I. NATURAL GAS GENERALITIES
The United States is the world’s largest producer of natural gas. 1 As of 2015, the United States produces 28.8 trillion cubic feet (TCF) of natural gas per year. 2 The United States natural gas production has been increasing every year since 2010, 3 and this increase in production can, in part, be contributed to the increasing use of fracking and other technologies that release previously untapped natural gases in shale. 3 According to US Energy Information Administration, the natural gas consumption is expected to rise to 31.6 TCF in 2040. Reference Sieminski4 Processing of natural gas is by far the largest industrial gas separation application. Reference Baker and Lokhandwala5 Every year close to 100 trillion standard cubic feet of natural gas are used worldwide. This large demand requires an effective technology to process and purify natural gas.
Natural gas consists primarily of methane, higher alkanes, carbon dioxide, nitrogen, and hydrogen sulfide as shown in Table I. In particular CO2 and N2 decrease the heat value of the natural gas. Therefore, it is highly desirable to remove CO2 and N2 from natural gas to improve its heat content, and to avoid the erosion of pipelines from CO2 in the presence of moisture.
TABLE I. Typical natural gas composition. 6
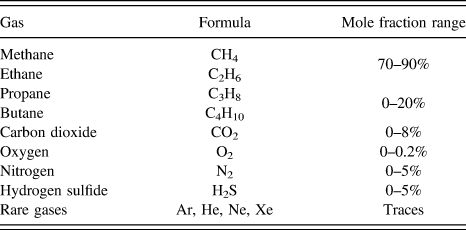
Approximately 14% of US natural gas contains >4% N2. Reference Baker and Lokhandwala5 However, most pipeline standards require that natural gas contains less than 4% inert gases. N2 dilutes the heating value of the gas, resulting in lower British thermal units. In the process of purification, it is highly desirable to maintain the gas at high pressure when explored to save recompression costs. In this respect, it is necessary to develop efficient processes to remove N2 from natural gas.
II. NATURAL GAS NITROGEN REJECTION TECHNOLOGIES
Nitrogen rejection is a challenging technical separation because of the similar molecular size of N2 (∼0.36 nm) and CH4 (∼0.38 nm). Several processes have been developed to treat natural gas to meet gas specifications. Reference Baker and Lokhandwala5 Many of these high-N2 gas streams are diluted with low-N2 gas to meet pipeline specifications. If this is not practical, an N2 removal unit is required. This unit includes cryogenic distillation, pressure swing adsorption (PSA), lean oil absorption, or membrane separation. Reference Baker and Lokhandwala5
A. Cryogenic distillation
Most of the N2 reject plants in the US apply a cryogenic distillation process. Reference Baker and Lokhandwala5 This process employs low-temperature thermodynamic distillation based on the boiling point difference between N2 (−195 °C) and CH4 (−162 °C). As the mixture cools, CH4 condensates first, allowing the two gases to be separated. Cryogenic plants are suited to large gas fields that can deliver 50–500 million standard cubic feet per day of gas for 10–20 years. Reference Baker and Lokhandwala5 The major disadvantages of this process are (a) high-energy requirement, (b) high operation cost, and (c) high tendency for blockage of process equipment.
B. Pressure swing adsorption (PSA)
In addition to cryogenic distillation process, PSA has been used to adsorb N2. Reference Rufford, Smart, Watson, Graham, Boxall, da Costa and May7 PSA is known to be one of the most economic and widespread processes for gas purification. Typically, PSA employs a zeolite absorbent or activated carbon to selectively adsorb CH4 and higher hydrocarbons. The adsorbed gases are then regenerated from the absorbent with a combination of pressure and thermal changes to give a gas free of N2. However, there are drawbacks to this process, such as (a) limited gas stream adsorption, (b) discontinuous circulation, (c) complex design of the process, and (d) difficulty in scale up.
C. Lean oil absorption
Lean oil absorption technology absorbs the CH4 away from the N2 in a heavy hydrocarbon and then regenerates the absorbed CH4 either through staged pressure reduction or through thermally driven distillation. 8 This technique uses the difference in liquid–vapor equilibrium constants between N2 and hydrocarbons to selectively absorb the gaseous hydrocarbons in the condensate at room temperature and high pressure, leaving N2 unabsorbed. The liquid fraction containing CH4 is then treated to give away N2-free gas as a product. However, this technique is a less competitive alternative with respect to cryogenic process because of its lower efficiency. Reference Carugati, Gambarotta and Pollesel9
For both PSA and lean adsorption, the resultant methane product would be at low pressure. However, typically the pipeline delivery for further use is at higher pressure and thus, the recompression could be cost prohibitive.
III. MEMBRANE TECHNOLOGY FOR MOLECULAR GAS SEPARATIONS
Separating gases through membranes offers the advantage of low-energy cost relative to the established gas separation processes, such as adsorption and distillation. During the last two decades, membrane technology has been widely used in several industrial separation applications. Reference Koros and Mahajan10,Reference Koros and Fleming11 For membrane separation, the challenge is to develop continuous and reproducible membranes with the necessary separation characteristics. The two main parameters that are used to assess membrane performance for gas separations are: permeance or permeability and separation selectivity α. Figure 1 shows the two types of membranes that can be used for N2 rejection: CH4-selective and N2-selective membranes. Reference Li, Zong, Zhou, Huang, Song, Feng, Zhou, Meyer, Yu and Carreon12
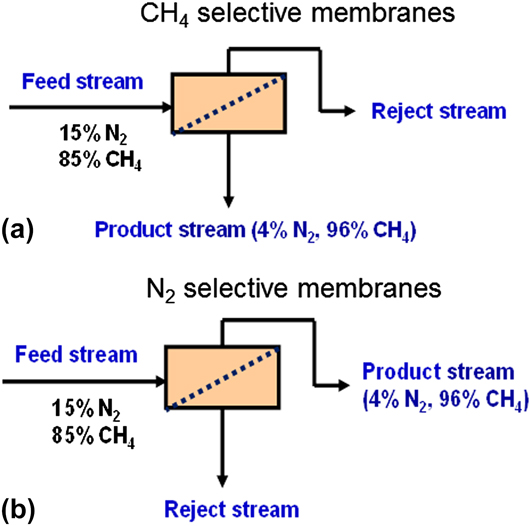
FIG. 1. Membrane configurations for N2 rejection: (a) CH4-selective membranes and (b) N2-selective membranes. Modified from Ref. Reference Li, Zong, Zhou, Huang, Song, Feng, Zhou, Meyer, Yu and Carreon12.
For CH4-selective membranes [Fig. 1(a)], CH4 is more permeable than N2 so that it is concentrated in the low-pressure permeate side and needs to be recompressed before it is passed to the pipeline. Meyer and Henson Reference Meyer and Henson13 evaluated the economics for CH4-selective membranes. Single-, two-, and three-stage systems were analyzed in their study. The estimated costs were too high to be of commercial interest for the state-of-the-art CH4-selective membranes unless the CH4/N2 selectivity could surpass 7. For N2-selective membranes, CH4 is rejected to the retentate [Fig. 1(b)], and thus there is a significant saving in recompression cost (by leaving CH4 in the high pressure side) as compared to CH4-selective membranes. However, the state-of-the-art N2-selective polymeric membranes display low N2 permeabilities, typically less than 10 barrers [1 barrer = 10−10 cm3 (STP) cm/(cm2 s cmHg)] at a selectivity of approximately 3. Polymeric membranes including PDMS can achieve higher N2 permeances but are CH4 selective. Reference Lokhandwala, Pinnau, He, Amo, DaCosta, Wijmans and Baker14 Commercial processes design and economic considerations for the removal of nitrogen from methane employing polymeric membranes are discussed elsewhere. Reference Lokhandwala, Pinnau, He, Amo, DaCosta, Wijmans and Baker14
Industrial relevant gas separations are dominated by polymeric membranes. Recently, there has been great interest in the development and application of inorganic membranes displaying molecular sieving properties. In the next sections, we review and discuss the state of the art of these molecular sieve membranes that have demonstrated the ability to effectively separate N2/CH4 gas mixtures.
IV. CARBON MOLECULAR SIEVE MEMBRANES FOR N2/CH4 SEPARATION
Carbon molecular sieve membranes (CMS) resulting from the high-temperature pyrolysis of some polymers at controlled conditions, represent a novel type of materials, which are highly appealing for molecular gas separations. Reference Rungta, Xu and Koros15–Reference Ning and Koros19 CMS consist of highly disordered sp 2-hybridized graphene-like carbon sheets, with very little long-range order (amorphous phases). Reference Wenz and Koros20 It has been suggested that these sp 2-hybridized carbon sheets align into lamellar-like structures, yielding ordered microporous structures. Reference Wenz and Koros20 Specifically, these CMS phases display open micropores connected by rigid slit-like ultramicropores to provide high sorption and diffusion coefficients. Reference Koros and Zhang21 Different from the well-defined microporous structures which characterize zeolites and metal organic frameworks, CMS exhibit distributions of micropores and ultramicropores. Reference Koros and Zhang21
Koros group reported the synthesis of a CMS membrane obtained from a controlled pyrolysis of a commercial polyimide Matrimid® 5218. Reference Ning and Koros19 During the conversion process, (polymer to CMS) scission and reorganization of polymer main chains occur, with small gas molecules released and free radicals formed. Reference Ning and Koros19 Those free radicals can form interchain or intrachain crosslinking leading to a conjugated aromatic 3D network with a graphene-like structure. With increasing temperature, these networks can form ordered structures. The packing imperfections between ordered regions can form a ‘slit-like’ pore structure, combined with a bimodal pore distribution. Therefore, the resultant CMS exhibit micropores with a size of ∼0.7–2 nm connected by molecular sieving ultramicropores with a size <0.7 nm. The separation performance of these CMS membranes was evaluated for N2/CH4 gas mixtures. The membranes displayed N2/CH4 selectivities as high as 7.7 and N2 permeability of 6.8 barrers at 24 °C, surpassing the polymer membrane upper bound line for N2/CH4. Reference Robeson22 Authors suggested Reference Ning and Koros19 that the distinctive morphology of CMS as well as the presence of ultramicropores led to high observed N2/CH4 separation selectivity.
V. ZEOLITE MEMBRANES FOR N2/CH4 SEPARATION
Zeolites are hydrated crystalline aluminosilicate minerals made from interlinked tetrahedral of alumina (AlO4) and silica (SiO4). These tetrahedrally coordinated atoms (T-atoms) are coordinated to four oxygen atoms and linked to other T-atoms by sharing oxygen with a neighboring T-atom tetrahedron. Reference Xu, Pang, Yu, Huo and Chen23 Zeolites are microporous crystalline solids with a relatively open, three-dimensional (3D) crystal structure built from the elements aluminum, oxygen, and silicon, with alkali or alkaline-earth metals plus water molecules trapped in the gaps between them. Reference Xu, Pang, Yu, Huo and Chen23 Zeolites exhibit very uniform pore size distribution, high specific surface area, high porosity, and tunable variable chemical composition making them highly attractive for challenging gas molecular separations.
Two types of zeolite compositions in membrane form have been reported to separate N2/CH4 mixtures: zeolite SSZ-13 and zeolite SAPO-34. SSZ-13 zeolite displays chabazite topology. Its framework contains a tridimensional pore system made up of 0.38 nm, eight-membered oxygen rings. Its pore size close to the kinetic diameter of CH4, makes zeolite SSZ-13 suitable for potential molecular sieving effect (for N2/CH4 gas mixtures). Wu et al. synthesized SSZ-13 zeolite membranes via secondary seeded growth on the outer surface of mullite porous tubes. Reference Wu, Diaz, Zheng, Zhou, Funke, Falconer and Noble24 The resultant membranes displayed remarkably high N2/CH4 separation selectivities of 13 but relatively low N2 permeances [2.2 × 10−8 mol/(m2 s Pa) = 66 GPU] at 20 °C for a feed pressure of 270 kPa. Of all the reported molecular sieve membranes for N2/CH4 separation, SAPO-34 membranes have displayed the best overall separation performance for this binary gas mixture. Therefore, the rest of this section will center on SAPO-34 membranes for N2/CH4 separation.
SAPO-34, is a chabazite small pore zeolite silicoaluminophosphate displaying average pore size of 0.38 nm and having the composition Si x Al y P z O2, where x = 0.01–0.98, y = 0.01–0.60, and z = 0.01–0.52. Reference Szostak25 Fig. 2 shows a schematic of the framework structure of SAPO-34. SAPO-34 represents an ideal candidate in membrane form to molecular sieve N2 from CH4. Two decades ago, the first example of a continuous SAPO-34 membrane was documented. Reference Zhang, Jia and Enze26 Noble and Falconer groups have demonstrated the successful synthesis of high-performance SAPO-34 membranes being able to efficiently separate diverse gas mixtures including CO2, H2, CH4, and other light hydrocarbons. Reference Wu, Diaz, Zheng, Zhou, Funke, Falconer and Noble24,Reference Poshusta, Tuan, Falconer and Noble27–Reference Funke, Chen, Prakash, Falconer and Noble35 The sharp molecular sieving properties of SAPO-34 membranes have been demonstrated for CO2/CH4, Reference Wu, Diaz, Zheng, Zhou, Funke, Falconer and Noble24,Reference Poshusta, Tuan, Falconer and Noble27–Reference Funke, Chen, Prakash, Falconer and Noble35 CO2/N2, Reference Venna and Carreon36,Reference Li and Fan37 CO2/butane, Reference Feng, Zong, Elsaidi, Jasinski, Krishna, Thallapally and Carreon38 and Kr/Xe Reference Feng, Zong, Elsaidi, Jasinski, Krishna, Thallapally and Carreon38,Reference Kwon, Kiang, Benjamin, Crawford, Nair and Bhave39 gas mixtures. In all these cases, the smaller molecules diffuse rapidly through the pores of SAPO-34, while the larger molecules at most diffuse slowly, resulting into high separation selectivities.

FIG. 2. General schematic of SAPO-34 structure (CHA topology). Modified from database of zeolite structures: http://www.iza-structure.org/databases/.
The next paragraphs describe and discuss the state of the art work related to SAPO-34 membranes for N2/CH4 separation. Typically, a continuous polycrystalline SAPO-34 membrane can be grown on a porous support to ensure mechanical strength. These membranes are mainly prepared by the in situ synthesis or secondary seeded growth. For the in situ crystallization approach, the porous support is immersed into the gel synthesis solution, and then the membrane grows solvothermally by direct crystallization. In the secondary seeded growth approach, crystals or seeds are used to promote heterogeneous nucleation (rather than homogeneous nucleation in solution) at the support–membrane interface. In general, this method gives a better control over the membrane formation and quality. Therefore, for SAPO-34 membrane synthesis, typically secondary seeded growth is preferred. All reported SAPO-34 membranes for N2/CH4 separation have been successfully grown via secondary seeded growth on porous alumina supports. Reference Li, Zong, Zhou, Huang, Song, Feng, Zhou, Meyer, Yu and Carreon12,Reference Wu, Diaz, Zheng, Zhou, Funke, Falconer and Noble24,Reference Huang, Wang, Song, Li and Yu40–Reference Zong and Carreon42 These porous tubes were typically 6 cm long with effective permeation areas between 6.5 and 7.5 cm2.
Wu et al. Reference Wu, Diaz, Zheng, Zhou, Funke, Falconer and Noble24 prepared SAPO-34 membranes by secondary seeded growth on the inside of seeded, porous Al2O3 tubes (1-cm O.D., 100 nm average pore size, 40–55% porosity). The membrane synthesis was carried out at 180 °C for 6 h using a gel composition of 0.85 Al2O3:1.0 P2O5:0.3 SiO2:2.0 TEAOH:155 H2O. The template was removed by vacuum calcination at 450 °C for 6 h with heating and cooling rates of 1 °C/min. The authors reported a N2/CH4 selectivity of 5–7 for a feed pressure of 350 kPa and N2 permeance of ∼300 GPU. Although the separation selectivity was ∼ half of that observed for SSZ-13 membranes, Reference Wu, Diaz, Zheng, Zhou, Funke, Falconer and Noble24 the observed N2 permeance was ∼5 times higher. The higher N2 permeance of SAPO-34 membranes as compared to SSZ-13 membranes was likely related to the membrane thicknesses (∼3 μm for SAPO-34 membranes versus ∼8 μm for SSZ-13 membranes).
With the hope of improving the separation performance of SAPO-34 membranes for N2/CH4 separation, we explored several synthesis parameters, including water content in the membrane gel, crystallization time, support pore size, and aluminum source. Reference Li, Zong, Zhou, Huang, Song, Feng, Zhou, Meyer, Yu and Carreon12 We found that high-performance N2-selective membranes were obtained on 100-nm-pore alumina tubes by using Al(i-C3H7O)3 as aluminum source with a crystallization time of only 6 h. These membranes separated N2 from CH4 with N2 permeance as high as 500 GPU and with N2/CH4 separation selectivity as high as 8 at 24 °C for a 50/50 N2/CH4 mixture. Reference Li, Zong, Zhou, Huang, Song, Feng, Zhou, Meyer, Yu and Carreon12 We found that the heat of adsorption was higher for CH4 (15 kJ/mol) than N2 (11 kJ/mol), leading to a preferential adsorption of CH4 over N2 in the N2/CH4 mixture. On the other hand, the N2 molecule diffused much faster than the CH4 molecule suggesting that differences in diffusivity played a more critical role than the competitive adsorption. As such, the SAPO-34 membranes were selective for N2 over CH4 in the mixture. Reference Li, Zong, Zhou, Huang, Song, Feng, Zhou, Meyer, Yu and Carreon12
In a very elegant work, Yu and Li ’s research groups used high-aspect-ratio-SAPO-34 seeds to synthesize via secondary seeded growth thin SAPO-34 membranes for N2/CH4 separation. Reference Huang, Wang, Song, Li and Yu40 Figure 3 shows the remarkable control over seeds aspect ratio from ∼1 to ∼20. These thin seeds were used to synthesize high quality SAPO-34 membranes. Authors claimed that some of these seeds contain the thinnest SAPO-34 crystals reported thus far, which correspond to ∼10 unit cells in thickness. Reference Huang, Wang, Song, Li and Yu40 These seeds were used to grow SAPO-34 membranes with thicknesses up to ∼2.3 μm. Interestingly, the authors found that the seed aspect ratio (AR) not only affected the thickness of membranes but also played a crucial role on the control of defect formation. High-aspect-ratio crystals packed much better and formed a much thinner seeding layer than cubic regular crystals. In principle, this helped to minimize the concentration of nonzeolite pores/defects while maintaining a thin membrane thickness. The resultant membranes were tested for separation of equimolar N2/CH4 mixtures at 22 °C and at a fixed feed and permeate pressure of 275 kPa and 101 kPa, respectively. These SAPO-34 membranes displayed N2/CH4 selectivities as high as 11.2 with N2 permeance of ∼860 GPU.

FIG. 3. SEM images (A) and XRD patterns (B) of SAPO-34 seeds with average aspect ratio (AR) of (a) 1, (b) 3, (c) 10, and (d) 20. The insets of each SEM images provided a schematic drawing of the typical morphology of a seed. Scale bars in (a), (b), and (c), 1 μm; in (d), 0.5 μm. Reprinted from Ref. Reference Huang, Wang, Song, Li and Yu40 with permission of Wiley-VCH.
In another report, we optimized synthesis conditions for SAPO-34 membranes toward N2/CH4 separation by diluting the membrane gels. Reference Zong, Feng, Huang, Song, Zhou, Zhou, Carreon, Yu and Li41 Specifically, we used the gel composition of 1.0 Al2O3:1.0 P2O5:0.3 SiO2:1.0 TEAOH:1.6 DPA:x H2O (where x varied from 150 to 400). In Figure 4 representative sanning electron microscope (SEM) images of the membrane surfaces [Fig. 4(A)] show well-intergrown micron range zeolite crystals for SAPO-34 membranes prepared with different water contents. Cross-sectional SEM images of the membranes [Fig. 4(B)] show zeolite layers. The thickness of the membranes decreased from ∼6.8 to ∼2.7 μm as the water content increases from 150 to 400; indicating that diluted gel forms a thinner membrane layer.

FIG. 4. Top (A) and cross-sectional view SEM (B) images of SAPO-34 membranes prepared with gel compositions of 1.0 Al2O3:1.0 P2O5:0.3 SiO2:1.0 TEAOH:1.6 DPA:x H2O: (a) x = 150, (b) x = 200, (c) x = 250, (d) x = 300, and (e) x = 400. Reprinted from Ref. Reference Zong, Feng, Huang, Song, Zhou, Zhou, Carreon, Yu and Li41 with permission of Elsevier.
These SAPO-34 membranes separated N2 from CH4 with N2 permeance as high as 1300 GPU and selectivity of 7.4 at 23 °C for a 50/50 N2/CH4 mixture. Reference Zong, Feng, Huang, Song, Zhou, Zhou, Carreon, Yu and Li41 Furthermore, membranes were reproducible and effectively separated gas mixtures having low N2 content which are relevant compositions of natural gas.
Recently our group reported SAPO-34 membranes synthesized in stainless steel autoclaves and Teflon autoclaves with water bath, ice/water bath, and flowing water cooling for N2/CH4 separation. Reference Zong and Carreon42 Interestingly, we found that the synthesis in the stainless steel autoclaves cooled with a water bath, ice/water bath, and flowing water cooling resulted in thinner SAPO-34 membranes (as compared to the Teflon liners) and thus into higher permeances for N2/CH4 separation and slightly improved N2/CH4 selectivities. Specifically, the best membrane displayed N2 permeance of ∼2600 GPUs and N2/CH4 separation selectivity of 7.4. This membrane was synthesized in the stainless steel autoclave with a water bath cooling. A more selective membrane with a N2/CH4 selectivity of 8.6 and N2 permeance of ∼2150 GPUs was also prepared in the stainless steel autoclave but with an ice/water bath cooling. These N2 permeances are the highest reported for any SAPO-34 membrane for this particular binary gas mixture.
Comparing the separation performance of the membranes prepared in the stainless steel autoclaves versus the Teflon liners, we found that the stainless steel autoclaves promoted better membrane performance than those in the Teflon liners, especially for N2 permeance. Reference Zong and Carreon42 The enhanced N2 permeance was explained by a simple one-dimensional Fourier heat law analysis. Reference Zong and Carreon42
In particular, we found that the heat flux rate of the stainless steel autoclaves during membrane preparation was ∼ two orders of magnitude higher than that of the membranes prepared in conventional Teflon liners. The higher heat flux rate slowed down the kinetics of crystal growth, translating into thinner and more N2 permeable membranes.
VI. ALUMINO PHOSPHATE MEMBRANES FOR N2/CH4 SEPARATION
AlPO-18 is a microporous aluminophosphate (AlPO), consisting of AlPO4- and PO4-tetrahedral units. Reference Lok, Messina, Patton, Gajek, Cannan and Flanigen43 AlPO-18 with AEI topology, possesses a three-dimensional framework with a crystallographic pore size of ∼0.38 nm. Reference Lok, Messina, Patton, Gajek, Cannan and Flanigen43 Based on the kinetic diameter of N2 (∼0.36 nm) and CH4 (∼0.38 nm) and the micropore size of AlPO-18, this aluminophosphate could potentially molecular sieve N2 over CH4 or at least make that N2 molecules diffuse rapidly through the pores, while CH4 at most will diffuse slowly meaning that high separation N2 selectivities could be potentially achieved based on molecular diffusion differences. Our group reported the first example of continuous AlPO-18 membranes exhibiting molecular sieving properties for CO2/CH4 gas mixtures. Reference Carreon, Li and Carreon44 Later reports by Zhou group confirmed the molecular sieving properties of AlPO-18 membranes for CO2/CH4, CO2/N2, and H2/CH4 binary gas mixtures. Reference Wu, Wang, Lu, Zhou and Chen45,Reference Wang, Hu, Wang, Zheng and Zhou46
Inspired by the molecular sieving ability of AlPO-18 membranes for these binary gas mixtures, our group recently reported the separation performance of this AlPO for N2/CH4 mixtures. Reference Zong, Elsaidi, Thallapally and Carreon47 These AlPO-18 membranes were grown via secondary seeded growth on alumina porous tubes. Figure 5 shows representative top and cross-section view SEM images of AlPO-18 membranes. The top view SEM shows well-intergrown rectangular AlPO-18 crystals on the surface of the alumina support [Fig. 5(a)]. The size of the surface crystals of the membrane was larger than that of the seeds, suggesting that secondary seed growth led to a recrystallization process. Cross-sectional SEM image showed a ∼2.4 μm thick dense aluminophosphate layer [Fig. 5(b)].

FIG. 5. Representative SEM images of AlPO-18 membranes: (a) top and (b) cross-sectional views. Reprinted from Ref. Reference Zong, Elsaidi, Thallapally and Carreon47 with permission of American Chemical Society.
These AlPO-18 membranes were used to separate premixed equimolar N2/CH4 mixtures. Reference Zong, Elsaidi, Thallapally and Carreon47 The separation results for these membranes are shown in Table II. AlPO-18 membranes displayed N2 permeances as high as 3076 GPU, with N2/CH4 separation selectivities as high as 4.7. To the best of our knowledge, this is the highest N2 permeance reported for any membrane for this particular binary mixture. Although the separation selectivity is moderate, preliminary economic evaluation for N2/CH4 separation Reference Li, Zong, Zhou, Huang, Song, Feng, Zhou, Meyer, Yu and Carreon12 suggests that high N2 permeances are essential to reduce the N2 rejection cost employing membrane technology. In Table II, separation index Reference Carreon, Li, Falconer and Noble31 is defined as [π = N2 permeance × (selectivity − 1) × permeate pressure]. This index has been used as a reliable parameter to predict porous crystalline membrane reproducibility. Reference Carreon, Li, Falconer and Noble31,Reference Feng, Zong, Elsaidi, Jasinski, Krishna, Thallapally and Carreon38,Reference Zong and Carreon42,Reference Zong, Elsaidi, Thallapally and Carreon47,Reference Wu, Feng, Elsaidi, Thallapally and Carreon48
TABLE II. Separation performance of AlPO-18 membranes for equimolar N2/CH4 mixtures. Reference Zong, Elsaidi, Thallapally and Carreon47

AlPO-18 membranes displayed separation indexes π in the 0.11–0.25 mol/m2 s range, indicating good membrane reproducibility.
Table III compares N2 permeances and N2/CH4 selectivities for the reported molecular sieve membranes. All these membrane compositions surpass the polymer membrane upper bound line for N2/CH4. Reference Robeson22 SSZ-13 membranes display the highest reported N2/CH4 selectivities, but only moderate N2 permeances. Reference Wu, Diaz, Zheng, Zhou, Funke, Falconer and Noble24 On the other hand, AlPO-18 membranes show the highest reported N2 permeances, at moderate N2/CH4 selectivities. Reference Zong, Elsaidi, Thallapally and Carreon47 SAPO-34 membranes show the best overall separation performance, with N2 permeances as high as 2591 GPU Reference Zong and Carreon42 and N2/CH4 separation selectivities as high as 11.3 Reference Huang, Wang, Song, Li and Yu40 In the next paragraphs, the separation mechanisms involved in these molecular sieve membranes for N2/CH4 gas mixtures are discussed and correlated with their observed separation performance.
TABLE III. Comparison of molecular sieve membranes used for N2/CH4 separation.

VII. MEMBRANE SEPARATION MECHANISMS
A. Carbon molecular sieve (CMS) membrane separation mechanisms
The idealized pore structure of CMS membranes has a bimodal pore size distribution consisting of micropores and ultramicropores. Reference Ning and Koros19 The rigid window pore aperture of the ultramicropores lies between the kinetic diameter of nitrogen and methane, and therefore high diffusion separation selectivity (due to effective size and shape diffusion) has been observed for N2/CH4 mixtures over CMS membranes. Reference Fu, Sanders, Kulkarni, Wenz and Koros18 Furthermore, the rigid pore size nature of CMS membranes introduced an additional factor contributing to the separation mechanism: entropic diffusion selectivity. This factor provides additional diffusion selectivity. Reference Rungta, Xu and Koros15,Reference Singh and Koros49
B. Zeolite and AlPO-18 membrane separation mechanisms
It has been proposed that N2/CH4 gas mixtures can be separated over SAPO-34 Reference Li, Zong, Zhou, Huang, Song, Feng, Zhou, Meyer, Yu and Carreon12,Reference Zong, Feng, Huang, Song, Zhou, Zhou, Carreon, Yu and Li41,Reference Zong and Carreon42 and AlPO-18 Reference Zong, Elsaidi, Thallapally and Carreon47 membranes by at least one of the following separation mechanisms: (a) molecular sieving, in which larger molecules cannot fit into the pores, and therefore the smaller molecules preferentially permeate; (b) competitive adsorption, in which one type of molecule is more strongly adsorbed on the microporous structure and thus can dramatically inhibit permeation of the second molecule; and (c) differences in diffusivity, in which the smaller, less hindered type of molecule in a mixture diffuses faster than the larger ones. In the case of SSZ-13 membranes, (although not reported) it is expected that at least one of these separation mechanisms is present. In principle, all these three membrane compositions (based on its crystallographic pore size and the kinetic diameter of nitrogen and methane) could display some degree of molecular sieving. However, competitive adsorption and differences in diffusivities have been identified as the most dominant separation mechanisms for N2/CH4 gas mixtures.
Nitrogen and methane adsorption isotherms have been used to learn about the competitive adsorption between these two gases. Column breakthrough experiments have been very useful to have a better understanding on the relative difference in diffusivities between N2 and CH4.
To learn about these separation mechanisms, our group collected adsorption isotherms for N2 and CH4 measured for AlPO-18. Reference Wang, Hu, Wang, Zheng and Zhou46 The adsorption isotherms at 140 kPa which corresponded to the transmembrane pressure used during the separation experiments showed CH4 uptakes of 12 and 15 cm3/g at 298 K and 278 K, respectively, and N2 uptakes of 4.5 and 10 cm3/g, respectively, (Fig. 6). These results indicated that, at the studied separation conditions of ∼140 kPa and at room temperature, AlPO-18 adsorbed ∼2.7 times more CH4 than N2. We attributed the preferential adsorption of CH4 over N2 in AlPO-18 to differences in polarizabilities of these two molecules. Specifically, CH4 has higher polarizability (25.9 × 1025/cm3) as compared to N2 (17.4 × 1025/cm3). Reference Poling, Prausnitz and O’Connell50 Therefore, stronger electrostatic interactions between the AlPO-18 surface carrying partial charges Reference Deroche, Gaberova, Maurin, Llewellyn, Castro and Wright51 and the higher polarizability of CH4 promoted its preferential adsorption over N2. Ideal adsorbed solution theory (IAST) indicated that at the used separation conditions, AlPO-18 adsorbed more CH4 than N2, in well agreement with the single adsorption isotherms. Reference Zong, Elsaidi, Thallapally and Carreon47

FIG. 6. Single component CH4 and N2 adsorption isotherms for AlPO-18 collected at 298 K and 278 K indicating CH4 preferential adsorption. Reprinted from Ref. Reference Zong, Elsaidi, Thallapally and Carreon47 with permission of American Chemical Society.
To learn about the relative diffusivity differences between N2 and CH4, we conducted column breakthrough experiments (Fig. 7) over AlPO-18. Reference Zong, Elsaidi, Thallapally and Carreon47 We found that the retention time of CH4 in the breakthrough was longer, and therefore N2 diffused faster than CH4. This was expected since nitrogen has lower affinity to AlPO-18 as demonstrated by the single adsorption isotherms and IAST, as well as to its smaller kinetic diameter. Therefore, breakthrough column experiments suggested that differences in diffusivity favor the separation of N2 over CH4 in the gas mixture.

FIG. 7. Column breakthrough experiments for 50:50 CH4/N2 gas mixture over AlPO-18. Reprinted from Ref. Reference Zong, Elsaidi, Thallapally and Carreon47 with permission of American Chemical Society.
Adsorption isotherms and IAST indicated that CH4 adsorbs more strongly than N2. Therefore, the preferential adsorption of CH4 would favor separating CH4 over N2 in the mixture. On the other hand, breakthrough experiments confirmed higher diffusivity of N2 over CH4 favoring N2 selectivity. Thus, the differences in diffusivities would favor separating N2 over CH4 in the mixture. These results suggested that for AlPO-18 membranes, differences in diffusivities between N2 and CH4 played a more dominant role than competitive adsorption.
For SAPO-34 membranes, adsorption isotherms and single-gas permeation were measured for N2 and CH4 to help explain the N2/CH4 separation mechanism for the N2-selective membranes. Reference Zong, Feng, Huang, Song, Zhou, Zhou, Carreon, Yu and Li41 As shown in Fig. 8, adsorption isotherms showed that CH4 adsorbs more strongly than N2 on SAPO-34 crystals (CH4 adsorbs 1.8–4 times more for than N2). Therefore the ideal N2/CH4 adsorption selectivities were in the 0.25–0.55 range. Thus, the preferential adsorption of CH4 would favor separating CH4 over N2 in the mixture. The preferential adsorption of CH4 over N2 in SAPO-34 was explained by differences in dipole polarizabilities of these two molecules. Due to local electronegativity differences between framework Si, Al, and P, SAPO-34 has an anionic framework with a net negative charge depending upon the silicon substitution into the framework. Reference Lok, Messina, Patton, Gajek, Cannan and Flanigen43 Therefore, stronger electrostatic interactions between the negatively charged SAPO-34 surface and the higher polarizability of CH4 promoted its preferential adsorption over N2. On the other hand, N2 a smaller molecule, as compared to CH4, diffuses faster. In fact, it has been reported that diffusivity of N2 is 24 times that of CH4 through a SAPO-34 membrane. Reference Li, Falconer, Noble and Krishna52 This indicates that differences in diffusivity would favor separating N2 over CH4 in the mixture. Since the reported SAPO-34 membranes were N2 selective, Reference Zong, Feng, Huang, Song, Zhou, Zhou, Carreon, Yu and Li41 these results (and similar to AlPO-18 membranes) suggest that differences in diffusivity played a more critical role than the competitive adsorption. Reference Zong, Feng, Huang, Song, Zhou, Zhou, Carreon, Yu and Li41

FIG. 8. Adsorption isotherms of N2 and CH4 on SAPO-34 at 23 °C. Reprinted from Ref. Reference Zong, Feng, Huang, Song, Zhou, Zhou, Carreon, Yu and Li41 with permission of Elsevier.
VIII. CONCLUSIONS AND OUTLOOK
During the last few years promising research progress has been done in the development of molecular sieve membranes for N2/CH4 separation. Some of these membranes display separation performances which are appealing for its potential use at industrial level for nitrogen rejection from natural gas. Although these membranes display overall good separation performance for N2/CH4 gas mixtures, practical challenges that may prevent its potential industrial application need to be addressed. Some of the keys aspects that require attention from the scientific community to envision these molecular sieve membranes as a potential economic viable technology to effectively separate this highly challenging gas mixture are discussed in the next paragraphs.
A. Novel membrane compositions
In principle, a “sharper” molecular sieving effect leading to higher N2/CH4 separation selectivities can be achieved by developing membranes with rigid pore sizes lying exactly between the kinetic diameters on N2 and CH4. In this respect, two ideal candidates for N2/CH4 separation are: SAPO-17 and AlPO-17. These microporous crystalline phases display an effective pore size of 0.36 nm, Reference Lohse, Löffler, Kosche, Jänchen and Parlitz53 ideal to molecular sieve N2 (0.36 nm) from CH4 (0.38 nm). Recently, the molecular sieve properties of SAPO-17 and AlPO-17 have been demonstrated for CO2/CH4 and CO2/N2 mixtures. Reference Zhong, Bu, Zhou, Jin, Yu and Li54 Another potential candidate to effectively separate N2/CH4 via molecular sieving is the zeolite DNL-6. This zeolite is composed of α-cages linking through 8-rings pore size of 0.36 × 0.36 nm having a body-centered cubic symmetry structure of RHO. Reference Su, Tian, Li, Zhang, Meng, He, Fan and Liu55,Reference Su, Tian, Fan, Xia, Yang, Xu, Zhang, Zhang, Wang and Liu56 If prepared in membrane form, DNL-6 can potentially separate N2/CH4 mixtures at high separation selectivities.
B. Ion exchanged membranes
In principle, membrane surface modification should change the adsorption capacity of N2 and CH4. SAPO-34 Reference Li, Zong, Zhou, Huang, Song, Feng, Zhou, Meyer, Yu and Carreon12 and AlPO-18 Reference Zong, Elsaidi, Thallapally and Carreon47 membranes display preferential adsorption of CH4 over N2, leading to a strong competitive separation mechanism for N2/CH4 gas mixtures. Therefore, if the preferential CH4/N2 adsorption selectivity is reversed or at least attenuated, then much higher separation selectivities than those reported so far can be achievable. Several mono and divalent cations have been used successfully to modify the surface of zeolite membranes and have been demonstrated to be successful in improving gas pair separation selectivities. Reference Hong, Li, Funke, Falconer and Noble57,Reference Chew, Ahmad and Bhatia58 In particular, Li+, Na+, and K+ ions are suitable candidates to ion exchange zeolite or aluminophosphate membranes to enhance the N2 adsorption capacity. It has been demonstrated that these cations considerably enhance the adsorption capacities to nitrogen as compared to non-exchanged zeolites. Reference Pham, Liu and Lobo59
C. Presence of impurities and realistic separation conditions
Realistic separation conditions for nitrogen rejection from natural gas may require the membrane to perform well at high pressures and or temperatures and in the presence of other natural gas impurities (see Table I). The overall separation performance of the membranes under high pressure and temperature as well as in the presence of other natural gas impurities, will likely decrease. To our best knowledge there are no reported studies in the open literature addressing the stability and performance of membranes under real natural gas conditions (presence of impurities, effect of temperature, and pressure).
D. Membrane reproducibility
Limited reported data on membrane reproducibility is a common problem found in microporous crystalline membranes. Studies on promising membrane compositions for N2/CH4 separation should address this issue. The separation index (π) Reference Carreon, Li, Falconer and Noble31 has been used as a metric to “quantify” membrane reproducibility for zeolites and aluminophosphates. Other metrics would be useful to assess membrane reproducibility.
E. Membrane scale-up studies
Once promising membrane compositions displaying good separation performance for N2/CH4 mixtures have been identified at laboratory level, scale-up studies may be required to assess the potential use of these membranes at pilot plant scale. Scaling-up membrane synthesis is not a trivial issue and requires considerable effort from the scientific community. Li et al. Reference Li, Carreon, Zhang, Funke, Noble and Falconer60 demonstrated the successful scale-up of SAPO-34 membranes for CO2/CH4 separations, a binary mixture which is of central relevance for natural gas purification. Studies like this are needed to assess the potential effectiveness of membranes for molecular gas separations not only at laboratory scale but also at pilot plant level.
F. Cost
The relatively high cost associated to the membrane support (in particular for zeolite and aluminophosphate compositions) may limit its potential practical application for N2/CH4 separations. The support accounts for the higher total membrane cost. Therefore, research efforts should consider the use of cheaper membrane supports. In addition, the economics of any separation technology needs to be evaluated in the context of metrics such as cost per kilogram of product and energy use per kilogram. Reference Sholl and Lively61
If these challenges and issues are properly tackled, one may see in the near future some of these molecular sieve membranes as a feasible economic technology for nitrogen rejection in natural gas processing.
ACKNOWLEDGMENTS
M.A. Carreon acknowledges the Renewable Energy Materials Research Science and Engineering Center (REMRSEC) under Award Number DMR-0820518.