characterization of the viruses in several buffalo subpopulations in eastern and southern Africa (Anderson et al., Reference Anderson, Doughty, Anderson and Paling1979; Vosloo et al., Reference Vosloo, Bastos and Kirkbride1996; Thomson and Bastos, Reference Thomson, Bastos, Coetzer and Tustin2004). Most buffalo populations in southern Africa, with the exception of those relict populations that survived the rinderpest in the Eastern Cape and Kwazulu/Natal Provinces of South Africa, have been shown to be infected. Random serological sampling of buffalo herds in KNP in South Africa, the Okavango delta in Botswana and the Zambezi valley in Zimbabwe demonstrate that over 95 per cent of those free-ranging buffalo have antibodies to at least one, but frequently two or even all three SAT virus serotypes, by the age of 12 months. Surveys in West, Central and East Africa showed a similar picture (Bronsvoort et al., Reference Bronsvoort, Parida and McFarland2008). In addition, FMD viruses of all three serotypes can regularly be isolated from oro-pharyngeal probang samples collected from these buffalo, and this carrier state may last for years (Jolle et al., Reference Jolle, Gorsich and Gubbins2021). The virus apparently survives almost indefinitely in certain dendritic cells of the tonsils, even in the presence of humoral antibodies. Most free-ranging buffalo show no clinical signs of infection.
Thus, until recently, there could be no doubt that infected buffalo herds were the ultimate source of all FMD infections in naive livestock, and most SAT-type FMD outbreaks in livestock could also be traced back to interface buffalo contact (Guerrini et al., Reference Guerrini, Pfukenyi and Etter2019). However, more recently, it would appear that certain SAT virus topotypes have adapted to cattle, and several outbreaks have recently occurred in South Africa without any identifiable buffalo contact (Thomson, personal communication). This has been confirmed in Kenya (Wekesa et al., Reference Wekesa, Sangula and Belsham2015). They found that FMDV serotypes O, A, SAT1 and SA2 were circulating among cattle in Kenya and cause disease, but only SAT1 and SAT2 viruses were successfully isolated from clinically normal buffalo. The buffalo isolates were also genetically distinct from isolates obtained from cattle. In another study in Kenya, Omondi et al. (Reference Omondi, Gakuya and Arzt2020) investigated the epidemiology of FMDV in buffalo, including the role of buffalo in the circulation of FMDV in livestock populations. By sequencing the virus’ VP1 coding region from blood and oropharyngeal fluids collected from wild buffalo and sympatric cattle in central Kenya, they were able to show that FMDV has a high seroprevalence in buffalo and targeted cattle populations. In addition, serotype SAT1 and SAT2 sequences from buffalo and serotype O and A sequences from sympatric cattle were recovered. These results further confirmed the findings of Wekesa et al. (Reference Wekesa, Sangula and Belsham2015), because amongt sympatric buffalo and cattle, no SAT1 or 2 sequences found in buffalo were found in cattle, which suggests that transmission of FMDV from buffalo to sympatric cattle in this region is rare. Similarly, there was no evidence that serotype O and A sequences found in cattle were transmitted to buffalo. Serotypes A and O have also been introduced with infected cattle into Ethiopia and other countries in the region. In Ethiopia, serology confirmed the presence of antibodies to serotypes A, SAT1 and SAT2 in clinically normal buffalo in Mago/Omo NP, whereas in neighbouring cattle, which have a seropositivity prevalence of between 5.6 per cent and 42.7 per cent with endemic distributions, serotypes O, A, SAT1 and SAT2 were documented (Sahle, Reference Sahle2004; Abdela, Reference Abdela2017). More recently (2021), Eurasian serotype O has been reported in cattle in Zimbabwe, Namibia and Mozambique.
FMD is rarely a fatal disease in cattle except for occasional calf mortalities, but it certainly does impact animal production, as dairy cows dry up and beef cattle lose condition. It is therefore not generally considered to be an important disease among pastoralists and subsistence farmers. However, due to FMD international control regulations (through the WOAH (World Organization for Animal Health, founded as the OIE (Office International of Epizootics)) directed by northern hemisphere countries, subsistence farmers living close to buffalo populations in Africa have been negatively impacted. Fortunately, in recent years there has been an increasing realization among world leaders that globalization is perpetuating the dominance of the developed world, and there is a genuine desire to address poverty issues in Africa (Ferguson et al., Reference Ferguson, Cleaveland and Haydon2013; Thomson et al., Reference Thomson, Penrith and Atkinson2013a, Reference Thomson, Penrith and Atkinson2013b; Chapter 12).
Buffalo-Associated Theileriosis
Theileria parva is a tick vector-borne protozoan infection of buffalo. It is transmitted by Rhipicephalus appendiculatus and R. zambesiensis, which are three-host ticks. It is naturally a silent infection in buffalo, but is highly pathogenic and causes an acute fatal disease in cattle, especially the European cattle breeds (Neitz et al., Reference Neitz, Canham and Kluge1955). It is generally a dead-end disease in these cattle, because they die before the lymphoid tissue-associated schizonts can produce erythrocyte-associated merozoites (small piroplasms), and are therefore unable to infect the vector ticks to maintain circulation of the infection. Small clustered outbreaks of buffalo-associated theileriosis still occur today in southern and East Africa where there is a buffalo/cattle interface. However, cattle-adapted strains have now also evolved, which cause the diseases known as East Coast fever (ECF) and Zimbabwe theileriosis (January disease). These diseases can circulate independently in cattle without any buffalo presence because these Theileria parva variants can complete their life cycle in cattle, produce small piroplasms, and are therefore able to infect the tick vectors (Irvin and Cunningham, Reference Irvin, Cunningham, Ristic and McIntyre1981). ECF occurs widely through the range of the same main vectors (R. appendiculatus and R. zambesiensis) in East and southern Africa north of the Zambezi River. In these regions, ECF, together with heartwater and trypanosomiasis, remain the greatest disease obstacle to agricultural development and prosperity. However, T. parva is absent in West Africa and most of Central Africa, including the whole Congo basin, because R. appendiculatus is absent. In Central Africa, the disease is only present in the far eastern side, above 500 m in the region of the Great Lakes: Burundi, Rwanda, Kivu in eastern DRC. This is a disease of great concern to most pastoralists in the affected countries because it has the potential to threaten food security. ECF was also introduced with cattle movement to regions south of the Zambezi River in 1901/02, but after resulting in the death of 1.25 million of the 4 million cattle present in the affected territories, was subsequently eradicated at great expense to governments and both farmers and pastoralists. From an agriculturalist/pastoralist perspective, this was a disaster, but for conservation and environmental objectives, these diseases have ensured that large tracts of land in Africa remain ecologically intact and viable, a vital element in the desired long-term recovery from anthropogenic impacts that are of such contemporary global concern. These diseases were eventually eradicated from southern Mozambique in 1917, from Rhodesia in 1954 and from South Africa in 1955 (Lawrence, Reference Lawrence, Norval, Perry and Young1992), but continue to circulate in cattle north of the Zambezi River.
There are other theilerias frequently found in buffalo blood samples, such as T. mutans (transmitted by Amblyomma spp.) and T. taurotragi (transmitted by R. appendiculatus). Both of these parasites generally cause benign infection in cattle. Buffalo-associated T. taurotragi also generally results in a mild infection in cattle, but occasionally causes a disease called turning sickness in young cattle, characterized by nervous signs, such as circling, head pressing, ataxia, paralysis and uni- or bilateral blindness (Lawrence and Williamson, Reference Lawrence, Williamson, Coetzer and Tustin2004).
Trypanosomiasis
Trypanosomiasis is a very important disease of cattle (known as nagana), camels (known as surra), horses, pigs and dogs in parts of sub-Saharan Africa, and certain Trypanosoma species may also affect humans (causing sleeping sickness disease). The various Trypanosoma spp. have different transmission modes, including biological transmission by tsetse flies, an insect genus endemic to Africa that had time to co-evolve with buffalo (T. brucei and T. congolense, T. vivax), and mechanical transmission by other biting flies of three main families, Tabanidae (genera Chryspos, Haematopota and Tabanus), Stomoxynae (genera Stomoxys and Haematobia) and Hippoboscidae (genera Melophagus and Hippobosca) (all previous plus T. evansi) (Itard, Reference Itard1981). With non-tsetse biting flies, trypanosomiasis expands well beyond the tsetse fly-infested areas.
Historically, the distribution of tsetse flies (known as tsetse belts) profoundly shaped and limited the distribution of livestock, and consequently severely hampered the development of the livestock industry. However, the situation has changed dramatically in the last decades under pressure from two main driving forces: (i) the drastic reduction of the tsetse distribution range, due notably to the destruction of their habitat such as forest galleries for agriculture (Gouteux et al., Reference Gouteux, Blanc and Pounekrozou1994; Cuisance, Reference Cuisance1996; Reid et al., Reference Reid, Russell and Kruska2000), tsetse eradication campaigns, the global climate change (Courtin et al., Reference Courtin, Rayaissé and Tamboura2010) and for savanna tsetse species such as Glossina morsitans, the disappearance of large game (Itard, Reference Itard1981); and (ii) the development and now widespread use of trypanocide drugs, both preventive and curative, which allow large transhumant cattle herds to graze in tsetse-infested areas during the dry season, including in Protected Areas, and leads to the degradation of the pastoral rangeland though excessive bushfire, overgrazing, tree felling, etc.
Preferred wildlife hosts of tsetse flies include African buffalo, wild porcines, spiral horned antelopes (Tragelaphs spp.), elephants, black rhinoceros and hippopotami, and these species are all capable of surviving well within the tsetse belts. They frequently develop significant infection rates with detectable parasitaemias, but without developing disease, and may therefore serve as natural maintenance hosts for the various Trypanosome spp. Interestingly, the respective historical distribution ranges of African buffalo and tsetse flies largely match (Olubayo, Reference Olubayo1991). Buffalo and tsetse flies have co-evolved in the same ecological system for millenaries to the mutual advantage of the three stakeholders, the parasite, its vector and its host, with the consequence of having made the buffalo both a maintenance host and one resistant to the parasite. The mechanisms of trypano-resistance of the African buffalo have been partially investigated (e.g. Olubayo, Reference Olubayo1991; Redruth et al., Reference Redruth, Grootenhuis and Olubayo1994; Wang et al., Reference Wang, Hamilton and Black2000; Guirnalda et al., Reference Guirnalda, Murphy, Nolan and Black2007).
Trypano-tolerant cattle breeds, all of them humpless Bos taurus (e.g. N’dama and Baoulé cattle), as well as trypano-tolerant goat and sheep breeds, have evolved in pastoral societies, mainly in West Africa, but also in Central and East Africa (Murray et al., Reference Murray, Morrison and Murray1979). They are, however, not trypano-resistant and may potentially serve as maintenance hosts.
An important conservation corollary is that many of the remaining and relatively pristine wildlife conservation areas in Africa owe their very existence to the presence of this disease, which made these areas unsuitable for agricultural expansion or human habitation (Chapter 12). However, as seen above, the situation is changing drastically with the wide use of trypanocide drugs. Pastoralists no longer hesitate to enter deep inside tsetse-infested Protected Areas with large cattle herds. To quote only one striking example from Cameroon, before trypanocid drugs no livestock entered Bouba Ndjidda NP and the seven surrounding Hunting Areas due to heavy tsetse infestation, while in 2015 the wildlife aerial census of these Protected Areas counted 526,233 (over half a million) head of cattle (Wildlife Conservation Society, Ministry of Forests and Wildlife and Ecole de Faune de Garoua, 2015), which was 21 times more than in 2008 (Omondi et al., Reference Omondi, Bitok and Tchamba2008), despite the continued presence of tsetse flies there today.
With the contemporary shrinkage of the distribution range of tsetse flies, their main remaining strongholds are now largely Protected Area complexes, which also are the residual strongholds of the African buffalo. In these restricted situations, the coevolution of the tryptic ‘buffalo/tsetse/trypanosome’ is maintained, including the resistance of buffalo to the disease, and buffalo remain there as a maintenance host and maybe even a bridge host. The question is raised whether these Protected Areas can now be regarded as maintenance sites, including for Human African Trypanosomiasis (HAT). HAT originating from wildlife has been explored to some extent, but only in a very limited number of wild species like bushbuck (Heisch et al., Reference Heisch, McMahon and Manson-Bahr1958). Much remains to be investigated with regards to the possible role of wildlife, including buffalo, in the maintenance of Trypanosoma brucei gambiense, which is responsible for HAT in West and Central Africa.
Peste des petits ruminants
The peste des petits ruminants (PPR) virus is closely related to the rinderpest virus (serologically indistinguishable with screening enzyme-linked immunosorbent assays, or ELISAs), from which it likely evolved (rinderpest of small ruminants) over recent centuries in West Africa. It spread to much of the rest of Africa and Asia, and this pandemic has gathered pace over the last two decades, entering East Africa and spreading south and across Asia, reaching the China seaboard in 2013. Serological surveys of wildlife, including mostly buffalo, during the rinderpest eradication campaign showed cross neutralization between rinderpest and PPR antibodies, and ELISA tests during epidemio-surveillance produced confusing results. Once differentiated, this confirmed widespread infection of wild artiodactyls, buffalo included, in Africa with the PPR virus where livestock were infected, and evidence suggests the small ruminants spilled the virus into adjacent wildlife populations (Kock, Reference Kock, Barret, Pastoret and Taylor2006; Mahapatra et al., Reference Mahapatra, Sayalel and Muniraju2015; Fernandez Aguilar et al., Reference Fernandes Aguilar, Mahapatra and Begovoeva2020). Buffalo were no exception and have shown the highest true prevalence for PPR virus infection among sampled species (Jones et al., Reference Jones, Mahapatra and Keyyu2021). No evidence of disease in African buffalo is reported, while only rare and, epidemiologically unconfirmed, reports of PPR disease have been made in sub-Saharan Africa in free-ranging antelope, notably gazelle in Sudan (Asil et al., Reference Asil, Ludlow and Ballal2019).
Malignant Catarrhal Fever
In Africa, the most important cause of malignant catarrhal fever (MCF) in cattle in the context of this chapter is wildebeest-associated MCF caused by Alcelaphine Herpesvirus 1. There is also a sheep-associated MCF affecting cattle caused by Ovine Herpesvirus 2. The important role played by wildebeest (Connachaetes spp.) in the maintenance and seasonal shedding of Alcelaphine Herpesvirus 1 has been elucidated (Plowright et al., Reference Plowright, Ferris and Scott1960; Plowright, Reference Plowright1967; Mushi et al. Reference Mushi, Karstad and Jesset1980). Free-ranging African buffalo in multi-species systems may be infected with this virus and sero-convert, but do not develop overt clinical signs of disease, or detected viral persistence, and thus appear totally unimportant in the maintenance or transmission of this important viral disease of cattle. West and Central Africa are outside the wildebeest distribution range and MCF infection has not been reported in buffalo there.
Rabies
Rabies is an ancient disease, and recognizable descriptions of it can be traced back to early Chinese, Egyptian, Greek and Roman records (Wilkinson, Reference Wilkinson, Campbell and Charlton1988). In sub-Saharan Africa, sylvatic rabies has been diagnosed in 33 carnivorous species and 23 herbivorous species, including African buffalo (Mitchell et al., Reference Mitchell, Steyl and Woodburn2021), with regional variation in dominant epidemiological role players (Swanepoel, Reference Swanepoel, Coetzer and Tustin2004). In spite of this, by far the largest number of rabies cases reported in the developing world occur in domestic dogs. In Africa, endemic rabies (caused by both viverid and canid biotypes) has been identified in certain communal burrow-dwelling wildlife species such as the yellow mongoose, and in bat-eared fox as well as various jackal species. African buffalo appear to be incidentally infected and do not appear to play any role in the maintenance of this infection.
Buffalo Involvement in Other Important Livestock Diseases
Tick-borne Diseases
Heartwater (Cowdriasis, Ehrlichia/Rickettsia ruminantium Infection)
Heartwater is a tick-transmitted rickettsial infection, and is one of the most important diseases of domesticated ruminants in sub-Saharan Africa. This disease causes high morbidity and significant mortality in cattle (especially Bos taurus types), as well as in sheep and goats, throughout the range of its biological vectors, which are present in most of sub-Saharan Africa with the exception of the extremely arid zones. The important biological vectors are Ixodid ticks of the genus Amblyomma, which are three-host ticks. Interestingly, in West and Central Africa, the historical distribution area of buffalo largely matches with the range of A. variegatum (annual rainfall over 500 mm). Some Amblyomma are specific to buffalo and suspected to be vectors, namely A. splendidum in forest buffalo (Syncerus caffer nanus), A. astrion in Central African savanna buffalo (S. c. aequinoctialis), A. cohaerens in East African buffalo and A. hebraeum in Southern African buffalo (Morel, Reference Morel1981).
Free-ranging African buffalo, together with giraffe, black wildebeest, blesbok and eland that occur within the distribution range of the Amblyomma ticks, frequently harbour this rickettsial organism without developing the disease. The infection in these species is generally subclinical due to the evolution of disease resistance over millennia and the development of an endemically stable host/pathogen relationship. Helmeted guineafowl, leopard tortoises and scrub hares, which are the preferred hosts of the larval and nymph stages of this vector, may also harbour this pathogen (Oberem and Bezuidenhout, Reference Oberem and Bezuidenhout1987). All of these silent carriers may potentially serve as sources of infection for the vector ticks, which theoretically could infect livestock in an open interface situation where livestock and wildlife share range and resources. However, it appears from recorded spatial and temporal patterns of disease outbreaks that the major source of infection for the vectors causing most livestock outbreaks are in fact the domestic livestock themselves, and this perpetuates the cycle of infection and disease.
Anaplasmosis
Both Anaplasma marginale subsp. centrale and A. marginale are regularly found in buffalo blood samples. Eygelaar et al. (Reference Eygelaar, Jori and Mokopaseto2015) report prevalence rates of 30 per cent for A. marginale subsp. centrale and 20 per cent for A. marginale in free-ranging buffalo in northern Botswana. These pathogens can be transmitted by several one-host and multi-host ticks of the genus Rhipicephalus; they can also be mechanically transmitted by biting insects, mainly by biting flies of the families Tabanidae and Stomoxynae, less so by mosquitoes (Morel, Reference Morel1981). A. marginale is pathogenic in cattle, causing severe anaemia and icterus, mainly in older dairy and beef cattle of the Bos taurus type. This disease is commonly known as ‘dry gall sickness’. In endemic areas, most calves are generally immune. A. marginale subsp. centrale infection is usually apathogenic in cattle, and is in fact used in a blood-based vaccine for cattle. It appears that buffalo have become incidentally infected by ticks that have fed on infected cattle and play a minor role, if any, in the maintenance of this disease. Buffalo do not develop any clinical signs of infection.
Babesiosis
The babesioses are tick-borne infections caused by intra-erythrocytic protozoal parasites of the genus Babesia. Four species are known to infect cattle in southern Africa, namely B. bovis, B. bigemina, B. occultans and an as yet unnamed species. B. bovis and B. bigemina are highly pathogenic and both cause redwater disease in cattle, but they have never been documented in African buffalo. Other pathogenic Babesia occur in sheep, goat, pigs and equids. Therefore, buffalo do not appear to play any role in the epidemiology of these two important cattle diseases. However, B. occultans, which causes benign infections in cattle, is also frequently found in buffalo, and a prevalence of 23 per cent has been reported in 120 buffalo blood samples collected in Northern Botswana (Eygelaar et al., Reference Eygelaar, Jori and Mokopaseto2015). The vector of B. occultans is Hyalomma marginatum rufipes, a two-host tick with a wide distribution range in southern Africa.
African Insect-Borne (Arbo-) Virus Diseases
Serological studies have shown that a whole host of wild artiodactyls, including African buffalo, are able to be infected with several of these insect-borne viruses, but the infections are naturally subclinical, indicating innate resistance or low pathogenicity in these wildlife species. These diseases include lumpy skin disease (LSD), caused by a capripox virus, bluetongue and epizootic haemorrhagic disease caused by orbiviruses, bovine ephemeral fever caused by a rhabdovirus, Rift Valley fever (RVF) caused by a phlebovirus and congenital arthrogryphosis/hydrancephaly caused by Akabane virus. In addition, some recent investigations have looked into the involvement of buffalo in certain Flavivirus and Bunyavirus infections.
Lumpy Skin Disease
Davies (Reference Davies1991) reported the detection of antibodies to LSD in buffalo in an endemic LSD area, but no clinical disease. In South Africa, sero-surveys of 440 free-ranging buffalo from KNP were all negative for serum-virus neutralizing antibody. In addition, experimental infection of sero-negative buffalo gave negative results (Howell and Coetzer, unpublished results). These animals did not even sero-convert. From these observations, it can be concluded that African buffalo are not susceptible to this virus and play no role in the epidemiology of this important disease of cattle.
Bluetongue
Serum-virus neutralizing antibodies have been detected in African buffalo, as well as many other wild sympatric ruminants (Davies and Walker, Reference Davies and Walker1974). However, clinical disease has not been reported, and the role of these wild ruminants in the epidemiology of this livestock disease remains speculative.
Ephemeral Fever
Also known as three-day stiff sickness, ephemeral fever occurs in most sub-Saharan countries in the form of epizootics in cattle. In between these epizootics, limited foci of disease may be encountered and there is evidence of sero-conversion in sentinel herds. The examination of a range of wild ruminant sera showed evidence of neutralizing antibody in 54 per cent of buffalo, 62 per cent of waterbuck (Kobus ellipsiprymnus), 9 per cent of wildebeest and 2.8 per cent of hartebeest (Alcelaphus buselaphus) (Davies et al., Reference Davies, Shaw and Ochieng1975). An interesting observation is that there were sero-conversions in waterbuck and buffalo in samples collected before the previous cattle epizootic of the disease. This would appear to indicate that the virus was circulating in wild ruminant populations during a period when no clinical disease was observed in cattle. Thus wild ruminants, including buffalo, may play a maintenance role during inter-epizootic periods.
Rift Valley Fever
Rift Valley fever is a zoonotic mosquito-borne virus disease of livestock and wild ruminants that has been identified as a risk for international spread. Typically, the disease occurs in geographically limited outbreaks associated with high rainfall events, and can cause massive losses of livestock. It is unclear how the RVF virus persists during interepidemic periods, but cryptic low-level cycling of the virus in livestock and/or wildlife populations may play a role. What is known is that the RVF virus can be efficiently maintained by certain floodwater breeding Aedine mosquitoes. In these mosquitoes, male/female sexual transmission as well as transovarial transmission of virus occurs. The eggs are laid on grasses, sedges and mud on the edge of rainwater pans, and these eggs are dormant and require a drying-out period followed by re-wetting to hatch. The time course for this drying out and re-wetting follows climatic cycles and may be weeks, months, years or even decades, and this is probably the main maintenance mechanism during the interepidemic periods (Linthicum et al., Reference Linthicum, Davies, Bailey and Kairo1984, Reference Linthicum, Davies, Kairo and Bailey1985).
In 1999, an abortion storm caused by the RVF virus occurred at a disease-free buffalo breeding project in KNP, followed by a second outbreak at another buffalo breeding project just south of the park. Serological surveys in free-ranging buffalo in KNP revealed generally low levels of sero-positivity that spiked during these outbreaks (Beechler et al., Reference Beechler, Bengis and Swanepoel2015). In addition, several other aborted buffalo foetuses were also positive for RVF (Mitchell et al., Reference Mitchell, Steyl and Woodburn2021). Sero-positivity also has been detected in a range of other wild ungulates in South Africa (Swanepoel, Reference Swanepoel1976) and Zimbabwe (Caron et al., Reference Caron, Miguel and Gomo2013). In Kenya, a sero-prevalence of around 15 per cent of RVF virus neutralizing antibodies has been detected in buffalo (Evans et al., Reference Evans, Gakuya and Paweska2008; Britch et al., Reference Britch, Binepal and Ruder2013). Many buffalo live in endemic RVF areas, and this could explain the seroconversion to this mosquito-borne disease. It is notable that other wildlife such as gazelles living outside these endemic regions can suffer clinical disease during periods of epizootic expansion, although this has not been observed in sympatric buffalo. It also has been observed that significant mortality from RVF occurred when naive scimitar-horned oryx (Oryx damma) were translocated into the RVF endemic area of Chad from the UAE, whereas the long-term resident oryx appeared resistant (Chardonnet, personal communication).
In 2010, during a major outbreak of RVF in the Orange Free State and Northern Cape Provinces in South Africa, apart from heavy livestock losses, RVF-associated abortions and mortality were recorded in ranched buffalo, eland (Taurotragus oryx), sable antelope, waterbuck, springbok (Antidorcas marsupialis), blesbok (Damaliscus dorcas phillipsi) and bontebok (D. d. dorcas), as well as exotic fallow deer, llamas and alpacas. Whether buffalo or any of these other species play a role in low-level maintenance cycling of the RVF virus during the interepizootic period remains speculative.
Mycoplasma
Contagious bovine pleuropneumonia (CBPP), caused by Mycoplasma mycoides subsp. mycoides, is one the three great historic cattle plagues of the world (OIE, 2022a), and Contagious caprine pleuropneumonia (CCPP), caused by M. capricolum subsp. capripneumoniae, is one of the most severe diseases of goats (OIE, 2022b). Moreover, these two diseases have gained renewed attention since the eradication of rinderpest and the PPR eradication programme. The African buffalo is not susceptible to CBPP and does not play any role in its transmission. In contrast with CBPP, which affects cattle only, CCPP also affects a number of wild ungulates, including some African antelopes; however, it is not known to affect the African buffalo. It is difficult to evaluate the prevalence of these mycoplasma diseases in wildlife due to sampling and transportation constraints. However, the use of specific serological tests and PCR may improve information in wildlife.
Emerging Infectious Diseases
So-called emerging animal infectious diseases include recently detected diseases (often in new geographies), variants of known diseases, diseases that have recently crossed the ‘species barrier’ and finally, truly novel diseases. With regard to African buffalo, the following emerging infectious diseases have been reported.
Bovine Papillomatosis
Infection with bovine papilloma virus types 1 or 2, causing cutaneous lesions in giraffe, Cape mountain zebra (Equus zebra zebra), sable antelope and African buffalo, have been described and confirmed by histopathology and immunohistochemistry. These cutaneous lesions varied from single or multiple wart-like growths to massive sarcoids (Williams et al., Reference Williams, van Dyk and Nel2011; Figure 9.4). This is a good example of a cattle disease that has crossed the species barrier.

Figure 9.4 Papillomatosis on an African buffalo.
Diseases Caused by Akabane and Related Simbu-Group Viruses
In KNP, several buffalo calves with arthrogryposis and hydranencephaly were born at a buffalo breeding facility. These congenital defects are frequently a result of in utero infection of the foetus with Akabane virus during certain critical stages of development. There is no reason to suspect that these congenital deformities do not also occur in free-ranging buffalo, but are rarely seen because most new-born animals with congenital defects are taken out by predators. In a sero-survey for Akabane virus infection in African wildlife, neutralizing antibody was detected in 222/979 buffalo (22.7 per cent; Al-Busaidy et al., Reference Al-Busaidy, Hamblin and Taylor1987).
In the past decade, mortalities associated with neurological clinical signs have been reported in several free-ranging wildlife species, including a variety of antelope, warthogs, white rhinoceros and African buffalo. Histopathology demonstrated a viral encephalitis characterized by glial apoptosis, neuronal degeneration and/or cerebral oedema (Mitchell et al., Reference Mitchell, Steyl and Woodburn2021). A Shuni virus of the Simbu group has been implicated.
Diseases Caused by Flaviviruses
Bovine viral diarrhoea virus (BVDV) and antibody have been detected in a whole range of free-ranging ruminants (Hamblin and Hedger, Reference Hamblin and Hedger1979), and in Africa, some buffalo and wildebeest populations suffer high infection rates (Hyera et al., Reference Hyera, Liess, Anderson and Hirji1992). In a recent report (Mitchell et al., Reference Mitchell, Steyl and Woodburn2021), two aborted buffalo foetuses and one neonatal mortality were confirmed to be caused by BVDV, using immunohistochemistry.
Macroparasitic Infections in African Buffalo
When reading this paragraph, what needs to be appreciated is that all free-ranging wildlife are ‘biological packages’ that are infected subclinically by a variety of endo- and ectoparasites. African buffalo are no exception, and host numerous different nematodes, cestode and trematode worms and several external parasites (Boomker et al., Reference Boomker, Horak, Penzhorn, Keet and Penzhorn1996). What is important is that most of these parasites do not appear to deleteriously affect the health of free-ranging wildlife in any way. In free-ranging multi-species wildlife systems, certain mammalian species may be susceptible to certain parasitic infections, and the uptake of larvae or ova by non-patent species functions as a natural balancing mechanism that reduces infection burden and helps to reduce parasite loads resulting in sustainable host/parasite relationships. In addition, in the co-infection context in which most wild species live, multiple minor parasitic infections may result in parasitic competition or in immune stimulation, both of which attenuate parasitic pathogenesis and minimize the effect on the host health (Chapter 11).
It is beyond the scope of this chapter to attempt to tabulate and describe all of the macro-parasites that have been detected in African buffalo, and we will limit our discussion to include mainly those parasitic infections of buffalo that are overtly visible in the live animal or in the dead animal carcass.
Ixodid Ticks
Most free-ranging wildlife are parasitized to a greater or lesser extent by one or more of the life-cycle stages of ixodid ticks. Certain species, such as African buffalo, giraffe and black and white rhinos, are preferred hosts for the adult stage of several of these ixodid ticks, which is the reproductive stage of these ticks. Adult ticks of the important genera Amblyomma, Rhipicephalus and Hyalomma are frequently found on these host species. In a study conducted in Ethiopia’s Mago/Omo National Park (Shiferaw and Kock, Reference Shiferaw and Kock2002), seven species of ticks, namely Amblyomma cohaerence, A. lepidum, A. variegatum, A. nuttali, A. gemma, Rhipicephalus pravus, R. pulchaellus and R. evertsi, were collected from buffalo. In southern Africa, A. hebraeum, R. appendiculatus and R. zambeziensis are the most common buffalo ticks. In Central African Republic, A. variegatum, A. astrion, R. longus, R. muhsamae, R. cliffordi, R. lunatus, Hyalomma nitidus and H. rufipes have been described on 100 per cent of 85 examined buffalo (Thal, Reference Thal1971).
Parafilaria bassoni Infection
Parafilaria bassoni is a spirurid nematode that infects the skin and subcutaneous tissues of buffalo, causing bleeding skin nodules (Figure 9.5). These bleeding nodules are associated with gravid female worms ovipositing embryonated eggs. These lesions occur mainly on the dorsum and lateral sides of the buffalo. Complications of these lesions develop in a low percentage of buffalo due to secondary bacterial infections forming subcutaneous abscesses, or as a consequence of a Type 1 hypersensitivity reaction that may result in vascular occlusion, and skin infarction with the development of cutaneous ulcers, which often become enlarged by oxpecker worry. A sero-survey using a Parafilaria ELISA in the Greater KNP Complex demonstrated that this parasite occurs in buffalo populations throughout this complex at a seroprevalence rate of approximately 34 per cent (Keet et al., Reference Keet, Boomker and Kriek1997).
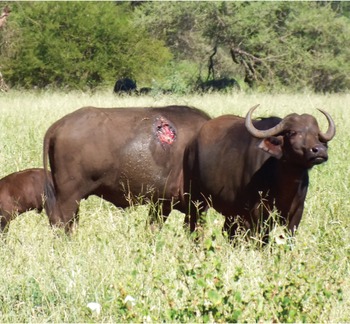
Figure 9.5 Parafilaria skin ulceration on an African buffalo.
Psoroptic Mange
Psoroptic mange is caused by a large sarcoptiform mite, Psoroptes pienaari, commonly found on buffalo. This is a large mite that can be seen with the naked eye. This mite is most commonly found on the head, neck, shoulders and rump of buffalo, and causes a pruritic scaley alopecia (hair loss), frequently giving the skin a thickened, hairless, greyish appearance.
Demodectic Mange
Demodectic mange is caused by the parasitic mite Demodex caffer, which inhabits the hair follicles in the skin of buffalo, and when present in large numbers may cause nodular parasitic and sebaceous cysts (Figure 9.6). These cutaneous skin nodules are most commonly seen in the younger age classes, and are generally limited to the head, neck and shoulder regions, but in severe cases, may be present over the entire animal (Dräger and Paine, Reference Dräger and Paine1980). If one of these nodules is incised and lateral pressure applied, a thick, creamy white material exudes, which consists of sebaceous cells and masses of Demodex mites. In a study in KNP, approximately 50 per cent of 203 buffalo examined had clinical signs varying from few to multiple skin nodules (Wolhuter et al., Reference Wolhuter, Bengis, Reilly and Cross2009).

Figure 9.6 Nodular demodex on a captured African buffalo.
Parasites Commonly Seen in Buffalo Carcasses
Buffalo serve as intermediate hosts for a number of tapeworms and enteric protozoa of sympatric predators, which are the definitive hosts. Cysticercosis usually seen in the muscles of the forelimbs, diaphragm and heart muscle of buffalo are the intermediate stages of the tapeworms of lions, leopards and hyaenas, such as Taenia regis, T. gonyamai and T. crocutae. In some regions where there is a close interface between buffalo and humans, cysticerci of the human tapeworm, T. saginata, have also been found in buffalo.
Two trematodes of common pathological importance for livestock are frequent in buffalo with no clinical signs, namely the giant fluke (Fasciola gigantea) and the small fluke (Dicrocoelium hospes), which are found in buffalo livers, often together and sometimes in a massive infestation. In Central African Republic, 12 of 33 inspected buffalo (36.3 per cent) were affected by both flukes with no apparent clinical signs (Thal, Reference Thal1971). Other trematodes of the genus Schistosoma also are frequently found in the mesenteric blood vessels of buffalo, again causing no clinical signs.
Hydatidosis and Coenurus Cerebralis
Hydatid and coenural cysts are parasitic cysts occasionally observed in buffalo carcasses. These cysts are the intermediate stage of the wild predator and domestic dog tapeworms, Echinococcus granulosis and T. multiceps. Hydatid cysts are most commonly found in the liver, lung and occasionally brain of buffalo, and may be large and multiple. Coenural cysts are much less common, and may be found in the brain or subcutaneous tissues.
Sarcosporidiosis
Sarcosporidia are protozoal parasites that have a two-host life cycle. The sexual reproductive cycle takes place in the small intestine of predators, and sporocysts are passed in their faeces and contaminate the environment. These sporocysts are then ingested by grazing herbivores and undergo asexual replication to form merozoites, which in turn form the sarcocysts in the muscle. Sarcocysts are cysts filled with thousands of bradyzoites, and it is these that will infect a predator when the muscle tissue is eaten, and thus the life cycle is completed. In the African buffalo, large macrocysts caused by Sarcocystis fusiformis (preliminary identification) are most commonly seen in the tongues and pharyngeal muscles of older buffalo, but can occur elsewhere (Quandt et al., Reference Quandt, Bengis and Stolte1997). Most buffalo appear to be infected, and these macrocysts are a common finding at meat inspection and may result in partial condemnation of the carcass.
Pentastome Larvae
The larval stages of a pentastome parasite of the paranasal sinuses of large carnivores, Linguatula serrata, are frequently found in the mesenteric lymph nodes, liver and cardiac chambers of African buffalo at meat inspection.
Conclusions and Summary
We hoped to provide a comprehensive view of infections and macro-parasites of African buffalo, although significant gaps remain in our knowledge. All in all, the African buffalo is a resilient and hardy species that is well adapted to harsh African conditions and pathogen challenges. Much of its reputation as a disease villain is not warranted, and its qualities as a resource for nature, ecosystems and humanity speak for themselves.
Introduction
African buffalo (Syncerus caffer) and domestic cattle (Bos taurus, B. indicus) coexist in large tracks of Africa. Both are large bovid species (but see Chapter 2) that are principally grazers with similar body sizes, and therefore rely on and compete for the same natural resources. Savannas are an important biome in Africa that have been maintained for the last millennia by the interaction of wild herbivores, livestock and their herders. Human-induced fire and livestock dung-related nutrient cycling play an important role in the enrichment and heterogeneity of these habitats (Marshall et al., Reference Marshall, Reid and Goldstein2018). Savannas offer important grazing that is more or less degraded or constrained by the footprint of human activities, including agricultural expansion and the scarcity of surface water, especially during the dry season (Valls-Fox et al., Reference Valls-Fox, Chamaillé-Jammes and de Garine-Wichatitsky2018). Today, most savanna African buffalo populations live in protected areas (Chapter 4), often with no physical separation to prevent interactions with livestock living on the periphery of, and more and more frequently within, these protected areas. Savanna buffalo populations outside protected areas live in areas where they can also encounter livestock (e.g. Garissa district, Kenya). Interactions between buffalo and cattle have increased significantly during the second half of the twentieth century due to the wider use of anti-trypanosomiasis drugs and the reduction of the range of trypanosomiasis vectors, Glossina sp. This provided an opportunity for herders to penetrate into grazing areas where cattle previously would have simply died, including in protected areas of West, Central and Eastern Africa. As livestock populations in these regions grew, so did demand for grazing resources, increasing competition with crop producers and placing livestock in closer proximity to buffalo populations relatively isolated from them until recently (e.g. Cuisance, Reference Cuisance1996). This phenomenon is not limited to savannas, as it can also be observed in rainforests in which forest buffalo (S. c. nanus) are increasingly interacting with intruding cattle.
Buffalo/cattle interactions are a source of conflict not only because both species compete for resources, but also due to the risk of disease transmission in both directions (Miguel et al., Reference Miguel, Grosbois and Fritz2017). These interactions can contribute to the disease burden of small-scale livestock production systems as buffalo can maintain or spread some diseases detrimental to the health of cattle (e.g. tick-borne diseases, bovine tuberculosis; Caron et al. Reference Caron, Miguel and Gomo2013; Chapter 9). Commercial livestock production, especially that intended for international trade, is very sensitive to some diseases that cannot be eradicated in buffalo, and therefore important trade regulations are imposed on producers depending on their exposition to buffalo/cattle interactions (e.g. foot and mouth disease, FMD; Scoones et al., Reference Scoones, Bishi and Mapitse2010; Thomson et al., Reference Thomson, Penrith and Atkinson2013; Chapter 12). Some of the diseases mentioned above are zoonoses, most of them hardly studied in African contexts and therefore with an (often unknown) impact on public health (e.g. Rift valley fever, brucellosis; Gadaga et al., Reference Gadaga, Etter and Mukamuri2016). Finally, the interactions work both ways, and cattle can transmit diseases that can threaten the survival of wildlife such as rinderpest, a cattle disease imported during European colonization that decimated wildlife populations in Africa (van Onselen, Reference van Onselen1972; Chapter 12). Buffalo/cattle interactions are therefore an important aspect of the management of African savannas and forests with socioeconomic, environmental and political implications. For example, the success of Transfrontier Conservation Areas in southern Africa connecting parks across borders and promoting wildlife mobility can be weakened by sanitary regulations aiming to protect cattle production from transboundary animal diseases (Ferguson et al., Reference Ferguson, Cleaveland and Haydon2013).
In this chapter, we will review the knowledge on the characterization of the buffalo/cattle interaction, the related ecology of pathogen transmission, and how this transmission can be modelled to improve the management and control of diseases. The geographical distribution of studies on buffalo/cattle interactions and the associated disease ecology is uneven, with almost none undertaken in rainforest habitats and most focused on savanna habitats in eastern and mainly southern Africa.
Characterizing the Buffalo/Cattle Interface
Wildlife/livestock interactions occur in wildlife/livestock interfaces that exist worldwide and represent a matter of concern for various reasons, including predation by wildlife, competition for resources, biodiversity conservation, cross-breeding and crop-raiding (Osofsky and Cleaveland, Reference Osofsky and Cleaveland2005). However, the risk of disease transmission at these interfaces has probably been the most burning issue in modern times (Kock, Reference Kock, Ososfsky, Cleaveland and Karesh2005). Frameworks to define and characterize these interfaces have also been proposed recently, including the definition of the interface that is used in this chapter: ‘the physical space in which wild and domestic species, as well as humans, overlap in range and potentially interact’ (Caron et al., Reference Caron, Barasona, Miguel, Vicente, Vercauteren and Gortazar2021). They principally focus on defining the geographic (e.g. spatial), physical (hard–soft edge) and dynamics (e.g. seasonality, small-scale and interannual dynamics) properties of the interface to understand if, where and when wild and domestic species interact.
For both buffalo and cattle, access to scarce water and grazing resources in the savanna ecosystem, including agricultural fields (which attract buffalo), is the main driver of buffalo and cattle movements across their respective land-use boundaries (i.e. protected areas and communal land). In addition, rainfall, natural and human-induced fires, as well as human activities and infrastructure are key factors influencing the distribution of buffalo and cattle in space and time (Higgins et al., Reference Higgins, Bond and February2007; Cornélis et al., Reference Cornélis, Benhamou and Janeau2011; Naidoo et al. Reference Naidoo, Du Preez and Stuart-Hill2012; Ogutu et al., Reference Ogutu, Owen-Smith and Piepho2012). These movements determine a spatial use that may or may not trigger contact between buffalo and cattle and create the buffalo/cattle interface.
In Africa, buffalo/cattle interfaces are found mainly in savanna ecosystems. Forest buffalo (S. caffer nanus) seldom interact with cattle, given their exclusive dwelling in forest habitats in which cattle husbandry seldom exists. However, recent changes in pastoral practices in Central Africa (e.g. south-west of the Central African Republic) have pushed cattle closer to forest buffalo habitat, especially during the dry season (Chardonnet, personal commmunication). In savannas, the buffalo/cattle interface can exist under the form of a ‘hard edge’, a type of interface found mainly in southern Africa and especially South Africa (e.g. the fence surrounding Kruger National Park on the South African side), but not exclusively (e.g. also in Botswana, Namibia; Figure 10.1). The remaining majority of the interfaces found in West, Central, Eastern and Southern Africa should be classified as ‘symmetric soft interfaces’ where both species can cross the edge and exploit resources a few kilometres maximum from the edge (Caron et al., Reference Caron, Barasona, Miguel, Vicente, Vercauteren and Gortazar2021; see e.g. Figure 10.2). In practice, few ‘diffuse interfaces’, where both cattle and buffalo permanently coexist on the same production unit, currently exist in Africa. These may occur locally within extensive mixed ranches associating cattle and buffalo for diversified ecotourism, hunting and meat productions. However, veterinary regulations applicable in most African countries prevent such associations to protect livestock from buffalo-borne disease transmission that would put a high burden on meat production both from a production and regulatory perspective. Early attempts in southern Africa to produce ‘disease-free’ buffalo herds, which could be associated with cattle herds on the same ranges, proved technically and financially difficult to maintain in the long run. From a spatial perspective, other types of interfaces such as ‘asymmetrical interfaces’ can exist but are rare, despite their potential to promote buffalo/cattle coexistence systems. The asymmetry can, however, emerge from ‘symmetric soft interfaces’ and produce some opportunities for coexistence between buffalo and cattle. For example, where cattle are penned at night to protect them from natural predation or theft, buffalo can use this nocturnal temporal window to use space previously used by cattle (Miguel et al., Reference Miguel, Grosbois and Fritz2017).

Figure 10.1 (a) Theoretical conceptual model of a wildlife/livestock (W/L) interface including wild buffalo (W) and domestic cattle (D) populations, human actors (H) as well as key landscape features including land-use boundaries (dark line separating a hypothetical protected area and its periphery) and key resources (pasture and surface water for example, represented by icons) that will help define hypotheses about the W/L interface (horizontal bidirectional arrow on top); the human component is only represented in panel (a) but it is assumed that the human driver is one of the most important to define W/L interfaces, defining cattle production practices, buffalo management and resource distribution. (b) Hard-edge interface: a fence or a natural impassable barrier (e.g. non-crossable river) limits the movements of buffalo and cattle: this is a hard edge; this type of interface is theoretical for many national park boundaries as animal movement-proof edges are rare. (c) Asymmetric semi-hard interface: only one of the two species (i.e. buffalo here) can cross the edge to use natural resources; the interface is limited to a small band in the cattle side; the reverse is of course also possible. (d) Symmetric soft interface: both species can cross the edge and exploit resources across the edge; this type of interface exists for many unfenced protected areas. (e) Diffuse interface: there is no edge and the home range of buffalo and cattle overlap extensively. In (c)–(e), the temporal dimension of the interface is crucial to understanding the dynamics of the interfaces.

(a) The Dete/Sikumi Forest interface in Zimbabwe without any fence: a mainly asymmetric interface during the rainy season when cattle enter the protected Sikumi Forest.

(b) The Malipati/Gonarezhou national park interface in Zimbabwe separated by the Mwenezi River that dries part of the year: asymmetric interface with buffalo entering the communal land most of the year but with some cattle incursions into the protected area during the cold-dry (and hot-dry) seasons.

(c) The Pesvi/Kruger national park in Zimbabwe/South Africa separated by the (large) Limpopo River that dries part of the year: an asymmetric interface with buffalo entering the communal land most of the year but with seasonal variations.
Figure 10.2 Three asymmetric and seasonal interfaces in southern Africa characterized by GPS localizations of cattle and buffalo in protected areas or communal land used for extensive subsistence livestock farming.
Characterizing wildlife/livestock interfaces has been the focus of recent research, supported by the development of technologies such as telemetry and remote sensing technologies (e.g. Richomme et al., Reference Richomme, Gauthier and Fromont2006; Pruvot et al., Reference Pruvot, Seidel and Boyce2014; Woodroffe et al., Reference Woodroffe, Donnelly and Ham2016; Campbell et al., Reference Campbell, Byrne and Menzies2019; Triguero-Ocana et al., Reference Triguero-Ocana, Laguna and Jimenez-Ruiz2021). To characterize interfaces, multidisciplinary approaches are often required. They can range from behavioural studies of wild and domestic species (e.g. telemetry or capture–marking–recapture techniques) to emerging non-invasive molecular techniques to assess the presence or absence of specific species (e.g. faecal or environmental sampling), as well as sociological studies to understand people’s perceptions, knowledge and practices regarding the state and management of the interface. Focusing on the characterization of buffalo/cattle interfaces, satellite remote sensing (SRS) offers an array of methodologies to monitor, characterize and quantify how natural resources impact buffalo and cattle movements in their respective environments (Rumiano et al., Reference Rumiano, Wielgus and Miguel2020). Optical and radar SRS imagery can be used efficiently to discriminate surface water and land covers at a landscape scale due to a wide range of sensors, with various spatial and temporal resolutions available (Corbane et al., Reference Corbane, Lang and Pipkins2015; Bioresita et al., Reference Bioresita, Puissant, Stumpf and Malet2018; Huang et al., Reference Huang, Chen, Zhang and Wu2018). The effects of fire on vegetation can spatially and temporally be detected using vegetation spectral signature as their intrinsic characteristics change over time (Meng and Zhao, Reference Meng, Zhao, Petropoulos and Islam2017). Whereas precipitation can be measured with advanced infrared (IR), passive microwave (MW) and radar sensors provide a complementary alternative to in situ records (Camberlin et al., Reference Camberlin, Barraud and Bigot2019). These SRS techniques are available to characterize interfaces across the range of buffalo and cattle in Africa at the spatial and temporal scale deemed most relevant to the issue at hand. Combining these SRS methodological approaches with telemetry studies on both species and the pastoralist and agro-pastoralist practices can provide a good understanding of buffalo/cattle interfaces.
Most of this research on wildlife/livestock interfaces has been done in the field of ecology (e.g. Hibert et al., Reference Hibert, Calenge and Fritz2010) and especially in the emergent field of disease ecology. The study of the ecology of pathogen and disease transmission at the wildlife/livestock interface seeks to: (1) understand the patterns of contact between wild and domestic species, especially the intensity and frequency of these contacts as well as their driving factors; (2) assess the proportion of these contacts that could trigger an ‘infectious contact’ defined as the interspecies transmission of a pathogen; and (3) model the host and pathogen population dynamics in this context and assess the efficiency of potential management options to mitigate or control diseases (de Garine-Wichatitksy et al., Reference de Garine-Wichatitksy, Miguel, Cornélis, Vicente, Vercauteren and Gortazar2021).
Measuring Infectious Contact at the Buffalo/Contact Interface
Measuring Contacts between Two Species
Determining the relative location of two individuals to each other (e.g. individual cattle and buffalo) is the first step to be able to estimate if there is a risk of interspecies pathogen transmission. This risk will be defined by the evaluation of potential infectious contacts between two individuals. As the observation of infectious contact per se is almost impossible (i.e. pathogens are invisible to the naked eye), interspecies contacts are used as a proxy. For a given pathogen, a direct mode of transmission requires close contact between an infected and a healthy individual, that is both hosts are at the same place and at the same time (Bengis et al., Reference Bengis, Kock and Fisher2002; Altizer et al., Reference Altizer, Nunn and Thrall2003). Indirect transmission can occur when a pathogen is excreted by the infected individuals in the environment at a specific location (e.g. directly on the ground or water) and subsequently infects a susceptible host using the same location after the infected host. Until recently, direct observation was the only way to determine the position of wild individuals, a time-consuming technique difficult to implement on two species. The advent of satellite telemetry using a global positioning system (GPS) has transformed the possibility to assess the temporal and spatial positions of animals in a given area with high precision and temporal accuracy (Cagnacci and Urbano, Reference Cagnacci and Urbano2008). This breakthrough in technology can generate a lot of data: a GPS collar collecting one GPS point every hour for two years will produce 17,520 locations of the individual in addition to its speed, the position of its head and the temperature among numerous data that can now be collected with captors integrated into the GPS collar. This technology has thus enabled new insights into the ecology of animal movements (e.g. patterns of biodiversity, ecological characteristics of individual species and ecosystem function; Kays et al., Reference Kays, Crofoot, Jetz and Wikelski2015; Eikelboom et al., Reference Eikelboom, Spruyt and Prins2021). Data describing the movements made by individual animals during their entire lifetime, and species-wide sampling from multiple populations, are now becoming available and offer new opportunities to measure and estimate contacts (Flack et al., Reference Flack, Fiedler and Blas2016). Wielgus et al. (Reference Wielgus, Cornélis and de Garine-Wichatitsky2020) used GPS telemetry to describe fission–fusion dynamics of buffalo in various groups at several sites. This example shows how GPS telemetry can define and improve species-inherent ecological behaviours that can potentially be used, by extension, to characterize intra- and interspecies contacts. Proximity loggers are another recently developed tool. While they only provide a measure of direct contacts between individuals (i.e. they detect and log events when tagged individuals are located within a predefined distance threshold; Böhm et al., Reference Böhm, Hutchings and White2009; Drewe et al., Reference Drewe, O’connor and Weber2013), they cost considerably less than GPS collars. This allows a larger number of individuals of a given wildlife or livestock population to be equipped, depending on the difficulties and costs associated with the capture/fitting of the collars.
Both technologies allow researchers to determine when, and for how long, two animals have been in proximity and, therefore, describe the contact patterns relevant for a directly or indirectly (only for GPS) transmitted pathogen. However, few studies on large herbivores occupying African savanna environments using these technologies have been conducted so far (Owen-Smith et al., Reference Owen-Smith, Hopcraft and Morrison2020). These new technologies have several constraints that can potentially limit their use. The most apparent is the cost of recording units (until recently between €1500 and €2500 per buffalo unit) to be fitted to individual animals (until recently between €1500 and €2500 per buffalo unit) (Cooke et al., Reference Cooke, Hinch and Wikelski2004) and the cost of the capture and then recapture to remove the collars (€1000–1500 per head). These devices are also not robust enough to study adult male buffalo and can be damaged by cattle during, for example, dipping for tick-borne disease control (Caron, personal communication). Moreover, GPS telemetry can affect animal behaviour, survival and well-being in some instances, and its system function is influenced by environmental variables (e.g. climatic factors, habitat types, terrain roughness) and animal behaviour (e.g. movement, orientation of the collar) (Tomkiewicz et al., Reference Tomkiewicz, Fuller, Kie and Bates2010). As a result, spatial inaccuracy of the acquired locations, and missing data in the form of failed location attempts, can potentially impact derived GPS telemetry data and lead to mistaken inferences on animal spatial behaviour, especially those involving movement paths and habitat selection (Frair et al., Reference Frair, Fieberg and Hebblewhite2010). Finally, movement is a continuous process that can only be tracked by sampling, usually at constant time intervals. This sampling is constrained by the limits of the technology used (battery life), which forces a trade-off between the sampling frequency of the displacement and the duration of the tracking. This trade-off is especially important when working on contacts between two individuals as we can assume that most of these contacts occur between sampling points. However, telemetry technology is developing rapidly and future systems may overcome some of these constraints.
Contact Estimation at the Buffalo/Livestock Interface
Few studies have investigated wildlife/livestock contacts for epidemiological or other purposes. Some of the main models studied so far are: the interface between the European badger (Meles meles) and cattle in the UK in relation to bovine tuberculosis (e.g. Woodroffe et al., Reference Woodroffe, Donnelly and Ham2016; Campbell et al., Reference Campbell, Byrne and Menzies2019); the interface between wild boar (Sus scrofa) and cattle in relation to the same disease in Spain (e.g. Barasona et al., Reference Barasona, Latham and Acevedo2014; Triguero-Ocana et al., Reference Triguero-Ocana, Barasona and Carro2019); the interface between white-tailed deer (Odocoileus virginianus) and cattle in the United States in relation to bovine tuberculosis (Ribeiro-Lima et al., Reference Ribeiro-Lima, Carstensen and Cornicelli2017); the interface between elk (Cervus canadensis nelson) and cattle in relation to brucellosis in the US (Proffitt et al., Reference Proffitt, Gude and Hamlin2011); and the buffalo/cattle interface in relation to FMD and bovine tuberculosis (e.g. Miguel et al. Reference Miguel, Grosbois and Caron2013, Reference Miguel, Grosbois and Fritz2017; Valls-Fox et al., Reference Valls-Fox, Chamaillé-Jammes and de Garine-Wichatitsky2018).
By combining telemetric and epidemiological approaches to sympatric cattle and buffalo, recent studies have provided good evidence that the contact rate with buffalo significantly influences FMD dynamics in cattle populations living at the periphery of conservation areas in Zimbabwe (Miguel et al., Reference Miguel, Grosbois and Caron2013, Reference Miguel, Grosbois and Fritz2017). In the latter study, 36 GPS collars were deployed on African buffalo and cattle to assess proximity patterns at the symmetric soft interface of three protected areas in Zimbabwe, namely Hwange and Gonarezhou in Zimbabwe and Kruger in South Africa. GPS acquired one location per hour and data collection ran between 14 and 17 months between 2010 and 2011. One head of cattle was equipped per herd (herd size averaged 12) on the assumption that the movement of one of the lead cows would significantly represent the daily movement of the herd. At night, cattle herds were penned in ‘kraals’ (a case of partial asymmetrical interface between cattle and buffalo at night; Figure 10.1) to protect them from predation and theft. Adult female buffalo were equipped and their movements were assumed to represent mixed herd movements (Chapter 6). To assess interspecies contacts relevant for FMD, direct and indirect contacts were calculated based on the buffalo–cattle dyad being: (1) at the same place together (i.e. direct contact); the 300 m radius accounts for GPS precision and herd size; or (2) one or the other being in a 300 m radius from a location of the other up to 15 days later; this spatial–temporal window was decided based on the potential survival of the FMD virus in the environment.
Contacts between buffalo and cattle varied between sites and seasons and individual cattle. Of importance, almost no direct contact was recorded during the entire study. The locations of indirect contacts were both inside the national parks and in the communal land and varied greatly between sites, with most of the spatial overlap occurring in the Kruger–Pesvi interface area (Pesvi is a small village across the Limpopo River in Zimbabwe, along the northern section of Kruger National Park; Figures 10.3 and 10.4). Contacts increased from the rainy season towards the late dry season.

Figure 10.3 Percentage of cattle/buffalo contacts relative to sites and land-use (inside national park – NP – or inside the Communal Land – CL): during the study by Miguel et al. (Reference Miguel, Grosbois and Caron2013).

Figure 10.4 Maps of cattle and buffalo home ranges (red-brown and red-yellow, respectively) and contacts at three national parks (NP) borders in southern Africa (KAZA-TFCA: Hwange–Dete and GL-TFCA: Gonarezhou–Malipati and Kruger–Pesvi). The locations of contact events between cattle and buffalo are represented by pink stars (i.e. cattle position recorded within 300 m of a buffalo position less than 15 days after the buffalo position has been recorded).
Because buffalo and livestock use similar resources, particularly water and grazing areas during the dry season, they use similar habitats, which explains the contact patterns observed. Quantitative observations of the density of vegetation on each side of the boundaries (National Park/Communal Land) were obtained, for the three interfaces, using satellite images and the calculation of NDVI (Normalized Difference Vegetation Index). Although NDVI does not allow grassland to be distinguished from shrubland and forest areas, this index can be used to measure the plant phenology and by extention, the distribution of available vegetation in communal lands and adjacent protected areas. High variability in terms of habitat use was observed across sites with NDVI structuring the buffalo habitat use. When NDVI was higher outside the protected areas (Kruger–Pesvi interface), buffalo exited from the Kruger NP boundaries to range inside the communal land areas (Figure 10.5).

Figure 10.5 NDVI estimations (lines) in communal lands and protected areas of the three sites studied in relation to the distance from the interface (dark vertical line). The cattle and buffalo pictogram illustrates the localizations of the contacts between the two species and the line below these pictograms represents the 95 per cent range of these contacts.
Surface water distribution among study sites varied significantly. Two river systems for Pesvi–Kruger (Limpopo River) and Malipati–Gonarezhou (Nuanetsi River) flow part of the year and only offer a few stagnant pools of water during the dry season. In Dete–Hwange, scattered water pans provide water across the year, with their number decreasing as the dry season proceeds. This contrast in water distribution patterns could explain the difference in contact rates between the three study areas. For example, in the Hwange–Dete study site, cattle and buffalo preferred open grassland habitats found close to water. During the rainy season, cattle entered the protected forest area daily, pushed by herders to avoid feeding on the crops growing just outside the protected forest border, and buffalo avoided cattle completely. During the dry season, when cattle ranged further into the protected area in search of forage, buffalo and cattle spatial overlap increased as water dependence took precedence over avoidance (Valls-Fox et al., Reference Valls-Fox, Chamaillé-Jammes and de Garine-Wichatitsky2018).
The role of lions in buffalo–cattle contacts was also explored in the same study site (Miguel et al., Reference Miguel, Grosbois and Fritz2017). Buffalo and cattle avoided the use of the same pasture up to 2 months after one species had used a specific location. Lions made frequent incursions in the interaction zone a few days to weeks after buffalo had used that zone and buffalo avoided areas recently used by lions. Lions could therefore impact the spatiotemporal overlap between cattle and buffalo and therefore buffalo–cattle contacts.
Finally, buffalo/cattle contacts were structured by land-use and resource gradients (mainly water and grazing) as well as the presence of wild predators. The small sample size of these studies (i.e. a few individuals tracked for a dozen months) limits the extrapolation of results at population levels. However, this limit is somehow attenuated by the gregarious organization of both buffalo (in mixed herds) and cattle (in managed herds) for which the movements of a few individuals represent the behaviour of the herd.
From Interspecies Contact to Infectious Contact
Besides direct contacts, the capacity of the pathogen to survive in the environment and to be able to infect another host will determine the temporal window in which transmission can occur. The same applies to vector-borne transmission (e.g. arthropod-borne) with the difference that a spatial window will need to be taken into account in addition to the temporal window to account for the potential mobility of the vector in the environment (Dougherty et al., Reference Dougherty, Seidel and Carlson2018).
Infectious contacts, that is contacts that result in the transmission of one or more pathogens, are invisible ecological processes that are currently impossible to characterize in real-time. An assessment of contacts as presented in the previous section provides some information about the spatial and temporal dynamics of infectious transmission but cannot be directly translated into an assessment of infectious contacts. For example, in the study by Miguel et al. (Reference Miguel, Grosbois and Caron2013), the GPS protocol at the buffalo/cattle interface was completed by a longitudinal survey of 300 cattle, with five repeated sampling sessions undertaken on known individuals over 16 months. Immunological assays, which allow the production of antibodies following infection or vaccination to be tracked, were used to assess serological transitions (i.e. incidence and reversion) in the surveyed cattle. The incidence in the cattle populations of FMD antibodies produced following infection varied among sites and as a function of contact rates with African buffalo. The incidence was higher for sites with higher contact rates between the two species and varied according to the season.
The use of genomics on hosts and pathogens can help in inferring infectious contacts and their direction. Kamath et al. (Reference Kamath, Foster and Drees2016) in the Greater Yellowstone ecosystem estimated the date and the frequency of brucellosis introduction events and found that the disease was introduced into elk (a.k.a. wapiti, Cervus elaphus) from cattle in this region at least five times. The diffusion rate varies among Brucella lineages and over time. They were also able to estimate the direction of transmission between hosts from different species with 12 host transitions from bison (Bison bison) to elk, and five from elk to bison. However, up to now, such a large-scale study using both telemetry and pathogen genetic studies has not been implemented for the characterization of buffalo/cattle interfaces (but see Musoke et al., Reference Musoke, Hlokwe and Marcotty2015).
Space–Time Window as a Proxy of Modes of Transmission
Infectious diseases spread through transmission routes between hosts, and each pathogen can use one or more modes of transmission to ‘jump’ from one host to another. Therefore, as seen for FMD in the previous section, the pathogen of interest and its specific mode(s) of transmission will define the space–time window in which a pathogen can spread from an infected to a susceptible host. The behaviour of both hosts (e.g. cattle and buffalo), the characteristic of the pathogen, and, when relevant, the ecology of the vector will therefore be crucial to estimating the risk of interspecies pathogen spread. This also means that a given contact network between buffalo and cattle can produce very different risks of interspecies spread when considering pathogens with different modes of transmission and similar risks when the modes of transmission and the characteristic of two pathogens are converging. Finally, the data collection method also can impact the quality of the assessment.
To define contacts responsible for FMD transmission between buffalo and cattle, Miguel et al. (Reference Miguel, Grosbois and Caron2013) used a spatial window of 300 m and a temporal window of 15 days. The spatial window took into account both the inaccuracy of the GPS measure and the ability of and cattle to move during a one-hour period. The temporal window accounted for the potential environmental transmission of the virus. Bovine tuberculosis is most often transmitted by respiratory routes, requiring close contact between buffalo and cattle, but the pathogen also can spread by indirect contacts, as the mycobacteria Mycobacterium bovis can survive in faeces for up to 1 month in natural conditions in southern Africa (Tanner and Michel, Reference Tanner and Michel1999). A space–time window to assess the probability of bovine tuberculosis transmission between buffalo and cattle (or vice versa) must take into account direct contacts between both hosts, as well as indirect contacts, with up to 30-day intervals to reflect the survival of the pathogen in faeces. Therefore, a single data set of contact patterns between buffalo and cattle will result in different estimations of the risks of pathogen transmission between species depending on the modes of transmission of the pathogen considered.
Modelling Pathogen Transmission at the Buffalo/Cattle Interface
The dynamics of pathogens in multi-species assemblages are complex. They are influenced by the interaction of each host–pathogen dyad (e.g. morbidity, mortality rate), host population dynamics (e.g. social dynamics, size of groups, intergroup contacts) and interspecies contacts. Various approaches exist to model each of these components, but they have yet to be integrated to produce a holistic model of the buffalo/cattle interface. Here we present examples of modelling approaches to buffalo and cattle population dynamics as well as of interspecies contacts that could support the integration of a pathogen or disease transmission model.
Contact Network and Graph Models
Contact networks, where individuals are represented as nodes and interactions between them as edges, expand the relevance of epidemiological models by capturing the patterns of interaction between individuals (Hamede et al., Reference Hamede, Bashford, McCallum and Jones2009, Reference Hamede, Bashford, Jones and McCallum2012; Yin et al., Reference Yin, de Knegt and de Jong2020). However, realism and precision can limit the applicability of contact data to general contexts (White et al., Reference White, Forester and Craft2015), especially as contact networks are rarely fully described for wildlife species. To address these issues, we can infer the rules behind the generation of contacts within the network and use them to extrapolate the contact structure in the entire population. Exponential random graphs models (ERGMs) provide an appropriate framework to do so. The purpose of ERGMs is to describe parsimoniously the local forces that shape the global structure of a network (Silk et al., Reference Silk, Croft and Delahay2017, Reference Silk, Finn, Porter and Pinter-Wollman2018). To this end, a network data set may be considered as the response variable in a regression model, where the predictors are based on individual traits (gender, age, group), such as ‘the propensity for individuals of the same sex to form partnerships’, or structural metrics of the network (degree, two-stars, triads), such as ‘the propensity for individuals to form a cluster’. The information gleaned from the use of an ERGM may thus be used to understand how contact networks are generated and to simulate new random realizations of networks that retain the essential properties of the observed network, which can be used to simulate disease dynamics (Reynolds et al., Reference Reynolds, Hirsch and Gehrt2015). Such an approach was attempted using the GPS data of 84 collared African buffalo from four populations (Wielgus et al., Reference Wielgus, Cornélis and de Garine-Wichatitsky2020). Unfortunately, no non-random structure of contact was found within the sampled networks because they were missing individuals representing, for example, adult males or juveniles. Nevertheless, ERGMs hold great potential for pathogen transmission modelling within buffalo populations if GPS data from a significant number of individuals within the same population can be sampled for several years.
Spatialized Mechanistic Modelling Approaches
Spatial models integrating the environmental drivers of buffalo and cattle mobility can be developed to assess the potential contacts between the two species and their variations in space and time. For example, the Ocelet domain-specific language and open modelling platform (www.ocelet.fr), based on the tool of interaction graphs (Degenne and Seen, Reference Degenne and Seen2016), allows the implementation of spatialized mechanistic modelling approaches (e.g. Grégoire et al., Reference Grégoire, Chaté and Tu2003) that connect ‘entities’ of different nature (e.g. buffalo, cattle, water bodies, grazing areas), define their interactions (e.g. interspecies relations, species-natural resources dependencies), and simulate their spatiotemporal dynamics. As a result, such models allow the assessment and visualization of the location and frequency of potential contacts between different species based on a wide range of variables that can evolve through time (e.g. animal behaviour, natural resources distribution, human-based social and economic processes, pathogen transmission). Such an approach was used to simulate the impact of the surface water spatial distributions and its seasonal variation on African buffalo movements in a given area (Rumiano et al., Reference Rumiano, Gaucherel and Degenne2021; Figure 10.6). From there, cattle movements can be added to assess the potential contact areas between the two species (Rumiano et al., in prep.), provided that ecological empirical knowledge on focal species is available to feed the model and determine its design. Of note, GPS telemetry data collected from previous works (Miguel et al., Reference Miguel, Grosbois and Caron2013; Valls-Fox et al., Reference Valls-Fox, Chamaillé-Jammes and de Garine-Wichatitsky2018) provide necessary information for calibration (conceptual phase) and validation (assessment phase) of the models.

Figure 10.6 Designed mechanistic model of buffalo movements according to surface water seasonality, geographic location and type of land cover. This movement model is divided into five behavioural phases per 24-hour period (Feeding phase, Rumination phase, To water phase, Watering phase, Free wandering phase) that are based on buffalo behaviour (i.e. median speed per hour) derived from collected telemetry data of three study sites (Miguel et al., Reference Miguel, Grosbois and Caron2013; Valls-Fox et al., Reference Valls-Fox, Chamaillé-Jammes and de Garine-Wichatitsky2018). All individuals move from their starting location to the next at discrete time steps by a fixed distance, their direction defined for each time step as an angle. This angle is correlated to the alignment (α) of each individual with respect to their close neighbours, thus allowing simulation of a collective movement of interdependent individuals (Grégoire et al., Reference Grégoire, Chaté and Tu2003). The value given to α will determine the behaviour of the buffalo. During the ‘Feeding phase’, the buffalo will move until they reach a ‘feeding’ land cover type. During the ‘Rumination phase’, the buffalo stay in motion in the same land cover type. For these two behavioural phases, land cover selections occur within a determined buffer area corresponding to the mean distance travelled per hour (Rumiano et al., in prep.). In the ‘To water phase’, buffalo move towards the closest surface water (varies seasonally) from the buffalo’s herd centroid position at the beginning of the phase. Once buffalo individuals are within 10 m of the targeted surface water point, the ‘Watering phase’ starts and all individuals stop their movements. During the ‘Free wandering’ phase, buffalo move freely in space. Land cover and surface water have been characterized at the landscape scale (10 m of spatial resolution) using supervised and unsupervised classifications on a selected time series of Sentinel-2 satellite images (Rumiano, Reference Rumiano2021). The spatialized classifications have then been integrated into the model thanks to the spatial modelling language Ocelet.
Combining Host Contact and Pathogen Transmission
Once interspecies host population dynamics have been modelled using one of the methodologies presented above, pathogen data can then be coupled with host population modelling to better understand the relationship between environmental drivers, host contacts and pathogen dynamics. This coupling will resolve an important limitation of most epidemiological models that assume homogeneous mixing between naïve and infected hosts, and thus omit the heterogeneity of host behaviour (Lloyd-Smith, Reference Lloyd-Smith2005; Paull et al., Reference Paull, Song and McClure2012). Thus far, the use of such applications in disease ecology has been limited, especially at an interspecies level, despite the importance of interspecies contact patterns on pathogen transmission and the impact of infection on host behaviour (Dougherty et al., Reference Dougherty, Seidel and Carlson2018). New insights into buffalo social dynamics will modify the dynamics of pathogens spread in buffalo groups (Chapter 6; Wielgus et al., Reference Wielgus, Cornélis and de Garine-Wichatitsky2020, Reference Wielgus, Caron and Bennitt2021). Gregarious species with connected and unfragmented social units (classical definition of a mixed herd) should facilitate pathogen spread compared to gregarious species with a higher level of fusion–fission dynamics (Sah et al., Reference Sah, Leu and Cross2017). Similarly, these fusion–fission patterns will have an impact on the risk of pathogen spread between cattle and buffalo (both ways) at interface areas.
Perspectives and Conclusion
Buffalo and cattle interactions and the sustainability of the systems that maintain both species are relevant to the coexistence between humans and nature in Africa. The potential spillover and spillback of pathogens between sympatric buffalo and cattle populations threaten biodiversity conservation, local and national agricultural economies and public health. If buffalo and cattle are to coexist in an open landscape, the sanitary risk will need to be managed according to a new paradigm relative to the level and types of risks that are acceptable. Currently, production systems have not managed to conceive a management process in which both species coexist (Chapters 12 and 14).
Different spatial models of animal movement, contact and interaction taking into account biotic and abiotic ecological features as well as behavioural mechanisms have been developed in recent years (Rastetter et al., Reference Rastetter, Aber and Peters2003; Moorcroft Reference Moorcroft2012; Westley et al. Reference Westley, Berdahl, Torney and Biro2018). Nonetheless, there is a need to further develop mechanistic animal movement, contact and interaction models that integrate independent and validated environmental SRS data enabling landscape-scale analysis of interspecies contact and interaction. Such models could benefit from the integration of especially characterized environmental SRS data while extending their application capacities to different environmental and ecological contexts (Neumann et al., Reference Neumann, Martinuzzi and Estes2015; Rumiano et al., Reference Rumiano, Wielgus and Miguel2020). Several SRS methodologies have already been developed to characterize spatial and temporal variations of environmental drivers, such as surface water (Naidoo et al., Reference Naidoo, Brennan and Shapiro2020) and vegetation (Zengeya et al., Reference Zengeya, Murwira and Caron2015), in relation to buffalo and cattle movements. By allowing the characterization of these environmental drivers at the landscape scale, SRS can improve the understanding of buffalo/cattle contacts and associated disease transmission estimations where in-situ environmental data are lacking.
Mechanistic models, even if they involve significant development and implementation costs, are less dependent on a correlation between ecological processes and environment properties than empirical modelling approaches (Dormann et al., Reference Dormann, Schymanski and Cabral2012). By mathematically simulating interactions and mutual constraints among animal species, mechanistic models improve the transferability to different environments (Kearney and Porter, Reference Kearney and Porter2009). Such models can therefore be adapted specifically for interspecies contacts and interactions by improving focal species ecological behaviour simulations regarding habitat selection and spatial and temporal distributions of natural resources. Advances in GPS telemetry, such as decreasing size, weight and cost of tags, computing power enhancement, and improving battery autonomy and durability, are allowing this technology to be used more efficiently on an expanded range of animal species, but also on animal population subcategories (e.g. female, juvenile, male) while increasing their temporal resolution (Kays et al., Reference Kays, Crofoot, Jetz and Wikelski2015). Improved GPS telemetry technology combined with the rapid growth of SRS use in functional ecology and the enhancement of specialized mechanistic models offer tremendous potential for evaluating inter-species interactions (Rumiano, Reference Rumiano2021). This type of approach can prove to be very valuable in environments such as the buffalo/cattle interfaces in African savannas that are limited in terms of natural resources, highly sensitive to climate condition fluctuations and prone to constant changes in land-use/management practices.
Despite the limits and constraints of these studies, the understanding of buffalo/cattle interactions is crucial to managing the interface and mitigating its negative consequences. Modelling is important to investigate the consequences of some management options that cannot be tested in situ. For example, as resources drive these interactions, appropriate water management could reduce contacts between buffalo and cattle (e.g. Mwakiwa et al., Reference Mwakiwa, de Boer and Hearne2013; Hilbers et al., Reference Hilbers, Van Langevelde and Prins2015). One could suggest manipulating cattle management practices or buffalo behaviours, taking into consideration ethical aspects of animal welfare and transdisciplinary approaches when working with local stakeholders. Modelling also can be important to explore how these interactions will evolve: buffalo/cattle interactions are a moving target as both cattle herding (e.g. pastoralism and agro-pastoralism) and wildlife populations are currently adapting to changing environments (e.g. climate change, human demographic explosion, global markets; Kock et al., Reference Kock, Kock, de Garine-Wichatitksy, Melletti and Burton2014). Modelling can also trigger essential discussions and debates between different actors (e.g. scientists, breeders, political institutions, etc.) and different research disciplines. This implies a participatory platform potentially allowing the integration of virtuous solutions for all (e.g. One Health).
In combination with other emerging initiatives such as commodity-based trade in southern Africa (Thomson et al., Reference Thomson, Penrith and Atkinson2013), the management of buffalo/cattle interactions can be a pillar of a sustainable coexistence between humans and nature in African landscapes (du Toit et al., Reference du Toit, Cross, Valeix and Briske2017). The current focus of the study of these interfaces in southern Africa (and to a lesser extent to East Africa) calls for more studies in different contexts including pastoralism of Central Africa, and different biomes including rainforests in which encroachment by cattle creates new types of interfaces.
Introduction
Parasites, spanning viruses, bacteria, helminths, protozoa and arthropods, live within or on a host, often affecting individual host health, survival and reproduction. Furthermore, these individual-level effects of parasites can have consequences that cascade to the population, community and ecosystem levels (Wilson et al., Reference Wilson, Fenton and Tompkins2019). Historically, host–parasite interactions were studied from a one host–one parasite perspective. However, given that most hosts are infected with more than one type of parasite simultaneously (Cox, Reference Cox2001), the study of concurrent infection (i.e. coinfection) has gained increasing attention from ecologists, epidemiologists, veterinarians and biomedical scientists (Hoarau et al., Reference Hoarau, Mavingui and Lebarbenchon2020; Mabbott, Reference Mabbott2018; Salgame et al., Reference Salgame, Yap and Gause2013). Crucially, wildlife studies occupy a unique niche in this research area because they can help uncover the real-world contexts in which coinfection, and the interactions occurring between coinfecting parasites, are most important (Ezenwa, Reference Ezenwa2016).
Just like free-living species in ecological communities, parasite species live in communities within their hosts where they interact by competing against or facilitating one another, with consequences for parasite community structure, host health and host fitness (Beechler et al., Reference Beechler, Boersma and Buss2019; Graham, Reference Graham2008; Pedersen and Fenton, Reference Pedersen and Fenton2007; Telfer et al., Reference Telfer, Lambin and Birtles2010). Many of the initial efforts to study parasite interactions in wildlife focused on co-occurrence patterns, revealing that coinfection is common, and that parasites and pathogens interact within hosts both directly and indirectly (e.g. Bush and Holmes, Reference Bush and Holmes1986; Lello et al., Reference Lello, Boag and Fenton2004). For instance, parasites may compete for space or resources (Budischak et al., Reference Budischak, O’Neal, Jolles and Ezenwa2018a; Clerc et al., Reference Clerc, Fenton, Babayan and Pedersen2019), such that the presence of one parasite decreases the likelihood another succeeds at growth and replication. Alternatively, one parasite may increase the success of another by providing resources or space (Dutt et al., Reference Dutt, Anthony and Andrivon2021; Zélé et al., Reference Zélé, Magalhães, Kéfi and Duncan2018). Parasite community interactions are further governed by the host immune response, where cross-immunity may cause one parasite to negatively affect the establishment and growth of another (Raberg et al., Reference Raberg, de Roode and Bell2006), or where one parasite may suppress the host immune response in a way that is beneficial to other parasites (Graham, Reference Graham2008). Recently, advances in molecular, immunological and statistical methods have enabled an increasingly mechanistic and/or predictive understanding of these types of parasite interactions in wild species (e.g. Clerc et al., Reference Clerc, Fenton, Babayan and Pedersen2019; Fountain-Jones et al., Reference Fountain-Jones, Packer and Jacquot2019; McDonald et al., Reference McDonald, Longo, Lips and Zamudio2020).
Studies of African buffalo have played a key role in advancing research on wildlife coinfection. Multiple facets of African buffalo ecology and life history make them an excellent system for understanding parasite interactions in free-living animals (Ezenwa et al., Reference Ezenwa, Jolles, Beechler, Budischak, Gorsich, Wilson, Fenton and Tompkins2019). Buffalo are relatively long-lived, large-bodied, gregarious animals that are common throughout sub-Saharan Africa. Furthermore, buffalo are host to a broad diversity of parasites, including bacteria, viruses, protozoa and helminths (Ezenwa et al., Reference Ezenwa, Jolles, Beechler, Budischak, Gorsich, Wilson, Fenton and Tompkins2019). These attributes allow parasite studies to be conducted on relatively large numbers of individuals across multiple spatiotemporal scales (Garabed et al., Reference Garabed, Jolles and Garira2020). Physiological similarity between domestic cattle and African buffalo further enables the use of readily available physiological (Couch et al., Reference Couch, Movius and Jolles2017), immunological (Beechler et al., Reference Beechler, Broughton and Bell2012) and diagnostic tools (Glidden et al., Reference Glidden, Beechler and Buss2018), as well as therapeutics to measure animal responses to infection, describe parasite community composition (Beechler et al., Reference Beechler, Boersma and Buss2019), and manipulate host–parasite interactions (Ezenwa and Jolles, Reference Ezenwa and Jolles2015). In this chapter, we describe insights on parasite interactions derived from the study of African buffalo. Focusing on results drawn from two large studies performed in Kruger National Park (KNP), South Africa (see Box 11.1), we discuss how pairwise and multi-parasite perspectives have been used to understand which parasite taxa interact most strongly, the mechanisms accounting for these interactions and the implications for both hosts and parasites. We also highlight general patterns that have emerged across parasite systems. We outline key technical tools, both computational and laboratory, that facilitate the ability to draw strong inferences and link phenomena across scales. We conclude by identifying future research directions that will help advance scientists’ understanding of the causes and consequences of parasite interactions.
Box 11.1 Studying Parasite Interactions in the Wild
Experimental and longitudinal approaches are important ingredients for studying parasite interactions in natural systems. By directly manipulating parasites in situ, researchers can identify how co-occurring parasites, as well as hosts, respond to changes in the parasite community and simultaneously investigate factors both internal and external to the host that govern variation in observed responses. Likewise, longitudinal approaches allow for parasite and host characteristics to be tracked over time, providing insight about the order in which events occur and helping to distinguish cause from effect. Either approach is valuable on its own, but in combination, these two methods represent a powerful tool for unravelling the causes and consequences of parasite interactions in free-ranging wildlife. Studies on wild African buffalo in KNP used these approaches to address a range of questions about parasite interactions.
Study 1 followed ~200 free-ranging young female buffalo captured from two herds in southern KNP over a four-year period. The animals were fitted with VHF collars (see Figure 11.1a) with recaptures occurring every 6 months to monitor changes in parasite communities, host physiology, health and performance (see Table 11.1). These animals were captured in the south-eastern portion of the park and animals were allowed to move and disperse as normal (Spaan et al., Reference Spaan, Epps, Ezenwa and Jolles2019). A goal of the study was to understand how gastrointestinal worms and bovine tuberculosis (bTB) interact, so half of the study animals received a long-acting anthelmintic drug applied every 6 months to reduce their worm burdens, while the other half were used as controls. Study animals were bTB-free at the onset of the experiment so that effects of anthelmintic treatment on bTB infection incidence and severity could be quantified.

(a) African buffalo fitted with a VHF collar.

(b) Double fence surrounding the 900 ha semi-natural enclosure containing the buffalo herd of Study 2.
Table 11.1 Measures of health and immunity used in African buffalo.
Measure | Method | Citation |
---|---|---|
Physiology and health | ||
Body condition | Manual palpation of buffalo | Ezenwa et al. (Reference Ezenwa, Jolles and O’Brien2009) |
Pregnancy status | Rectal palpation | Beechler et al. (Reference Beechler, Manore and Reininghaus2015) |
Lactation status | Manual milking of teats | NA |
Cortisol as a measure of stress | Radioimmunoassay of faecal samples | Spaan et al. (Reference Spaan, Pitts and Buss2017) |
Haematocrit and red blood cell measurements | Haematological assessment of whole blood | Beechler et al. (Reference Beechler, Jolles and Ezenwa2009) |
Total protein, albumin, kidney and liver enzymes | Chemistry profile on plasma | Couch et al. (Reference Couch, Movius and Jolles2017) |
Immunity | ||
White blood cell counts | Blood smear on whole blood | Beechler et al. (Reference Beechler, Jolles and Ezenwa2009) |
Bacteriacidal ability | Bacterial killing assay on plasma and whole blood | Beechler et al. (Reference Beechler, Broughton and Bell2012) |
Lymphocyte proliferation ability (LPA) | Whole blood LPA | Beechler (Reference Beechler2013) |
Cytokines (IFNy, IL4, TNFa, IL12) | Enzyme-linked immunosorbent assay (ELISA) of plasma | Beechler et al. (Reference Beechler, Manore and Reininghaus2015); Ezenwa and Jolles (Reference Ezenwa and Jolles2015); Glidden et al. (Reference Glidden, Beechler and Buss2018) |
Acute phase proteins (SAA, Hapto) | ELISA of plasma | Glidden et al. (Reference Glidden, Beechler and Buss2018) |
Total globulins | Chemistry profile on plasma | Couch et al. (Reference Couch, Movius and Jolles2017) |
Study 2 followed one herd of ~80 mixed age and sex buffalo, housed in a 900 ha semi-natural, predator-free enclosure in central KNP (see Figure 11.1b) that had been in place since the early 2000s and managed by KNP veterinary wildlife services. In this ‘mesocosm’ setting, study animals were captured every 2–3 months to collect finer-scale information on parasite communities and host traits. This short capture interval allowed for a better understanding of transmission patterns of microparasites like viruses and bacteria that are quick to spread throughout a population.
For both studies, samples (e.g. blood, faeces) collected at capture were used to quantify and describe the parasite community. These samples were also used to perform a suite of assays to assess host physiology, immunity and overall health (see Table 11.1). In combination with information about external environmental conditions (e.g. seasonality), the data on host traits and parasites were used to test a range of hypotheses about the nature and implications of parasite interactions. For both studies, animal handling and scientific permits were acquired from appropriate institutions (see Ezenwa and Jolles, Reference Ezenwa and Jolles2015 for Study 1 and Jolles et al., Reference Jolles, Gorsich and Gubbins2021 for Study 2 permit information).
Parasite Interactions: Combining Pairwise and Multi-Species Perspectives
Studies of parasite interactions in African buffalo have ranged in scale from studies focused on pairwise parasite interactions to studies examining interactions among multiple co-occurring parasites using taxonomic and trait-based approaches (Table 11.2). In most cases, a key goal of the work has been to uncover how the presence of more than one parasite modifies host and parasite responses to infection. Below, we review these studies to identify notable commonalities across them as well as methods used to develop a multi-parasite perspective. Findings highlight the value of pairwise and multi-parasite perspectives: integrating both perspectives identified immunological and ecological mechanisms underlying pairwise interactions and assessed the relative importance of those mechanisms in more complex parasite communities.
Table 11.2 Summary of research on parasite interactions in African buffalo. The studies span a range of designs, including experimental (E), longitudinal (L) and case control (CC) studies. The design column indicates the study design type and whether the data were derived from Study 1 or 2 as described in Box 11.1. The scale column indicates whether the study focused on two parasites (pairwise), a group of parasites (e.g. helminths or Theileria), or all of the parasites that were screened (WC, for whole community). It also describes whether the interaction was between microparasites (micro–micro), macroparasites (macro–macro) or both (macro–micro). The interaction type and effects columns define whether the parasites positively (facilitation) or negatively (competition) influenced each other during a subsequent observation or across multiple observations (succession).
Parasites (diseases) | Design | Scale | Interaction type | Effects on other parasite/parasite communities/host | Citation |
---|---|---|---|---|---|
Theme 1: Pairwise studies | |||||
Mycobacterium bovis (bTB) vs. Brucella abortus (brucellosis) | L – 1 | Pairwise. Micro–micro | Facilitation and competition | Effects on other parasite: Brucellosis infection was twice as likely in buffalo with bTB compared to uninfected buffalo, but brucellosis infection was not correlated with risk of bTB. Mathematical modelling suggests the net effect of bTB on transmission and mortality results in competition at the population-level: R0 and endemic prevalence predictions for bTB were lower in populations in which both pathogens co-occur. Effects on host: Mortality rates were higher after individuals became infected with either bTB or brucellosis, with the highest risk occurring in co-infected buffalo. Neither infection reduced fecundity, measured by calf observations. | Gorsich et al. (Reference Gorsich, Etienne and Medlock2018), |
bTB vs. helminths | E – 1 | Pairwise. Micro–macro | Competition | Effects on other parasite: Experimental anthelmintic treatment did not influence risk of bTB. The predicted R0 of bTB is 8-fold higher in treated populations due to decreases in mortality when worm burdens decline. Effects on host: Mortality rates were lower in buffalo that received the treatment compared to controls. | Ezenwa and Jolles (Reference Ezenwa and Jolles2015), |
bTB vs. helminth resistance | L/E – 1 | Pairwise, Micro–macro | Competition | Effects on other parasite: There was no effect of natural host resistance to worms on bTB infection risk. Effects on host: Worm-resistant individuals were more likely to die of bTB than were non-resistant individuals despite having lower worm burdens, and bTB progressed more quickly in the lungs of non-resistant individuals. Anthelmintic treatment moderated but did not eliminate this pattern, implicating ‘resistance’ to worms and not simply current worm infection as a driver of the interaction. | Ezenwa et al. (Reference Ezenwa, Budischak and Buss2021) |
bTB vs. Rift Valley fever virus | L – 1 | Pairwise. Micro–micro | Facilitation | Effects on other parasite: RVF infection was twice as likely in buffalo with bTB compared to uninfected buffalo. Mathematical modelling suggests this results in larger and faster spreading RVF outbreaks. Effects on host: Foetal abortion rates were 6.6 times higher in coinfected buffalo compared to infection with RVF alone. | Beechler et al. (Reference Beechler, Manore and Reininghaus2015) |
Helminths, namely Haemonchus spp. vs. Cooperia fuelleborni | E – 1 | Pairwise. Macro–macro | Effects on host: Host body condition was lower in buffalo that underwent experimental anthelmintic treatment compared to controls. In controls, increases in Haemonchus egg counts were negatively associated with changes in condition, while increases in Cooperia egg counts were associated with increases in condition. Neither parasite directly influenced survival or fecundity (likelihood of being pregnant or lactating). However, treated buffalo had higher survival and both treated and untreated buffalo in good condition had both higher survival and fecundity. | Budischak et al. (Reference Budischak, Wiria and Hamid2018b) | |
Schistosoma matthei vs. Cooperia fuelleborni | –L – 1 | Pairwise. Macro–macro | Facilitation | Effects on other parasite: Schistosome burdens varied seasonally. Wet season gains in burden were not correlated with helminth coinfection, but coinfection did influence the magnitude of dry season reductions in burden. Buffalo infected with Cooperia maintained higher schistosome burdens throughout the dry season. | Beechler et al. (Reference Beechler, Jolles and Budischak2017) |
Parasites (diseases) | Design | Scale | Interaction type | Effects on other parasite/parasite communities/host | Citation |
---|---|---|---|---|---|
Theme 2: Multi-parasite studies applying taxonomic approaches | |||||
6 gastrointestinal helminthsFootnote a | –E – 1 | Helminths | Succession | Effects on parasite community: After anthelmintic treatment, helminth communities in treated buffalo had a lower total abundance and higher diversity compared to communities in undisturbed control buffalo. With increasing time since treatment, treated helminth communities resembled those found in undisturbed control buffalo. | Budischak et al. (Reference Budischak, Hoberg and Abrams2016) |
6 respiratory pathogens associated with bovine respiratory diseaseFootnote b | L – 1 | WC | Facilitation | Effects on parasite community: Five of the respiratory pathogens were continuously circulating. Viral coinfection was the best predictor of viral infection; host physiology and season had little effect on odds of viral infection. Coinfection with bTB was positively associated with risk of BRSV but none of the other pathogens. Anti-helminthic treatment was not associated with any of the respiratory pathogens. | Glidden et al. (Reference Glidden, Coon and Beechler2021) |
10 Theileria phylotypesFootnote c | –L – 1 | Theileria | Facilitation, competition, succession | Effects on parasite community: Interaction networks change over time; young animals are infected with Theileria interaction networks composed of many negative and positive interactions, while adult interaction networks are composed of three positive interactions. 7/10 phylotypes exist with 80–90% prevalence in adult animals, this coexistence is likely the result of phylotype-specific immunity and facilitation. Two phylotypes infect young animals early (within 1 month), but are later displaced in adult animals and facilitation. | Glidden et al. (Reference Glidden, Coon and Beechler2021), |
Theme 3: Multi-parasite studies applying trait-based perspectives | |||||
bTB, brucellosis, 3 haemoparasites,Footnote d 6 respiratory pathogensFootnote b coccidia, strongyle nematodes, Schistosoma matthei | C–C – 1 | WC | – | Effects on parasite community: Animals that acquired bTB experienced a greater increase in parasite assemblage richness and functional richness compared to age-matched buffalo that did not acquire bTB. The traits of parasite communities after bTB were less variable (measured as multivariate dispersion) and dominated by contact-transmitted parasites with simple life cycles and fast replication times. | Beechler et al. (Reference Beechler, Boersma and Buss2019), Figure 11.4 |
11 haemoparasitesFootnote d vs. BTB, brucellosis, helminths, coccidia, ticks (Ambylomma hebraeum, Rhipicephalus spp.) | L – 1 | WC | Facilitation and competition | Effects on parasite community: Parasites infecting the same tissue type were associated with the probability of haemoparasite infection (e.g. other haemoparasites). For pairs of haemoparasites, the direction of the association can be predicted based on shared resources, cross immunity, and having a shared vector. In contrast, associations between haemoparasites and parasites infecting other tissue types were weak or non-existent. | Henrichs et al. (Reference Henrichs, Oosthuizen and Troskie2016) |
Tick-borne parasites Gastrointestinal parasites Respiratory pathogens | –L – 2 | WC | – | Effects on parasite community: Parasite communities follow patterns of succession similar to free-living communities. The median age of first infection differs between parasite taxa, with tick-borne and helminths parasites first occurring in animals less than 1 year old and directly transmitted infections first occurring after 2 years. | Combrink et al. (Reference Combrink, Glidden and Beechler2020) |
a Cooperia fulleborni, Haemonchus sp., Parabronema sp., Trichostrongylus sp., Africanastrongylus giganticus, Africanastrongylus buceros.
b Bovine adenovirus-3 (AD-3), bovine herpes virus (BHV), bovine parainfluenza-3 (Pi-3), bovine respiratory syncytial virus (BRSV), Mycoplasma bovis (MB), Mannheimia haemolytica (MH).
c T. parva, T. sp (buffalo), T. sp (bougasvlei), T. velifera, T. velifera B, T. mutans-like 1, T. mutans-like 2, T. mutans-like 3, T. mutans, T. mutans MSD.
d Anaplasma centrale, A. marginale, A. sp. Omatjenne, A. phagocytophilum, Babesia sp., Ehrlichia ruminantum, Theileria parva, T. mutans, T. sp (buffalo), T. sp (sable), T. velifera.

(a) The estimated reproductive number (R0) of bTB in buffalo subpopulations that did (treated) versus did not (control) receive anthelmintic drug treatment. R0 was approximately eight times higher for treated individuals (2 vs. 15.5), with upper and lower estimates of 3.4 and 69.8, respectively.

(b) The estimated prevalence of bTB in buffalo populations with single bTB (left panel, line with circle) or concurrent bTB and brucellosis (left panel, line with triangle) infections. bTB prevalence declined in the presence of brucellosis, but there was no reciprocal effect of bTB on Brucella prevalence (right panel, line with circle vs. line with triangle).
The studies of pairwise parasite interactions cover a broad taxonomic scope, including bacteria, viruses, protozoa and helminths (Table 11.2, Theme 1). They investigate parasite interactions and the consequences for hosts and parasites in real-world settings. For example, in laboratory studies, immunological mechanisms of interaction between parasites are well described, but if and how these interactions manifest in wild populations has been unclear. Early work in African buffalo provided seminal evidence that cross-regulated immune responses can shape parasite population dynamics (Jolles et al., Reference Jolles, Ezenwa and Etienne2008), and that as in laboratory rodents, infection with parasites like gastrointestinal helminths can induce immune cross-regulation (Ezenwa et al., Reference Ezenwa, Etienne, Luikart, Beja-Pereira and Jolles2010). Studies in the KNP buffalo population (Box 11.1) expanded on this foundation using manipulative experiments and longitudinal tracking of individuals to confirm that in a wild setting, clearance of one type of parasite (gastrointestinal helminths) has ramifications for host immunity and the severity of infection with a second parasite, in this case Mycobacterium bovis, the causative agent of bovine tuberculosis (bTB) (Ezenwa and Jolles, Reference Ezenwa and Jolles2015). Another key result from the pairwise studies was the broad importance of bTB on host immunity, health and survival. M. bovis infection was associated with lower innate immunity and higher inflammatory cytokine secretion, measured as Escherichia coli killing capacity and interleukin-12 concentration, respectively (Beechler et al. Reference Beechler, Broughton and Bell2012, Reference Beechler, Manore and Reininghaus2015). Accordingly, prior infection with bTB was associated with an increased likelihood of acquiring both Brucella abortus (the causative agent of brucellosis) and Rift Valley fever virus (RVF) (Beechler et al., Reference Beechler, Manore and Reininghaus2015; Gorsich et al., Reference Gorsich, Etienne and Medlock2018). These studies provide a starting platform for investigating the consequences of bTB for a wider range of co-occurring parasites. Given the broad effects of bTB on African buffalo survival, health and susceptibility to other parasites, mechanistic models are useful to predict the consequences of bTB for the population-level dynamics of a second parasite. For example, Gorsich et al. (Reference Gorsich, Etienne and Medlock2018) parameterized a mechanistic model of bTB–brucellosis dynamics that represented a host’s increased likelihood of acquiring brucellosis and increased mortality rates if the host was also infected with bTB. The model predicted the net consequences of these effects for prevalence and R0, thereby linking the within-host mechanisms explored in previous papers (Beechler et al., Reference Beechler, Broughton and Bell2012, Reference Beechler, Manore and Reininghaus2015) to population-level patterns of disease spread.
Further studies investigated multi-parasite interactions by applying a multi-parasite approach (Table 11.2, Themes 2 and 3). These studies can be conceptually divided into those that quantify higher-order association patterns that emerge from complex multi-parasite interactions (Theme 2) and those that simplify complex multi-parasite interactions into generalizable patterns (Theme 3). The former relies on taxonomic approaches where parasites are classified according to their taxonomy, while the latter applies a trait-based perspective where parasites are classified by their biological features, such as how they differ in physiological, morphological or life-history traits. The two approaches reveal how different dimensions of the parasite community respond to change. However, because traits or trait distributions can be directly linked to host or environmental conditions, trait-based approaches allow for more mechanistic predictions about how a community may change in response to coinfection (see McGill et al., Reference McGill, Enquist, Weiher and Westoby2006 for a review of traits-based approaches in ecology).
Importantly, the multi-parasite approaches (Table 11.2, Themes 2 and 3) and the pairwise approaches (Table 11.2, Theme 1) have proven highly complementary. For example, Beechler et al. (Reference Beechler, Boersma and Buss2019) applied a trait-based approach to evaluate how parasite community composition – including 14 parasites ranging from viruses, bacteria, protozoa and helminths – differed before versus after buffalo were infected with bTB. Interestingly, parasite communities tended to have higher taxonomic and functional richness (e.g. unique parasite traits) after hosts acquired bTB infection (Figure 11.4a). Furthermore, while the number of unique parasites tended to increase after bTB, the traits of these parasites were functionally similar to each other, as quantified by multivariate dispersion. Multivariate dispersion measures the amount of trait space occupied by a given community; thus, after bTB infection, parasite communities became more homogenous in terms of their traits even though the communities contained more species. This pattern was associated with communities becoming dominated by certain traits (e.g. contact transmission, fast replication rate), with less representation of other traits (slow transmission rate, environmental transmission).
Taxonomic studies occurring in the same system corroborate this result. The dominance of contact-transmitted, fast-replicating parasite taxa is supported by a longitudinal, multi-parasite study investigating associations among five contact-transmitted, fast-replicating respiratory pathogens (Theme 2). This analysis showed that after accounting for bTB infection, pathogen co-occurrence explained the largest proportion of variation in three focal viruses (bovine adenovirus-3, bovine herpes virus-1, bovine parainfluenza-3), with all three positively influenced by coinfection (Glidden et al., Reference Glidden, Coon and Beechler2021). Additionally, the lack of slowly transmitted or environmentally transmitted pathogens is supported by pairwise-longitudinal and experimental studies investigating associations between bTB, brucellosis and gastrointestinal nematodes (Theme 1). Coinfection with both gastrointestinal nematodes and brucellosis was associated with higher mortality in bTB-positive individuals (Ezenwa and Jolles, Reference Ezenwa and Jolles2015; Gorsich et al., Reference Gorsich, Etienne and Medlock2018). These examples illustrate the value of combining approaches to investigate parasite communities.
Notable Commonalties Across Studies
Studying diverse parasite interactions can reveal commonalities across combinations of parasites that provide new insight into how the consequences of coinfection manifest in nature. At least one such common thread has emerged from studies of parasite interactions in wild African buffalo – the presence of conflicting outcomes across scales. Two studies of pairwise parasite interactions, one focused on interactions between gastrointestinal helminths and bTB (Ezenwa and Jolles, Reference Ezenwa and Jolles2015), the other focused on interactions between brucellosis and bTB (Gorsich et al., Reference Gorsich, Etienne and Medlock2018), both found evidence that from a host perspective, whether the outcome of coinfection is negative or positive differs at the individual host versus population scale.
Understanding the mechanisms that cause interactions between parasites is a cornerstone of coinfection research, in large part because uncovering these mechanisms should facilitate the development of effective disease intervention and control strategies. Immunological mechanisms are widely implicated as a driver of interactions between helminth parasites and many microbial pathogens (e.g. viruses, bacteria). For example, in mammals, helminth infections typically trigger a T-helper cell 2 (Th2)-type immune response, but the upregulation of this response can suppress T-helper cell 1 (Th1)-type immune responses directed against microbial pathogens (Mosmann and Sad, Reference Mosmann and Sad1996). The individual-level repercussions of this immune cross-regulation can include any or all the following: increased susceptibility to microbial infection, faster disease progression and more severe disease (Ezenwa and Jolles, Reference Ezenwa and Jolles2011). Moreover, these individual-level effects may scale up to influence the spread of microbes at the population-level if their combinatorial effects are sufficient to alter microbial population growth rates (Fenton, Reference Fenton2008). Consequently, in populations where hosts are faced with concurrent helminth and microbe infection, treating individuals for their worms (e.g. via anthelmintic drug therapy) may be an effective strategy for mitigating the negative health impacts of certain microbial infections and reducing the population-level spread of these microbes (Hotez et al., Reference Hotez, Molyneux and Fenwick2006). However, how eliminating helminths will affect the population dynamics of a coinfecting microbe depends on the net effect of helminths on the different parameters relevant to microbial transmission.
The anthelmintic treatment study of wild buffalo in KNP (Box 11.1, Study 1) tracked the effect of experimental deworming on two key parameters that influence bTB dynamics in buffalo: (i) the probability that an individual becomes infected with the disease, and (ii) the disease-associated mortality rate. Results showed that although treatment boosted buffalo anti-bTB immunity, treated animals were equally likely as untreated controls to acquire bTB (Ezenwa and Jolles, Reference Ezenwa and Jolles2015). In contrast, treatment drastically reduced bTB-associated mortality, with treated animals almost nine times less likely to die of their bTB infections compared to controls (Ezenwa and Jolles, Reference Ezenwa and Jolles2015). The population-level consequences of these effects were estimated by considering the impact of treatment on the basic reproductive number (R0) of bTB, a metric that generally reflects how fast a pathogen can spread in a host population. Theoretically, anthelmintic treatment can either decrease or increase the R0 of bTB, but in this case, treatment was associated with a nearly eightfold increase in bTB’s R0 (Ezenwa and Jolles, Reference Ezenwa and Jolles2015). As R0 is defined as the number of secondary cases produced by a single infected individual in a fully susceptible population, this means that, on average, an anthelmintic-treated buffalo infects eight conspecifics with bTB for every one infected by an untreated buffalo. Thus, although anthelmintic treatment has a positive outcome for individual health (i.e. bTB infected buffalo that receive treatment survive better), there appears to be a population-level cost of this strategy in terms of faster bTB spread (Figure 11.2a). This conflicting outcome likely arises because of the asymmetrical effects of worm treatment on bTB infection probability and mortality – as anthelmintic-treated, bTB-infected buffalo live longer (positive individual-level effect), they have more time to spread the disease to others (negative population-level effect). In the real world, therefore, broad-scale anthelmintic treatment and elimination or eradication of helminth parasitism may have unintended effects on the dynamics of certain microbial infections like bTB, despite vastly improving individual health outcomes.
In many cases, the within-host mechanisms underlying interactions between parasites are unknown, and a priori hypotheses about potential modes of intervention or control are lacking. Nevertheless, longitudinal studies can be used to understand the impacts of coinfection on both individuals and populations and extract insight about potential consequences of control strategies. Interactions between bTB and brucellosis in buffalo were studied in this way, with results pointing to another intriguing case of conflicting outcomes for individuals and populations. Taking advantage of the coupled longitudinal design of Study 1 (Box 11.1), the interactions between these two chronic bacterial infections on the risk of infection of buffalo to each pathogen and mortality were examined. While bTB infection increased brucellosis risk, there was no consistent effect of brucellosis on bTB infection risk, revealing an asymmetry in the effects of these pathogens on one another (Gorsich et al., Reference Gorsich, Etienne and Medlock2018). In terms of mortality risk, buffalo infected with both bTB and brucellosis experienced a more than eightfold increase in mortality compared to uninfected buffalo (Gorsich et al., Reference Gorsich, Etienne and Medlock2018). A mathematical model exploring how these changes in infection risk and mortality affected the R0 and prevalence of both pathogens showed that the presence of brucellosis reduced both the R0 of bTB and population-level prevalence, whereas the presence of bTB had no consistent effect on brucellosis (Gorsich et al., Reference Gorsich, Etienne and Medlock2018). Thus, even though bTB infection renders individual buffalo more susceptible to brucellosis, the presence of brucellosis moderates the prevalence of bTB at the population level (Figure 11.2b), highlighting yet another conflicting pattern across scales. In this case, the conflict arises because buffalo infected with bTB are more likely to acquire brucellosis and die (a negative individual-level outcome); however, this decreases the timeframe over which they can spread bTB to others, thereby reducing bTB prevalence at the population level (a positive population-level outcome). This result means that coinfection can help moderate the population-level spread of pathogens in certain circumstances, even if the individual-level outcomes of coinfection are undesirable.
These two case studies reveal an intriguing contrast between individual- and population-level consequences of infectious diseases in multi-parasite systems. Both examples suggest that the presence of one parasite or pathogen (helminths, brucellosis) can moderate the spread of another (bTB). In both contexts, cross-scale contrasts arise because the relative effects of helminths and brucellosis on individual host susceptibility to bTB are negligible compared to effects on mortality during coinfection (Gorsich et al., Reference Gorsich, Etienne and Medlock2018). If this imbalance between mortality and susceptibility/transmission effects is general, the implications for designing disease control and intervention strategies may be applicable to multiple host–pathogen systems. In wildlife, single-pathogen focused disease control and management programmes may inadvertently increase the prevalence of non-target infections or facilitate the invasion of novel pathogens. More generally, the presence of conflicting cross-scale outcomes raises intriguing new questions about parasite interactions. Among these questions, identifying the circumstances in which such conflicting outcomes are most likely, including the host and parasite attributes that contribute to this pattern, represent new frontiers in research on coinfection.
Tools for Studying Parasite Interactions
The growing understanding of the community ecology of buffalo parasites is in part attributable to the variety of empirical approaches (e.g. experiments, longitudinal tracking, case control designs) that have been used to draw inference as well as the parallel development of new computational and laboratory tools. Here, we highlight these tools as they apply to the studies described in Table 11.2.
Pairwise Interactions: Causes and Consequences
Expanding our knowledge of host- versus parasite-level outcomes of coinfection is one of the largest contributions the buffalo study system has made to parasite community ecology. The ability to track large numbers of individual buffalo make them well suited for revealing these processes. Specifically, following animals through time allowed for parameterization of dynamical compartmental models as the number of susceptible, infected, coinfected and recovered (in some cases) individuals could be accurately assessed in real-time, and fecundity and/or survival rates could be parameterized using time-to-event analyses (Beechler et al., Reference Beechler, Bengis and Swanepoel2015; Ezenwa and Jolles, Reference Ezenwa and Jolles2015; Gorsich et al., Reference Gorsich, Etienne and Medlock2018). As dynamic compartmental models integrate multi-scale information to describe mechanistic processes, this methodological framework paints a clear and robust picture of multi-scale outcomes of parasite coinfection. Notably, in Ezenwa and Jolles (Reference Ezenwa and Jolles2015), the application of an anthelmintic drug typically used for cattle supported causal inference as well as guaranteed a relatively even sampling design of helminth infection status across animals.
A longitudinal study design and application of an anthelmintic drug also aided Budischak et al. (Reference Budischak, Hoberg and Abrams2016) in revealing patterns of parasite succession and in characterizing the outcome of a gain in infection on host health. Likewise, Beechler et al. (Reference Beechler, Jolles and Budischak2017) benefited by uncovering seasonally dependent effects of helminth infection on schistosome loss. In both of these studies, mixed effects models were able to parse out the effect of explicit covariates and animal-level random variation. As such, not only do these methods account for repeated measures and non-independence among samples, but they also identify the presence of latent animal traits not included in the model.
Community-Wide Analyses: Characterizing Complexity and Emergent Patterns
Advancing statistics and diagnostic approaches have been crucial in understanding communities beyond pairwise interactions. The ubiquity of concomitant infections in African buffalo has made it an ideal system for application of these techniques. For example, Glidden et al. (Reference Glidden, Coon and Beechler2021) used diagnostic tools initially developed for cattle to identify five respiratory pathogens infecting buffalo. Conditional Markov-random field models were then used to estimate the relative effect of the associations among all pathogens versus animal traits related to animal exposure and susceptibility on odds of infection. Notably, these methods can also be used to estimate how association strength among pathogens varies with host traits. In the study described here, the authors found that association strength differed with herd membership and animal lactation status (Glidden et al., Reference Glidden, Coon and Beechler2021). If examining interaction networks at multiple scales (individual, population, meta-population) joint-species distribution models can yield similar results (Fountain-Jones et al., Reference Fountain-Jones, Packer and Jacquot2019; Tikhonov et al., Reference Tikhonov, Abrego, Dunson and Ovaskainen2017).
Advanced time series analyses can also be used to detect causal associations (i.e. true interactions) between parasites in longitudinal observational data, without an experimental component, thereby making it possible to quantify multidimensional parasite communities. In Glidden (Reference Glidden2020), empirical dynamical modelling, a technique that uses time series to detect information transfer among variables (Clark et al., Reference Clark, Ye and Isbell2015), was paired with high throughput sequencing of an 18S genus-specific marker specific to describe non-linear and time-varying interactions among 12 subtypes of Theileria. This empirical dynamical modelling revealed that parasite interaction complexity decreases as animals age, with adult animals’ interaction networks containing only four facilitative interactions and no competitive interactions, whereas the Theileria community was connected via a dense web of both facilitative and competitive interactions in juvenile animals. General additive mixed models were then used to estimate the non-linear relationships among interaction strengths and host immune response, detecting a correlation between antibody concentration and mean interaction strength, suggesting that change in interaction networks may be related to shifts in immune dynamics. In this context, panel regression models are also a powerful tool as they use time series of multiple units (e.g. individuals) to detect causal relationships (Dudney et al., Reference Dudney, Willing and Das2021). If considering linear interactions, autoregressive models (Solvang and Subbey, Reference Solvang and Subbey2019) can similarly leverage time series data to identify causal interactions and characterize true interaction networks (Clark et al., Reference Clark, Ye and Isbell2015). Overall, increasingly accessible computational tools have expedited insight on the complex and interacting factors shaping high-dimensional pathogen community assemblages, with particularly novel tools allowing for causal inference from observational studies.
Trait-Based Analyses: Bridging Complexity to Prediction and General Rules
In recent years, the community ecology of free-living organisms has moved to expand characterization of biodiversity to include functional and trait-based descriptions. The sharing of pathogens of veterinary importance between livestock and buffalo has resulted in a fairly detailed knowledge of pathogen traits in African buffalo (e.g. infected tissue, transmission route), enabling classification of functional diversity. Unsupervised machine learning methods were used by Beechler et al. (Reference Beechler, Boersma and Buss2019) to cluster pathogen communities by functional traits pre- and post-bTB infection. Classification by traits has also eased interpretation of grouped analyses such as in Combrink et al. (Reference Combrink, Glidden and Beechler2020), where time-to-event analyses were used to measure age of first infection across a range of parasite and pathogen taxa, and clear patterns emerged based upon pathogen transmission route and taxonomic group where animals were typically first infected by tick-borne protozoa and last infected with directly transmitted respiratory viruses and bacteria. Combrink et al.’s (Reference Combrink, Glidden and Beechler2020) investigation was made possible by the ability to track African buffalo from birth, allowing observation of natural parasite succession. The same study system (Study 2, Box 11.1) was used to identify patterns of succession of Theileria subtypes, while the application of non-linear regression and high-throughput sequencing allowed for a fine-scale identification of groups of Theileria subtypes with unique life-histories (colonization, relative abundance in adults; Figure 11.3).

Figure 11.3 The succession of Theileria subtypes in African buffalo demonstrates the unique applicability of combining longitudinal study designs with high-throughput sequencing to identify how pathogen communities change over time. By combining infection time series with information on host traits (Table 11.1) we can determine the assembly processes that shape African buffalo parasite communities. Here, the bTB axis represents within-host parasite relative abundance, the x-axis represents animal age. The regression line is the output of a general additive mixed model with a Dirichlet-multinomial distribution, allowing for modelling composition and clustered data.

Figure 11.4 Bovine tuberculosis (bTB) infection altered the parasite communities in buffalo. By assessing changes in parasite communities both taxonomically (by species and genus) as well as functionally (e.g. using parasite traits such as speed and site of replication), Beechler et al. (Reference Beechler, Boersma and Buss2019) showed that animals that acquired bTB had higher parasite richness after bTB (phase 2) than before (phase 1) both taxonomically (panel a) and functionally (panel b). Furthermore, the magnitude of this increase was greater than that experienced in non-bTB infected control animals. Additional analysis suggested that becoming infected with bTB shifted the parasite community to be dominated by parasites with three key traits (panel c): direct contact transmission, fast replication time and simple life cycle (rather than complex with intermediate hosts). These results suggest that bTB altered the parasite community in buffalo in particular ways, lending the ability to predict how the invasion of bTB in other host populations may affect parasite communities.
Advances Making Work More Feasible
Advances in genomic techniques have accelerated the ability to describe pathogen communities in African buffalo across a multitude of taxa from the genera to strain level (Glidden et al., Reference Glidden, Koehler and Hall2020) and explore relationships between pathogens and microbiomes (Couch et al., Reference Couch, Stagaman and Spaan2021; Sabey et al., Reference Sabey, Song and Jolles2021). Pairing genomic tools with non-invasive sampling, such as 18S sequencing of faecal samples to exhaustively characterize gastrointestinal parasite communities (e.g. Gogarten et al., Reference Gogarten, Calvignac-Spencer and Nunn2020), will continue to further our understanding of pathogen community assembly, the effect of coinfection on host fitness, and variation in pathogen communities across scales. Improvement in contact and GPS collars will support a better integration of pathogen exposure and host movement data into our understanding of pathogen community dynamics (Owen-Smith et al., Reference Owen-Smith, Hopcraft and Morrison2020). The development and reduction in cost of transcriptomics will further help to characterize complex immune responses to infection and coinfection (Sallé et al., Reference Sallé, Deiss and Marquis2020).
At the forefront of parasite community ecology, these genomic tools are starting to be used to uncover evolutionary drivers of observed coinfection patterns, helping to explain how trade-offs among evolved pathogen defences may drive responses to coinfection (Ezenwa et al., Reference Ezenwa, Budischak and Buss2021). These tools have the capacity to answer long-standing ecological questions, in a range of wildlife host–parasite systems.
Conclusions and Future Directions
Our past studies on parasite communities in African buffalo have contributed novel insights into the mechanisms by which parasites interact within their hosts, and how these interactions scale up to affect individual hosts, population-level disease dynamics, and parasite community structure. We have employed experimental and longitudinal approaches to infer causal links between infection patterns by different parasites, and have viewed coinfections both through the lens of pairwise species interactions, and at a broad community-wide scale. Along the way, we have developed and refined methods for diagnosing a range of infections in African buffalo and quantifying buffalo immune responses, other aspects of host physiology, and fitness. We have also taken advantage of new methods for analysing multivariate longitudinal data sets including interacting networks of dozens of parasite taxa. This work has set the stage for African buffalo to serve as a tractable model system for the study of disease processes in natural populations. However, our studies have raised more questions than they have answered. With new technologies and tools becoming available for data collection and analysis, there is broad scope for future disease ecological investigations in African buffalo. In particular, this model system is poised to: (i) help advance our understanding of ecological and evolutionary disease dynamics in the context of environmental change, and (ii) provide an empirical basis for evaluating whole-system impacts of disease interventions.
Current environmental changes are presenting wild animals with novel physiological challenges and assemblages of infectious organisms. In this context, mechanistic disease models are essential to providing robust predictions of disease dynamics and impacts of disease control interventions. Statistical extrapolation relies on previously observed variation to predict future conditions; however, when environments shift outside the boundaries of previously observed state space, non-linearities in host and pathogen functional responses may lead to novel infection patterns and outcomes (Kock et al., Reference Kock, Orynbayev and Robinson2018). Improvements in animal tracking technologies and metabolic loggers can provide continuous, fine-scale data on animal movement, interactions, activity levels and metabolic rate. Coupled with high-resolution environmental data streams, and non-invasive sampling for infectious diseases, these technological advances set the stage for studies connecting environmental variation with host physiological and behavioural responses (Williams et al., Reference Williams, Shipley and Rutz2021), contact patterns (Hamilton et al., Reference Hamilton, Jones and Cameron2020) and, ultimately, disease dynamics (Devan-Song, Reference Devan-Song2021). Building on this, assessing the metabolic and fitness costs of infections in natural populations becomes tractable, yielding insights on selection gradients imposed on hosts by parasites and pathogens. Complementary to this, quantitative molecular diagnostics provide detailed information on life-history variation among parasite strains and across different hosts. Faster, cheaper, deeper genetic sequencing techniques can elucidate host immunogenetic variation and parasite population genetics (Galen et al., Reference Galen, Borner and Williamson2020; Jax et al., Reference Jax, Müller and Börno2021). Taken together, these data streams promise to provide an unprecedented empirical foundation for coevolutionary studies in model natural host–pathogen systems, such as African buffalo and their parasite community.
Our previous work has uncovered the ubiquity of interactions among coinfecting parasites, and their importance in shaping disease dynamics and host fitness. However, host–microbe interactions include the full spectrum of mutualistic to parasitic interactions. Elucidating the involvement of the microbiome in host health and disease in natural populations confronted with the full gamut of environmental variability and infectious challenges is an exciting frontier in disease ecology (Leung et al., Reference Leung, Graham and Knowles2018; Williams et al., Reference Williams, Caraballo-Rodríguez and Allaband2018). Importantly, disease control interventions are likely to affect not only the specific target organisms, but also – directly or indirectly – the host’s extended infracommunity of parasites and microbiota. Previous work on successional processes in parasite communities of African buffalo has used novel analytical techniques for disentangling host and microbial factors that shape microbial infracommunity dynamics (Budischak et al., Reference Budischak, Hoberg and Abrams2016; Combrink et al., Reference Combrink, Glidden and Beechler2020; Glidden, Reference Glidden2020). However, the glimpse into parasite life-history variation and succession that we have provided is far from comprehensive. The intersection of quantitative molecular diagnostics (e.g. Glidden et al., Reference Glidden, Koehler and Hall2020; Sisson et al., Reference Sisson, Hufschmid and Jolles2017) and analytical techniques for resolving community dynamics in complex, interacting species networks (e.g. Sugihara et al., Reference Sugihara, May and Ye2012) as applied in Glidden (Reference Glidden2020) allows causal inferences to be drawn from observational parasite community data sets. This places a much broader understanding of parasite community responses to perturbations – such as disease control interventions, pathogen invasions or environmental change – within reach.
Overall, the foundational work on parasite interactions and community dynamics in African buffalo we describe in this chapter helps sets the stage for future studies in this model system addressing what is one of the central challenges in disease ecology: how to predict and mitigate infectious disease threats during a time of unprecedented, rapid environmental change.
Introduction
The African buffalo (Syncerus caffer) has historically been maligned in African colonial and post-colonial veterinary and livestock communities because of its reputation for being maintenance hosts for several infectious diseases that can impact the viability of the commercial livestock industry (Michel and Bengis, Reference Michel and Bengis2012). We provide here some historical context that justified this position, but will argue that this is an unfortunate and perhaps misguided and out-of-date narrative. The dogma perpetrated throughout colonial times that African livestock systems would naturally follow northern hemisphere production systems and disease control models has been, through retrospective social and economic analysis, challenged: ‘(…) the assumptions that the high-value/high cost option [in terms of livestock industry] is necessarily the best [in Africa] – and the one that should be striven for – and the low value/low cost [of extensive livestock] is automatically bad news are not upheld’ (Scoones et al., Reference Sintayehu, Heitkönig and Prins2010). The mandates of veterinary services are almost exclusively set up to protect intensive animal-based agriculture investments and trade. This paradigm is promoted by high-income industrialized countries, and most international trade ignores the impacts on wildlife economic opportunities and the realities of domestic livestock limitations in African countries that face restricted international trade opportunities. There is also a vested interest in maintaining this situation of perceived risk from disease, especially for the expansion of European breeds and modern intensive systems of livestock agriculture, which are often described as ‘improved, productive and efficient’ in contrast to African extensive and pastoral systems, reflecting a kind of neo-colonialism.
We argue that as the ‘new deal’ required by recognition of the Anthropocene becomes accepted, the dogma will change. Future economic, agricultural and overall development models will need to fit into the finite environmental envelop that will constrain human activities by choice or by force. There is a greater appreciation of the negative environmental, climate, health and socioeconomic externalities of intensive livestock systems, and of the need for more inclusive landscapes where biodiversity conservation and local citizens’ well-being meet and more value is put on indigenous knowledge and value systems (cf. Gordon et al., Reference Gradwell, Schutte, Van Niekerk and Roux2016). African continental ownership of future policy has been controversial for decades and may well be the final arbiter on this controversy (Artz et al., Reference Artz, Motsamai, Zacharias and Tueller1991; Prins, Reference Prins and Verwey1989).
The Development of Coexistence of African Pastoralism with Wildlife
Contemporary views on buffalo reach back to the introduction of Eurasian cattle along with the colonization of the African continent. In the following sections, we focus on eastern and southern regions of Africa where most of the ‘conflict’ is expressed, and mostly exclude western and northern regions of the continent, where cattle herds have deep histories, including truly African breeds (Prins, Reference Prins, Prins, Grootenhuis and Dolan2000). Issues between cattle and buffalo in relation to disease are less pronounced in these regions, if only because buffalo are absent in the north and there are only small, scattered buffalo populations in the west where pastoral systems dominate (Kock et al., Reference Kock, Kock, de Garine-Wichatitksy, Chardonnet, Caron, Melletti and Burton2014; Chapter 4).
Before colonization, in the seventeenth century, large areas of the southern and western regions of southern Africa were sparsely populated by the nomadic hunter-gatherer San people and nomadic farmers (Khoikhoi/Khoisan) who were probably the first livestock owners in these southern lands. The more eastern and northern areas of southern Africa were inhabited by primarily Bantu groups who were also pastoralists, owning Sanga (Nguni) cattle, sheep and goats, and also growing edible crops (Maggs, Reference Maggs, Cameron and Spies1986).
The situation in East Africa in the pre-colonial context was composed of extensive grasslands and traditional cattle owning coexisting with abundant wildlife. Wildlife behaviour and traditional livestock practices such as pastoralism and transhumance coevolved in East Africa, sharing space and participating in the engineering of open grassland savannas (Chapter 2). Bos taurus africanus or Sanga cattle is generally considered the indigenous African cattle from eastern African origins. African cattle were likely derived from complex introductions over centuries, and even from aurochs in Egyptian times from the Near East (Prins, Reference Prins, Prins, Grootenhuis and Dolan2000). Some species from North Africa are recognized to have existed beyond Egypt but are now extinct, for example Bos primigenius mauretanicus (Tikhonov, Reference Tjaronda2008). Along with hybridization between B. taurus and B. indicus or zebu cattle, a variety of breeds now constitute cattle populations in sub-Saharan Africa. These breeds coexisted with wildlife for over a thousand years and developed some resilience to infection, parasites and drought. Both pastoralism and wildlife may have benefited from each other, co-creating integrated landscapes. Wild ungulates used human-occupied areas to avoid predation by wild carnivores or, in the case of smaller antelopes, to benefit from areas grazed by cattle, feeding off early shoots after heavy cattle grazing (Augustine et al., Reference Asibey2011; Georgiadis et al., Reference Georgiadis, Ihwagi, Olwero and Romanach2007; Odadi et al., Reference Odadi, Karachi, Abdulrazak and Young2011). In the case of the hirola antelope (Beatragus hunteri), the most endangered artiodactyl in Africa today, its survival in a narrow range in Kenya was closely linked to local pastoralists. Hirola, having become extinct elsewhere apart from a small part of Kenya, often concentrate, feeding around nutrient-rich old boma sites and short grasses established by livestock grazing pressure where predators are persecuted. Hence, they benefited inadvertently from traditional pastoralism while indigenous people considered the presence of hirola as a good sign for their cattle (Andanje, Reference Andanje2002). The relationship with wildlife was used to predict resource availability (tracking movements) and as a source of culture and food when necessary (Lankester and Davis, Reference Lankester and Davis2016). Presently, with the wide availability of guns, rifles and other weaponry in the hands of pastoralists, this has changed, and the hirola is now next-to-extinct and features on the IUCN Red List.
Archaeological data indicate that diseases at the wildlife/livestock interface may also have been an important component of this interaction. Even with indigenous breeds of cattle, the establishment of pastoralism in some African ecosystems was constrained by wildlife and vector-mediated diseases, such as tick-borne diseases (e.g. East Coast fever, ECF), trypanosomiasis and malignant catarrhal fever (MCF). Practices such as fire, bush-clearing and contact avoidance, respectively, have taken time to evolve to counter sanitary threats and allow pastoralism to colonize new landscapes (Gifford-Gonzalez, Reference Glover, Macfarlane and Bengis2000). Most of Africa’s livestock farmers practiced (and still do) unfenced extensive systems with indigenous breeds of cattle (historical hybrid B. indicus and B. taurus africanus). These breeds and systems have proven to be more sustainable and resilient to infectious diseases, parasites, heat and droughts when compared to most B. taurus breeds and livestock systems imported from Europe (Mattioli et al., Reference Mattioli, Pandey, Murray and Fitzpatrick2000; Morris, Reference Morris2007). Under conditions of widespread vectors and pathogens in Africa, acquired resistance enables greater sustainability of traditional livestock keeping and resilience in the face of epidemic and endemic diseases. Little control is practiced, and fencing is very limited in its use in African rangeland, mostly to separate a few historical ranches from open range. Most of the separation between buffalo and African cattle herds derives from behavioural determinants, mostly human aggression and use of dogs for reasons other than disease, with an avoidance response from buffalo herds leading to their predominance in protected areas where humans and domestic animals are mostly excluded.
Re-Drawing of the African Landscape – Colonization and De-Colonization – Emergence of Production-Oriented Livestock Systems in Africa
Southern Africa was one of the earliest subregions to be colonized by European settlers, which took place in the seventeenth and eighteenth centuries. The settlers landing in the southern Cape were European with a drive towards settled agriculture and land tenure, which was not part of indigenous tribal cultures. These moves led to major conflicts not only between different European cultures but with several tribes over land and resources. This territorial expansionism by the settlers accelerated in the nineteenth century and culminated in the forming of several nation-states. These included the Boer republics in South Africa and periods of British sovereignty over large areas of southern Africa up to the miombo belts and forests of East and Central Africa, as well as the German colonization of South West Africa (Namibia) and East Africa (Tanganyika, Ruanda-Urundi). In addition, around the end of the nineteenth century, serious colonization of East Africa by the Germans and British happened with Tanganyika ultimately falling under British rule on behalf of the League of Nations.
During these territorial expansion periods, settlers, European hunters, war and disease all eventually had major impacts on wildlife populations. The settlers were heavily reliant on hunting to supply their daily protein needs, and there are many historical accounts of wild herds stretching from ‘horizon to horizon’ (e.g. Beard, Reference Beard1977; Prins and De Jong, Reference Prins, de Jong, Bond, Kiffner and Lee2022). There was also a large amount of commercial hunting for dried meat and hides as well as sport hunting for trophies, the slaughter continuing unabated as though the resource was limitless and infinite. These practices were also used to clear land to make space for grazing domestic stock, with wildlife numbers suffering through depredation and competition. In South Africa, the kwagga (Equus quagga), Cape lion (Panthera leo melanochaita) and bluebuck (Hippotragus leucophaeus) were driven to extinction, and the bontebok (Damaliscus dorcas dorcas), white rhinoceros (Ceratotherium simum) and Cape mountain zebra (Equus zebra) were pushed to the brink. Countless animals were killed to feed trading safaris, and colonial armies also were fed meat from game. Lands were cleared of wildlife species to create space for white settlers and local farmers alike, as in Kenya (Adamson, Reference Adamson1968, pp. 97 ff, 120) and many other places. The total number of African elephants (Loxodonta africana) killed by elephant control officers in East and southern Africa may perhaps be equal to the number that was poached for their ivory. The onslaught on Kenyan wildlife during World War II is jaw-dropping, where herds of game were used as targets to represent enemy troops and elephant herds were even bombed (Prins and De Jong, Reference Prins, de Jong, Bond, Kiffner and Lee2022).
In southern Africa, the power lay with cattle keeping and agriculture communities during colonization, while National Parks (NPs) were seen as the only way to address perceptions of irrational pastoralism (Lankester and Davis, Reference Lankester and Davis2016). Ironically, the strong militarization of protected areas, including the post-independence exclusion of people from traditional lands and even the banning of hunting (both sport and traditional), generated animosity which may even have laid the foundations for the poaching of elephant, rhinoceros and other species after the colonies collapsed. In each of these arenas, there was inevitably conflict. For example, in southern Rhodesia, wildlife were culled extensively to create buffer zones between wildlife-rich areas and colonial farmer production areas for various reasons (Mutwira, Reference Mutwira1989), and to target the preferred hosts of tsetse flies in order to reduce tsetse-infested areas and the occurrence of trypanosomiasis (e.g. Zululand; Andersson and Cumming, Reference Anderson, Doughty, Anderson and Paling2014).
With colonization, pastoralism was also catastrophically reduced through the introduction of contagious bovine pleuropneumonia, followed by the great rinderpest pandemic (the Masai–Maa cultural group was reduced by two-thirds: Prins and De Jong, Reference Prins, de Jong, Bond, Kiffner and Lee2022; Box 12.1). Some wildlife species also suffered huge losses in East and southern Africa, deregulating African savannas’ trajectories in the following decades with alteration of the habitat (e.g. bush encroachment; Holdo et al., Reference Holdo, Sinclair and Dobson2009) and wildlife diversity and abundance (reduction then increase following the space liberated by human populations). The phylogenetic relationship between African buffalo and cattle, although quite distant (Chapter 2), leads to shared pathogens and led to the frequent accusations of the buffalo being a reservoir of key livestock diseases during colonial and post-colonial eras. The buffalo became synonymous with the early concept of the so-called wildlife–livestock interface in Africa (Kock, Reference Kock, Barrett, Pastoret and Taylor2006), showing the buffalo’s prominent role in several diseases (Chapter 9). During colonial times, the epidemiology was poorly understood and quantified, and most of the evidence was derived from a few human-altered ecosystems, mainly in southern Africa. At least in this region, the negative perception of wildlife and diseases led to clear segregation of land uses dedicated to livestock production and the separation of domestic and wild ecosystems. New diseases and pressure from white colonialists forced traditional pastoralists and their livestock into newly emerging tsetse fly (Glossina spp.) belts (Prins and De Jong, Reference Prins, de Jong, Bond, Kiffner and Lee2022). In East Africa, this was less of an issue because local livestock and forestry, for instance, could be combined (Brasnett et al., Reference Brasnett, Richmond and Dimbleby1948). This divergence between concepts of ‘modern’ livestock agriculture and traditional pastoralism, between the south and the north, took on sociocultural and political dimensions. During colonial or European rule, a narrative against traditional local livestock keeping in Africa developed, and this has persisted to some extent, frequently justified by the disease paradigm and from where the power in control policies lies, which is mostly within industrialized western nations. This was understandable given that epidemic and vector-borne livestock diseases were a major constraint to the expansion of European livestock systems in southern Africa (e.g. Gunn, Reference Ferguson and Hanks1932), while in the east and west of the continent, the pastoral systems thrived. Attempts at ranching cattle gradually declined over the twentieth century in East Africa, with large landholdings reverting successfully to wildlife–cattle integrated management with meat and tourism activities and, sometimes, communal ownership (NRT, 2020).
Box 12.1 Impact of the Great African Plague Rinderpest on Livestock Development
A major event that influenced agricultural thinking was the emergence of novel pathogens exotic to Africa, for example, rinderpest and contagious bovine pleuropneumonia or CBPP (Chapter 9). The great pandemic of the late nineteenth century caused by Rinderpest (a morbillivirus) killed almost all cattle it infected and wiped out a large proportion of the indigenous wildlife herds from North to South Africa, resulting in huge epidemics in buffalo with massive mortality. In many cases, only small relict populations of some species survived in remote pockets or were entirely extirpated from their former ranges. Impacts were seen on some keystone species including migrating East African wildebeest (Connochaetes taurinus). This had major impacts on the scale of migration and habitat with a transformation in vegetation types and distribution (Holdo et al., Reference Holdo, Sinclair and Dobson2009).
Veterinary services were launched at about the same time as colonial administration became established, with the task of disease control to support the further development of livestock systems. Ironically, the loss of indigenous breeds made way for the colonists to import European breeds and hybrids favoured for their high potential for milk and meat production. These colonial cattle herds, with their innate vulnerabilities, soon came into conflict with buffalo in southern Africa.
These changes were reversed, with the elimination of the virus (officially in 2011) proving a strong disease and ecology relationship, uncommonly proven.
Positively, the colonial era inspired protection of game and areas of land in law. However, as royalty had given hunting rights exclusively to the wealthy in Europe, colonialists discriminated against local people and limited people of pastoral communities’ access, making wildlife a preserve of the rich behind a conservation banner. People and animals were negotiated or shifted away and excluded from extensive productive rangelands, and this has persisted to this day.
The pattern of wildlife decline was repeated in eastern and Central Africa during the period of decolonization from the mid-twentieth century. Indigenous communities and rebel armies slaughtered game in the transition periods, partly during conflict for food, and to push back against colonial masters, conducting revenge killings after years of exclusion and to avoid future restrictions by eliminating game. However, some positive post-colonial developments based on the colonial systems should be noted. For example, in Kenya, these included much-debated bans on hunting (Anon., 1977) in attempts to slow the decline in wildlife; improved protection agencies; and eradication of rinderpest, a big killer of buffalo (Kock, Reference Kock, Barrett, Pastoret and Taylor2006). These measures appear to have stabilized buffalo numbers, at least in Kenya, over the last three decades (Grunblatt et al., Reference Guerrini, Pfukenyi and Etter1996; KWS, 2021). Ironically, after the end of the colonial era, the same colonial model of disease management was adopted by emerging states with identical results. For the natural ecologies in these areas to recover, old paradigms had to be overturned or remain to be challenged. Interestingly, in the fields of human health and global health, this theme has also been gathering momentum as shackles remain on poor countries and a few high-income countries dictate the human health and health industry agenda (Büyüm et al., Reference Büyüm, Kenney and Koris2020).
Current Situation at The Interface, The Burden of History and the Weight of Changes
Today, many new pressures, including climate change, human population growth, associated buffalo–cattle–human conflicts (Matseketsa et al., Reference Martínez-Avilés, Iglesias and De La Torre2019) and agriculture (Prins and De Jong, Reference Prins, de Jong, Bond, Kiffner and Lee2022) are reducing the viability of buffalo populations, now highly dependent on protection. In much of sub-Saharan Africa, with under-investment from abroad, land-use pressure is mounting with heavy investment in extractive industries, including forestry, and mining, while the human population is growing at a particularly high rate. This human population impact on land is, to some extent, compensated by urbanization, but demand for food continues to grow. As a result, the lands that were designated for wildlife and pastoralism are being encroached on and put under increasing pressure. Increasing populations of livestock, more or less replacing wild ungulates, have been documented in some countries and regions such as Ethiopia (Gebretsadik, Reference Gifford-Gonzalez2016), and countries with strong wildlife economies like Kenya have also been affected, but at a slower rate (Ogutu et al., Reference Ogutu, Piepho and Said2016). Political power lies in the hands of urban communities and agriculturalists, while pastoralists are weakly represented in government. These communities have historically been persecuted and discriminated against through land-use policy that removes key resource areas from their control. Some of these conflicts, especially in West and Central Africa, remain serious and violent, with recent examples within the Fulani (a.k.a. Peul) pastoralists community around the Lake Chad basin. Today, there is an East and Central African model, where open rangeland remains available and true pastoralism and transhumance are still practiced, and a southern African model, where land tenure has dominated the scene for the past 200 years, and little ‘open’ rangeland still exists.
The ‘trade sensitivity’ around the main diseases impacting livestock production that were prominent in Europe at the time of colonization was simply transplanted into African colonial systems (see, for instance, Empire Marketing Board, 1930). In some regions of sub-Saharan Africa, disease in wildlife is still seen by animal health agencies as a significant barrier to agricultural development. However, we posit, that is mostly a result of residual dogma and supporting narrow policies that benefit the main agribusinesses trading in a globalized world. Veterinary fencing, with a primary role to separate buffalo from cattle populations, has been used extensively across southern Africa (Chigwenhese et al., Reference Channumsin, Ciosi and Masiga2016; Ferguson and Hanks, Reference Ferguson, Cleaveland and Haydon2010), but is cropping up in Europe too (like the wild boar fence separating Denmark from Germany) and has been in place in Australia since the end of the 1800s. Even until the present day, the government veterinary services of South Africa, Zimbabwe, Botswana and Namibia all practice so-called ‘hard edge’ disease control measures, which include barrier fences such as the Kruger NP (KNP) western and southern boundary fence (restricting contact with livestock in South Africa – while the eastern Mozambique fence is only partially closed), the Ngamiland buffalo fence (partly separating the Okavango Delta from livestock areas in Botswana) and the Namibian veterinary cordon fence to prevent contact between commercial livestock and wildlife, particularly buffalo. In Zimbabwe, 55 per cent of the buffalo population is fenced today, and this policy is sometimes supported by conservation non-governmental organizations (NGOs) sharing an interest in preventing cattle and people from entering the protected areas and wildlife from getting out. A similar cordon line exists between Western Zambia and Angola. These veterinary measures clearly separate commercial livestock production that is protected for their markets versus wildlife systems and small-scale livestock production imprisoned in the fenced areas and unable to be marketed. Indeed, Botswana’s fences protect blocks that are designated for commercial trade with a foreign region (European Union) and certainly constrain local production and compromise local food security, with devastating consequences on wildlife communities and ecosystems. To show the tenacity of such beliefs and practices, the Veterinary Cordon Fence in Namibia was enacted by the German Reichstag in 1905, and still discriminates between livestock producers from beyond the fence and those ‘on the right side’ of it (e.g. Miescher, Reference Miescher2012; Tjaronda, Reference Thomson, Bastos, Coetzer and Tustin2008). In addition, certain designated geographical control zones have been declared for diseases such as foot and mouth disease (FMD), African swine fever and corridor disease (buffalo-associated theileriosis) in South Africa. As in Botswana, these are primarily intended to protect designated production zones exporting to high-end meat markets. Its mirror side, however, is that by framing cattle meat as coming from potentially ‘dangerous’ areas where veterinary control may be wanting, farmers in these importing countries are enabled to prevent competing meat from coming to ‘their’ market (Robinson, Reference Robinson2017; Whittington et al., Reference Whittington, Donat and Weber2019). This fencing policy, mostly imposed by the state on local stakeholders is costly, opposed by pastoralists and accepted by commercial livestock and crop farmers for obvious reasons. In all but a few cases (UNEP, 2011), fences are detrimental to wildlife movements and conservation, and they require significant maintenance (De Jong et al., Reference de Garine-Wichatitsky, Caron and Gomo2020; Gadd, Reference Gebretsadik2012). In the absence of good maintenance, fencing deteriorates and becomes porous (Chigwenhese et al., Reference Channumsin, Ciosi and Masiga2016).
However, to some extent fencing for disease control and for reducing wildlife conflict with people and agriculture has taken hold in much of the continent. In East Africa, arguments against this approach seem to have helped to slow any progression down this path for reasons of disease control (Kock, Reference Kock, Ferguson and Hanks2010). In many situations, with open interfaces between protected areas and communal land, buffalo’s natural and adaptive avoidance of cattle reduces the risk of disease spillover, and these systems show high tolerance to infection without many diseases expressed (Caron et al., Reference Caron, Cornélis and Foggin2016; Meunier et al., Reference Meunier, Sebulime, White and Kock2017; Valls-Fox et al., Reference Valls-Fox, Chamaillé-Jammes and de Garine-Wichatitsky2018). In times of drought, contact rates can change dramatically and disease epidemics are more likely to occur (Bengis et al., Reference Bengis, Kock and Fischer2002; Kock, Reference Kock, Osofsky, Cleaveland and Karesh2005). A severe drought in Kenya from 1993 to 1994 probably precipitated the large rinderpest outbreak in the 1990s in East Africa (Kock et al., Reference Kock, Wambua, Mwanzia and Wamwayi1999, Reference Kock, Wamwayi and Rossiter2006), killing 60 per cent of buffalo in the Tsavo National Park ecosystem. However, this drought killed some 70 per cent of the buffalo in the Masai Mara even before the disease arrived (Dublin and Ogutu, Reference Dublin and Ogutu2015). In East Africa, livestock remained in open pastoral systems and coexisted with wildlife, which thrived (Homewood et al., Reference Homewood, Trench and Brockington2012), but mostly with buffalo only surviving in protected areas and buffer zones. Agropastoral, farm or ranching communities and pastoralists are known to use self-managed movement control to avoid epidemics. However, increasing densities of people and their livestock ultimately can continue to lead, in the absence of new policy, to the demise of wildlife (Prins, Reference Prins1992; Prins and de Jong, Reference Prins, de Jong, Bond, Kiffner and Lee2022). This is not inevitable; for example, more integrated developments in pastoral land use, such as in Kenya, have led to remarkable overall stability in wildlife populations over decades, despite rapid development, human population expansion and declines of wildlife on state lands (KWS, 2021).
When there is insistence on disease elimination rather than control in livestock, the interface becomes more threatening. De Vos et al. (Reference De Vos and van Niekerk2016) clearly described, for example, the challenge in South Africa of perceptions around certain species and diseases stating,
The majority of endemic pathogens found in protected areas do not kill large numbers of wild animals or infect many people, and may even play valuable ecological roles; but occasional disease outbreaks and mortalities can have a large impact on public perceptions and disease management, potentially making protected areas unviable in one or more of their stated aims. Neighbouring landowners also have a significant impact on park management decisions. The indirect effects triggered by disease in the human social and economic components of protected areas and surrounding landscapes may ultimately have a greater influence on protected area resilience than the direct ecological perturbations caused by disease.
In more extensive pastoral systems, wildlife and livestock remain integrated to some degree, with designated protected areas allowing the survival of core buffalo populations. The protected area models adopted in West and Central Africa, with core protected areas surrounded by buffer zones with limited human activities (e.g. game hunting, some pastoral activities) offered management of the buffalo/cattle interface that has allowed the survival of core buffalo populations, even if isolated (Bauer et al., Reference Bauer, Chardonnet and Scholte2020), as long as there was no security crisis. That system has subsequently collapsed in many places in West and Central Africa (Scholte et al., Reference Scholte, Pays and Adam2021), and perhaps only timber concessions and privately managed reserves appear to maintain buffalo (Chapter 4). Even though open systems have allowed wildlife to thrive, buffalo are not tolerated by pastoral livestock owners due to the aggression sometimes shown by buffalo to pastoralists and direct competition for water and grazing. This has led to the virtual extirpation of buffalo from some communal lands (Metzger et al., Reference Metzger, Sinclair, Hilborn, Hopcraft and Mduma2010). There are a few exceptions with forest buffalo (Syncerus caffer nanus) and some savanna buffalo in forested areas such as Boni Dodori in Kenya, where large populations >10,000 share habitat with hunter-gatherer/small-scale cropping communities (Chapter 4). A similar peaceful coexistence can be seen in along the Kazinga Channel between Lakes Edward and George in Uganda (Kock, personal observation).
Where a wildlife economy dominates as a source of foreign exchange revenue over agriculture, such as in Kenya and Tanzania, the political establishment lends a more sympathetic ear. In addition, and perhaps as a result, all attempts at draconian veterinary measures detrimental to the wildlife and pastoral economy have never been applied successfully, even if policies exist on paper. A sustainable balance is often achieved, allowing for livestock keeping and healthy wildlife populations to be conserved and contributing to tourism and the economy. Increasingly this tourism industry is locally owned and beneficial to indigenous communities (Mureithi et al., Reference Mureithi, Verdoodt, Njoka, Olesarioyo, Van Ranstithi, Kideghesho and Rija2019; Tyrrell et al., Reference Tyrrell, Russell and Western2017; Western et al., Reference Western, Tyrrell and Brehony2020). While in the south of the continent, where livestock owners were well connected politically and largely dominated the land-use arguments in favour of agriculture for over a century (Munangándu et al., Reference Munangándu, Siamudaala and Mambota2006), this has been reversed to some extent more recently, with an expansion of wildlife ranching and conservancies (Chapter 13). In many of these ranches and conservancies in South Africa, integrated farming with livestock and wildlife now takes place, but with the exclusion of buffalo and large predators. Legislation dictates that buffalo and cattle may not be kept on the same property.
In addition to these influences, a failure to invest in local communities around wildlife protected areas brings more pressure. Estimates of locally shared revenue from conservation areas like the Serengeti are only 5 per cent of total annual income and only go to a few households, with the majority of beneficiaries being a distant private sector and government exchequers (Homewood et al., Reference Homewood, Trench and Brockington2012; Lankester and Davis, Reference Lankester and Davis2016). In Zimbabwe, where Operation CAMPFIRE first resulted in much higher revenue sharing with local communities, this community benefit fell to only a few euros per year after the CAMPFIRE strategy was ‘invaded’ by local politicians and bureaucrats (Poshiwa et al., Reference Poshiwa, Groeneveld and Heitkönig2013a, Reference Poshiwa, Groeneveld and Heitkonig2013b). We are not judging what was or is right or wrong in this debate on ‘human versus biodiversity rights’, but are trying to present the different perspectives and historical precedence around disease which may explain past and current actions.
Recovery of African Pastoralism and Wildlife in Africa – Is This Possible?
With ongoing climate change and continuing human population growth, as resources decline and drylands increase, the use of available water from rivers and wetlands will probably increase the likelihood of buffalo and cattle meeting. Increased grazing pressure may result in ecological disturbance and degradation of natural resources. If this progresses, the natural disease regulation benefits of the ecosystems may begin to decline and vector–host–pathogen dynamics may be disturbed, which will impact livestock more. Eventually, wildlife may also suffer as malnutrition and stress erode even their resilience to disease, and whole ecosystems may begin to decline with population crashes. As buffalo are removed from pastoral or agriculturally designated lands, they are still frequently blamed as a source of diseases for livestock kept by communities surrounding the conserved areas where buffalo are mostly found. In addition, the ecological consequences of agriculture, ranching and overall degradation of fenced land, especially with high stocking rates, create poor conditions for ungulates and increased vulnerabilities to disease irrespective of the presence of carrier animals peripherally or in the parks (Glover et al., Reference Gordon, Prins and Squire2020; Kinne et al., Reference Kinne, Kreutzer, Kreutzer, Wernery and Wohlsein2010). However, the conflict remains high in livestock keepers’ minds. As populations of cattle grow, the domestic animals themselves become more epidemiologically significant, through a mere numerical relationship, and become the main carriers of diseases and a preferred food source for disease vectors (Channumsin et al., Reference Condy and Vickers2019; Clausen et al., Reference Chigwenhese, Murwira and Zengeya1998). This can change the epidemiological dynamics of pathogens locally, shifting the role of wild and domestic species, and can drive the further spread of disease within the domestic population, the community of wild and domestic ungulates, which may include spillback to wildlife.
With today’s improved epidemiological knowledge, better diagnostic tests and better livestock vaccines, it is hoped that African endemic disease control can become less conflictual and more environmentally friendly. The movement of diseases between wildlife and livestock is in fact bi-directional. With dwindling wildlife numbers in many countries (especially in West and Central Africa: Chapter 4), wild animals can also be threatened by persistent livestock disease spillover (e.g. bovine tuberculosis, peste des petits ruminants and brucellosis) to naive and sometimes critically endangered wildlife (Pruvot et al., Reference Pruvot, Fine and Hollinger2020; Shury et al., Reference Shiferaw, Abditcho, Gopilo and Laurenson2015; Viggers et al., Reference Viggers, Lindenmayer and Spratt1993; White et al., Reference White, Wallen and Geremia2011; Chapter 9).
More recently, changing views on livestock management and values of wildlife have resulted in the fading away of earlier red lines on diseases such as FMD (Ferguson et al., Reference Gadd, Somers and Hayward2013; Weaver et al., Reference Weaver, Domenech, Thiermann and Karesh2013). Strengthening wildlife-based economies in Africa, and innovative thinking around integrative management of wildlife and livestock and the rangelands in which they coexist, are increasing environmentally friendly land uses (Ferguson et al., Reference Gadd, Somers and Hayward2013). Development of softer disease policies such as the use of commodity-based trade (CBT) to circumvent FMD trade restrictions renders FMD elimination an obsolete goal (Thomson et al., Reference Tikhonov2013) even if currently its acceptance remains slow. Nevertheless, there are now increasing opportunities for trade without disease control burdens. The emphasis on intensive husbandry of livestock with production and profit as the main goal is shifting towards more sustainability in food systems. Other benefits of mixed rangeland management include climate change mitigation and reduction in disease control costs. Perhaps the ultimate arbiter of future livestock systems will be the concerns over their role in biodiversity loss, as competitors for food crops which might otherwise be used for humans and climate change ramifications. A shift from animal- to plant-based diets is gaining momentum in many countries in Africa where meat consumption remains low per capita compared to other continents while biodiversity remains high (Figure 12.1).

Figure 12.1 Meat supply per person in 2017.
In South Africa, a buffalo production model emerged in the 1990s and has evolved to this today with controversial outcomes (Box 12.2). The more visionary wildlife ranching and community-based natural resource management (CBNRM) practiced in Namibia and Zimbabwe since the 1970s, utilizing mainly the extensive and relatively free-range systems and conservancies, has been important in bringing communities on board. Kenya and Tanzania have taken strides in recent decades, with integrated pastoral livestock and wildlife ecosystems such as the Northern Rangeland Trust and Ngorongoro Conservation Area, respectively. However, upheavals and violence in the latter area in 2022 may throw another light on the success of this narrative (Kihwele et al., Reference Kihwele, Veldhuis and Loishooki2021; ROAPE, 2022). In regard to such initiatives, South Africa is lagging behind, with Uganda and Ethiopia and countries in West Africa (with large and small buffalo populations) even more so.
Box 12.2 Buffalo as a Production Animal in South Africa: A Case Study
The uses of buffalo as a production animal or for trophy hunting are covered in Chapters 13 and 16. However, it is pertinent to showcase the intensification of buffalo production (semi-intensive and intensive production systems: Chapter 13), separated from cattle production but along the modern northern hemisphere economic model (privatization, compartmentalization, commodification of nature for capitalist markets) within a sector sometimes disconnected from nature.
In South Africa, KNP authorities developed a project between 1996 and 2006 in response to concerns about invasive bovine tuberculosis (bTB) and the unique genetics of their infected buffalo population, as well as commercial interests (Bengis and Grobler, Reference Bengis and Grobler2000). The objective was to breed specific pathogen-free (SPF) buffalo calves from infected parent stock. Approximately 460 SPF buffalo calves free of FMD, theileriosis, bTB and bovine brucellosis were produced during the lifetime of the Kruger project. Many more so-called SPF calves were also produced from infected parent stock in private facilities within the FMD control zone. The offspring of these original buffalo were translocated to other NPs that did not have buffalo, which was in itself a major conservation goal, and today, Pilanesberg, Vaalbos, Marikele, Mountain Zebra and Mokala NPs all have viable, relatively free-ranging populations of Kruger buffalo in multi-species and extensive systems. Some SPF offspring were also supplied to private wildlife ranches throughout South Africa. Today, these privately owned SPF buffalo are being kept under intensive, semi-intensive and extensive conditions. Under more ‘controlled’ and intensive ranching conditions, population health appears to deteriorate and any resilience benefit of wildlife over cattle is diminished. Diseases in intensively managed captive buffalo further show this tendency for a shift in pathogenicity when animals are removed from their natural ecosystems with, for example, FMD expressed through weight loss and lymphadenopathy (Vosloo et al., Reference Vosloo, de Klerk and Boshoff2007), bTB and Rift Valley fever-associated morbidity and mortality also expressed under certain conditions (Beechler et al., Reference Beechler, Bengis and Swanepoel2015). With these more intensive systems, endo- and ectoparasite control also became important. In addition, some SPF buffalo raised under conditions of minimal exposure to disease vectors have actually died from theileriosis, MCF and even heartwater after significant tick exposure or contact with wildebeest or sheep. These are diseases to which buffalo are normally totally resistant.
The fact that these SPF buffalo are now present on wildlife ranches in all nine provinces of South Africa has been problematic for the State Veterinary Services. Legislation requires that all farms that have buffalo must be registered, and any buffalo movement from one property to another requires animals to be retested for all four diseases. There is also concern over veterinary management options should an outbreak of any of these dreaded diseases occur in this diffuse privately owned population. This concern has continued to lead to discrimination against buffalo in the last decades; for example, SPF buffalo breeding project expansion in South Africa has been curtailed by the Veterinary Department. As the evidence shows, replacing a commercial domestic cattle model with a wildlife ranch model may not work as any intensification and interruption of natural ecological processes is fraught with problems and disease is clearly one.
Some of these trends relate to the veterinary controls and historical separation of animals and subsequent commercialization of wildlife species. In this regard, the difference between extensive versus more intensive forms of buffalo production needs to be appreciated. While extensive systems can certainly achieve important conservation goals, in contrast, the more intensive forms of production, despite claiming to be contributing to species conservation, are not recognized by conservation bodies such as IUCN. The recent legal change in South Africa where some of these species may be listed as farming animals for intensive commercialization further demonstrates this shift and effectively disconnects these populations from nature. Animals raised in these intensive production systems should not be used for conservation purposes, such as reintroductions or reinforcements of natural populations, due to the risk of introduction of production diseases or of animals which are ‘disease-free’ becoming exposed to natural disease cycles. In addition, a potential genetic shift and/or altered production genes may be deleterious to natural ecologies (cf. wild boar Sus scrofa; Martínez-Avilés et al., Reference Martínez-Avilés, Iglesias and De La Torre2020). Land uses in which SPF buffalo are produced intensively and artificially selected should not be connected to natural ecosystems or protected areas for conservation purposes.
Under several proposed future alternative development scenarios, if land is released from animal production for rewilding, disease epidemics are likely to decrease without abundant domestic animal host populations. Historic concerns about wildlife as disease reservoirs will dissipate, resource competition will decline and wildlife-based economic opportunities will arise.
Are Buffalo and the Diseases They Carry Still a Concern in the Modern African Landscape?
As observed, the diseases which have been much thought of in the context of buffalo and cattle are African strains of FMD, corridor disease (theileriosis), bTB, brucellosis, trypanosomiasis, heartwater and Rift Valley fever (Michel and Bengis, Reference Michel and Bengis2012; Chapter 9). African buffalo are believed to serve as maintenance or incidental hosts and amplifiers of these cattle diseases with potential spillback (Musoke et al., Reference Musoke, Hlokwe, Marcotty, du Plessis and Michel2015). However, there is relatively little supportive evidence for spillback happening, and new evidence is challenging long-held assumptions. In truth, cross-species infections are rarely documented or confirmed, but epidemics can occur, especially where there is a policy on disease elimination in livestock, and a hard boundary between disease-free and infected populations created by fencing. The following paragraphs provide examples to illustrate the trends.
There is no doubt that FMD SAT strains are maintained by buffalo, and they may represent the original coevolved host (Anderson et al., Reference Andersson, Cumming, Andersson, de Garine-Wichatitsky, Cumming, Dzingirai and Giller1979; Thomson and Bastos, Reference Thomson, Penrith and Atkinson2004). However, as with many ‘emerging’ infectious diseases, confirming origins retrospectively is nearly impossible. Cattle that are FMD-free can be at risk of a breakdown in status at any interface with buffalo and other carriers of the virus (Guerrini et al., Reference Gunn2019; Hargreaves et al., Reference Hargreaves, Foggin and Anderson2004; Miguel et al., Reference Miguel, Grosbois and Caron2013). However, even this strong narrative of buffalo being the original sole ‘source’ of SAT strains of FMD in Africa is now in question. Buffalo may perhaps have been so historically, but more recent evidence in certain regions of the continent shows that cattle can also maintain cattle-adapted SAT strains for extended periods (Omondi et al., Reference Omondi, Gakuya and Arzt2020; Wekesa et al., Reference Wekesa, Sangula and Belsham2015). Not all cattle outbreaks with SAT viruses might have been from buffalo, but in several southern African countries, cattle outbreaks have been shown to be caused mainly by buffalo isolates. Many of these buffalo isolates that are regularly mutating have subsequently been incorporated into cattle vaccines.
Some vector-borne diseases – for example, the tick-borne disease theileriosis and heartwater – can cause very high mortality (up to 100 per cent) in naive African cattle (Lawrence, Reference Lawrence, Norval, Perry and Young1992; Neitz et al., Reference Neitz, Canham and Kluge1955), while certain exposed cattle populations living at extensive buffalo/cattle interfaces suffer fewer losses (Young, Reference Young, Irvin, Cunningham and Young1981). As with FMD, cattle-adapted Theileria strains have evolved and emerged, causing the diseases known as East Coast fever (ECF) and Zimbabwe theileriosis (January disease), and these diseases can circulate independently in cattle without any buffalo presence. In addition, with regards to heartwater, the development of premunity and endemic stability has resulted in fewer losses. Other vector-borne diseases can have multiple reservoir hosts. With trypanosomiasis, wildlife certainly provides reservoir hosts, some preferred by tsetse flies, such as buffalo, wild porcines, spiral-horned antelopes and elephants, but from a risk perspective, these populations can also dampen environmental infection loads away from livestock and humans, reducing disease risk and impacts (Channumsin et al., Reference Condy and Vickers2019; Clausen et al., Reference Chigwenhese, Murwira and Zengeya1998). As with the Rift Valley fever virus, buffalo are susceptible but are only one among a myriad of susceptible wild and domesticated ruminants (Swanepoel, Reference Swanepoel1976).
On the bacterial side, even if livestock-origin brucellosis and bTB have crossed the ‘species barrier’ many times, their impact on free-ranging populations of buffalo appears to be ecologically insignificant. For brucellosis, this is true even in populations where brucella spp. antibody prevalence is quite high, as in KNP, South Africa (De Vos and van Niekerk, Reference De Vos, Cumming and Cumming1969; Herr and Marshall, Reference Herr and Marshall1981; Ndengu et al., Reference Ndengu, Matope and de Garine-Wichatitsky2017) with occasional reports of disease in buffalo and spillover to other species (Condy and Vickers, Reference Clausen, Adeyemi and Bauer1972; Gradwell et al., Reference Grunblatt, Said and Wargute1977). Certainly, the observation of hygromas in older buffalo is not uncommon throughout the buffalo range. Much more has been published on bTB in buffalo, but almost exclusively focused on South Africa (but see Sintayehu et al., Reference Sintayehu, Prins, Heitkönig and de Boer2017a), where it is thought to have been introduced with cattle imports in the eighteenth and nineteenth centuries (Paine and Martinaglia, Reference Paine and Martinaglia1928). The most attention is given to the ‘compressed’ fenced or semi-fenced protected areas such as KNP and Hluluwe/Umfolozi NP (Bengis et al., Reference Bengis, Kriek and Keet1996; de Garine-Wichatitsky et al., Reference Dasmann2010), with high buffalo densities. Here, there are concerning trends with buffalo apparently suffering some disease and mortality. There is evidence that this species is also driving infection in other wildlife species such as lions, Panthera leo (Keet et al., Reference Keet, Kriek, Bengis, Grobler and Michel1996), greater kudu, Tragelaphus strepsiceros (Keet et al., Reference Keet, Kriek, Penrith, Michel and Huchzermeyer2001) and chacma baboons, Papio ursinus (Keet et al., Reference Keet, Kriek, Bengis and Michel2000). This has raised conservation concerns regarding less-populous species and predator/scavenger impacts. In more open unfenced systems in Uganda, bTB was confirmed around the 1960s and seroprevalence for bTB has been consistently high in buffalo over the intervening years, yet the disease is rarely reported (Guilbride et al., Reference Guilbride, Rollison and McAnulty1963; Meunier et al., Reference Meunier, Sebulime, White and Kock2017; Woodford, Reference Woodford1982a, Reference Woodford1982b). In Ethiopian pastoral systems, and likely in other pastoral systems too, the patterns of bTB are closely linked to human social networks (Sintayehu et al., Reference Sintayehu, Prins, Heitkönig and de Boer2017a). There are also efforts in Ethiopia to establish risk factors for cattle TB associated with wildlife (Sintayehu et al., Reference Swanepoel, Crafford and Quan2017b). These are multi-species and slowly developing diseases with a long time course before clinical expression, and they are both zoonoses, so need to be monitored.
Anthrax is another multi-species disease that occurs on most continents, and in Africa epidemics occur in wildlife and these are sometimes associated with epidemics in livestock (Mukarati et al., Reference Mukarati, Matope and de Garine-Wichatitsky2020). Epidemiologically, this spillover may well be mostly driven by insect mechanical vectors such as blowflies and biting flies and through contamination of browse, pasture or water (Bengis, Reference Bengis, Miller and Fowler2012; De Vos and Turnbull, Reference De Vos, Turnbull, Coetzer and Tustin2004; Ebedes, Reference Ebedes1976; Hugh-Jones and De Vos, Reference Hugh-Jones and De Vos2002; Prins and Weyerhauser, Reference Prins and Weyerhaeuser1987). Evidence from Ethiopia suggests some significant recorded events in wildlife occurred after a series of livestock outbreaks (Shiferaw et al., Reference Scoones, Bishi and Mapitse2002), and in the Serengeti, wildlife anthrax epidemics tend to occur during droughts, clustered around contaminated sites such as water holes/salt licks and similar locations where aggregation and mixing of species can occur (Hampson et al., Reference Hampson, Lembo and Bessell2011).
It certainly can be argued that introduced cattle diseases on the African continent have had impacts on both the cattle industry and wildlife. Indirectly, the impact on wildlife has been seen through the implementation of control measures. Fortunately, in open mixed rangelands systems, these introduced diseases and the buffalo-endemic diseases are of little consequence to wildlife populations, other than rinderpest. There is a need to re-evaluate historic and modern disease dialogues, rather than perpetuating the old narratives and the prejudice against wildlife as disease reservoirs.
Buffalo have attracted significant research, often becoming the centre of investigations, with this focus perhaps reinforcing preconceptions of their relative disease role and significance. Of 79 publications recorded in a scoping review on viruses in ungulates (Swanepoel et al., Reference Shury, Nishi, Elkin and Wobeser2021), 41 were on FMD in buffalo. This high number is most probably due to the funding available and international interest among researchers for this disease. Buffalo are overstudied without considering other species found in the same environment or the role of cattle themselves in the persistence and spread of infection. As a consequence, the roles of these other wild ungulates (e.g. greater kudu, Thompson’s gazelle [Gazella thomsonii], impala [Aepyceros melampus] or blue wildebeest [Connochaetes taurinus]) are relatively unknown despite evidence of their role in a few specific breakdowns and cattle epidemics historically (Weaver et al., Reference Weaver, Domenech, Thiermann and Karesh2013). In KNP, which has an endemically infected buffalo population, clinical spillover FMD has also been confirmed in impala, greater kudu, bushbuck (Tragelaphus scriptus), common warthog (Phacochoerus africanus) and giraffe (Giraffa cameleopardalis), but with relatively mild symptoms observed rarely (e.g. impala; Vosloo et al., Reference Vosloo, Thompson and Botha2009).
Towards a New Vision
In the context of disease transfer between buffalo and cattle, who is to blame? The most significant problems for wildlife and cattle stem from the introduction of exotic breeds and their diseases into Africa and the production and trade model that came with them. Models of coexistence between buffalo and African cattle breeds existed during the pre-colonial era only to be disturbed by the introduction of susceptible European breeds and their northern hemisphere pathogens during the colonial period. In addition, the endemic disease risks to introduced cattle, especially for the so-called improved breeds, are very high. The full benefits of buffalo (as a comparable bovid) in the context of the animal and land use are clear but are not being realized, except in some specific land use examples. Most buffalo populations have been reduced to small relict herds, especially in West and Central Africa. If buffalo go extinct, cattle will provide poor compensation for this highly adapted species.
On the other hand, from a disease risk perspective, the management of wildlife species such as buffalo along similar lines to livestock production makes little sense. Extensive free-ranging unfenced systems are already of proven value for harvesting, sport hunting and tourism, and as bulk grazers in maintaining ecosystem’s integrity and function. The history of the development of both cattle and wildlife managed systems in Africa and trends in associated disease problems provide abundant evidence for this conclusion. The resilience and health of species are highly connected to the ecological resilience of the systems in which they have coevolved. Therefore, it is likely that community-based (pastoral) systems that can be mixed, rather than agribusiness-driven fenced monoculture (wild or domestic), are a route to sustainable tourism and animal-based food production systems and economies in Africa. Much of African livestock and wildlife will remain more or less in open conditions for the foreseeable future, and thus these systems should be reinforced by appropriate policy and investment rather than discriminated against. This approach has the added value of ecological recovery of highly degraded landscapes from over-intensive livestock agriculture, reinforcing biodiversity conservation, supporting the delivery of natural ecosystem goods and services as well as related income streams. It could innovatively contribute to the target of 30 per cent of land protected by 2030 recently set by the High Ambition Coalition adopted by 69 countries (HAC, 2021). The ambition is high indeed, but realities on the ground suggest it will simply be too late in many countries to progress before major land-use changes and settlements have degraded habitats, especially in Central African savannas (Scholte et al., Reference Scholte, Pays and Adam2021). Climate change mitigation benefits will also accrue, while still contributing high-quality protein and food security, in a continent that has the lowest meat consumption per capita on Earth. However, to achieve this means shedding colonial legacies around land use and tenure, livestock development and animal health production systems imposed on Africa. It may require reversion to more traditional views on extensive animal use, harvesting and integrated low-cost–low-risk systems of management that are not new and were widely discussed in the twentieth century (Asibey,Reference Asibey1974; Dasmann, Reference De Jong, van Hooft and Megens1964; Ledger, Reference Ledger1964).
As a new community of scientists and veterinarians emerges across Africa with a novel vision, knowledge from the past and ideas about future agro-ecologies and mixed land use will likely come into play. What may help is that there is now a major shift in perception of animal-based food systems in the very countries that promoted intensive livestock production in Africa. The question that really matters is what do Africans think of alternate futures? Will they remain embedded in old development dogma, or will they surf on the economic and cultural opportunities offered by wildlife? Could mixed land use become a dominant policy? Already, new land management, with mixed livestock and conservation initiatives, has shown considerable success in Namibia and Kenya, building on earlier innovation in Zimbabwe under the CAMPFIRE project, which under difficult political and economic circumstances remains nascent.
New ideas and opportunities beyond conventional systems of agriculture and wildlife protected areas will undoubtedly emerge, becoming hopefully more conducive to both local economic growth, ecosystem stability, resilience and biodiversity conservation. Planetary health demands it. In this chapter, we have shown the need for a reappraisal of history and the risk in the context of buffalo and diseases of concern to the livestock industry. Much of the narrative for ‘land clearing’ of wildlife is historic and ‘blames’ buffalo for diseases such as FMD, tick-borne infections, brucellosis and bTB, which early on justified fencing and the compartmentalization of land. In many cases their roles in the epidemiology of these pathogens are tangential and no longer highly significant in evolving contexts in which cattle populations are exploding and buffalo populations are maintained or decreasing in many ecosystems.
The genetic modification of farmed animals for objectives of higher production creates breeds more susceptible to pathogens when buffalo are disease-resistant in African contexts. Putting them together does not make the buffalo the culprit. The current industrial approach may work in highly transformed temperate systems but not in Africa’s landscapes, where there is high microbial and vector diversity and a multitude of hosts. This failure of livestock intensification to develop in Africa without subsidization has led to the narrative that it is only through promoting greater separation and higher biosecurity that animal-based food economies can develop and reduce the risk of catastrophic disease epidemics in heavily invested livestock industries. This is basically underpinning policy on disease control and has been supported by unrealistic standards in disease management generated by the World Animal Health Organization (WOAH). These industries are effectively highly subsidized through international funding, and agencies are dedicated to forcing through these agricultural development agendas. Ironically, most of the benefits from these policies accrue to high-income countries that are not African, for instance through blocking access to their lucrative markets. Globally, these policies have come at a major cost to biodiversity and ecosystems in return for little more than cheap protein and high profits for agribusinesses. Africa now stands to gain substantially from shifts in diet, with reduced meat consumption and an increasing acknowledgement of the value of natural land and biodiversity. None of the externalities from, for example, climate change impacts to the loss of habitats or biodiversity are currently born by the livestock industry, although the internalization of these externalities has been called for by the Ecosystem Approach (Principle 4) under the Convention of Biological Diversity (CBD Decision COP V/6) and again under the Principles for Sustainable Use by the same (binding) Convention (CBD Decision COP VII/12). This situation will change as there is increasing pressure for accountability and determination of externalities of various industries for future sustainable development. Buffalo may well still have a bright future.