Introduction
The human central nervous system (CNS) is a highly complex biological tissue, comprised of neurons and support cells called glia (Refs Reference Silbereis1, Reference Augusto-oliveira2). Together with neurons, glial cells produce and assemble a highly organised extracellular matrix (ECM) that makes up approximately 20% of the total volume of the adult CNS (Refs Reference Bignami, Hosley and Dahl3, Reference Dzyubenko, Gottschling and Faissner4, Reference Song and Dityatev5). The ECM has a unique composition and is mainly composed of proteoglycans (aggrecan, brevican, neurocan and versican), glycosaminoglycans (GAGs) (e.g., hyaluronan), glycoproteins (e.g., tenascin-R and tenascin-C), and relatively low levels of fibrous proteins such as collagen and fibronectin (Refs Reference Simão6, Reference Burnside and Bradbury7). Today, it is known that each ECM component in the CNS plays diverse roles in neurite outgrowth, axonal guidance, cell migration and differentiation as well as synaptogenesis (Refs Reference Bonneh-Barkay and Wiley8, Reference Melrose, Hayes and Bix9).
Once formed, the composition of the mature ECM is relatively stable under physiological conditions (Refs Reference Zimmermann and Dours-Zimmermann10, Reference Jakeman, Williams and Brautigam11). However, inflammatory responses to prevent the expansion of tissue damage after traumatic injuries or during neurodegenerative diseases alter the composition of the CNS ECM and result in remodelling (Ref. Reference Willis and Crocker12). Tissue damage leads to upregulation and overexpression of some inhibitors along with a breakdown of ECM molecules, altering ECM homeostasis and the ability to prevent neurodegeneration (Refs Reference Castillo13, Reference Busch and Silver14, Reference Berretta15, Reference Afshari, Kappagantula and Fawcett16). Although neurogenesis and migration are increased to the injured area, most of the new neurons die or migrate to other areas because of the improper environment (Ref. Reference Pérez-Garnes17).
Over the past decade, numerous studies have revealed that biomaterials have promoted CNS tissue regeneration (Refs Reference Zhong and Bellamkonda18, Reference Khaing19, Reference Chen, Harn and Chiou20) through enhancing cell-tissue attachment and/or entrapment by mimicking the native tissue, as well as improving the environment to provide vascularisation and promote cell survival (Refs Reference West and Moon21, Reference Cooke, Vulic and Shoichet22). Although individual ECM components, such as collagen, hyaluronic acid, and fibronectin scaffolds, have been developed for CNS applications, the majority of the solutions have not fully recapitulated the native tissue due to the highly complex composition of the ECM. Therefore, decellularised CNS ECM recently utilised as the native tissue can be more closely mimicked.
Decellularisation is the process of removing all cellular and nuclear components from a tissue or an organ to prevent an initial immune response while preserving the ultrastructure and composition of the native ECM (Refs Reference Seon23, Reference Garreta24). The decellularised tissue can be in the form of a porous solid, ground to obtain powder form and gelated to formulate a hydrogel. The well-preserved decellularised ECM possesses similar concentrations and ratios of GAGs, fibrous and adhesion proteins and can promote the regenerative response specific to the ECM's tissue or organ of origin (Ref. Reference Beachley25). Furthermore, decellularised extracellular matrix (dECM) can be well tolerated even by xenogeneic recipients as the molecules are conserved across species (Ref. Reference Zhang, Pizzute and Pei26). Compared to solid dECM, injectable form is more suitable for CNS applications, particularly for neurogeneration, allowing a minimally invasive delivery without the need of surgical access for implantation (Ref. Reference Osama27).
For effective neuroregeneration, stem cells (SCs) with responses such as mitogenesis, chemotaxis and secretion of several neurotrophic factors with subsequent neuroprotective/neurorestorative effects have been reported (Refs Reference Crapo28, Reference Yasuhara29). Transplanted SCs have to survive, grow, proliferate and differentiate specific to its location and also integrate into the host circulatory system for accomplishing regenerative outcomes (Refs Reference Mahla30, Reference Saglam-Metiner, Gulce-Iz and Biray-Avci31). For recellularisation studies of brain dECM scaffolds and neurorestoration via cell transplantation, mesenchymal SCs (MSCs) and induced pluripotent SCs (iPSCs) have been utilised (Ref. Reference Yasuhara29). MSCs are widely studied adult SCs as part of therapeutic cell transplantation to repair failed tissues and organs for regenerative medicine and treatment of various diseases such as Parkinson's, and ischemic stroke with the capability to replace and regenerate damaged tissues with no immunogenic effect (Refs Reference Maleki32, Reference Barzegar33, Reference Schmelzer, Mckeel and Gerlach34). Various studies have shown that neural, bone marrow and adipose-derived MSCs can be induced to express a neuronal cell phenotype, trans/differentiate to neural precursors and/or mature neurons and promote neuroprotection and neurogenesis in vitro under specific experimental conditions for the treatment of CNS diseases (Refs Reference Crapo28, Reference Scintu35, Reference Han36, Reference Baiguera37, Reference Urrutia38). On the other hand, iPSCs are embryonic SC-like cells that are reprogrammed from somatic cells to a pluripotent stage by the induction of specific transcription factors (four well-described reprogramming factors: Oct4, Sox2, c-Myc and Klf4), having the capacity of unlimited self-renewal and differentiation to create all three germ lines and all cell types in the human body, including neurons (Ref. Reference Forsberg, Ilieva and Michel39). The immunogenicity of iPSCs has been reported to change due to fatal errors or mutations during reprogramming and differentiation processes, while the risk of rejection has been shown to be low when administered autologously (Ref. Reference Barzegar33), also presenting a potential as a regenerative medicine in CNS diseases (Refs Reference Yasuhara29, Reference Zakrzewski40).
Apart from highlighted applications, dECM-based biomaterials have been utilised in various tissue engineering approaches such as support materials for 3D cell culture studies (e.g., brain organoids), injectable hydrogels for the repair of tissue damage, carriers for drug and growth factors, composite materials for neuroregeneration, substrates mimicking native tissue microenvironment in organ-on-a-chip platforms, and bioinks for 3D bioprinting (Fig. 1). This review focuses on the physical, chemical and biological methods for decellularisation of brain and spinal cord ECM in the context of CNS applications and specifically discusses innovative applications.
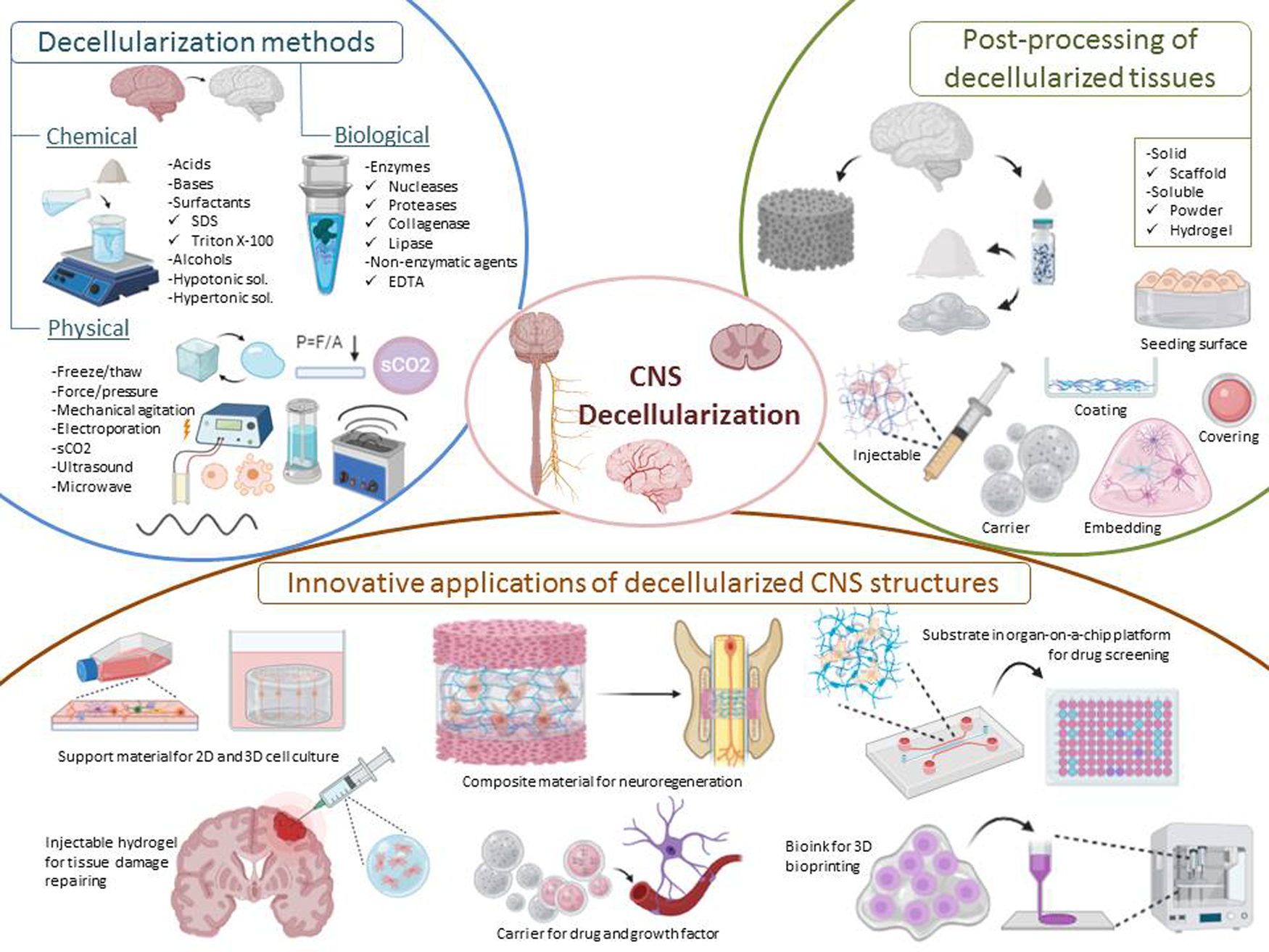
Fig. 1. Representative image of decellularisation methods, post-processing of decellularised tissues and innovative applications of CNS structures.
Decellularisation methods
As the decellularisation process requires a delicate balance between the preservation of native ECM structure and effective cell removal, complete removal of all cellular components such as DNA, nuclei, membrane lipids from a tissue is not possible. However, these cellular remnant components have been shown to initiate a pro-inflammatory response upon in vivo implantation, resulting in inhibition of constructive tissue remodelling. Therefore, three criteria have been proposed to prevent an adverse host response; (i) the amount of DNA should be <50 ng/mg dry weight of ECM, (ii) the length of DNA fragments should be <200 bp, and (3) visible nuclear material, cell debris, endotoxin and bacterial contamination should not be observed in histological analysis (Ref. Reference Crapo, Gilbert and Badylak41). However, DNA fragments have to be <24 bp for CNS applications as immune system cells such as microglia and mast cells can recognise DNA fragments of 24 bp. Residual DNA, degraded ECM components and cell debris in the decellularised tissue turn into a damage-associated molecular pattern (DAMP) when break down, leading to unwanted inflammation activation through Toll-like receptors, microRNA activation and secretion of IL-6, IL-1, TNF and IFN-γ as pro-inflammatory cytokines in M1 macrophage polarisation. Although some of the DAMPs have constructive functions, often destructive effects are observed on ECM (Refs Reference Crapo42, Reference Kamat, Kudchadkar and Simon43, Reference Hillebrandt44).
Decellularisation protocol depends upon several factors such as cell density, lipid content and thickness of the tissue (Ref. Reference Chakraborty, Roy and Ghosh45), which have to be taken into account for an effective decellularisation. In this context, various methods including physical, chemical and biological methods have been employed.
The majority of the physical methods rely on heating, cooling, mechanical and electrical approaches (Table 1). Freeze–thaw consists of freezing tissues or organs at −80 °C and thawing it at biological temperature (37 °C). During the process, intracellular ice crystal formation disrupts cell membranes, leading to cell lysis. However, multiple freeze–thaw cycles or the combination of freeze–thaw cycles and detergents may be required to enhance decellularisation, especially in dense tissues (Ref. Reference Burk46). It is important to note that freeze–thaw may cause certain disruption of the ECM ultrastructure (Ref. Reference Pulver47). Cells on the surface of a thin tissue organ (i.e., small intestine and skin) can be effectively removed by mechanical abrasion (Ref. Reference Hopkinson48). However, this method is often used by coupling with hypertonic saline, enzymes, or chelating agents to facilitate efficient detachment of cells from underlying basement membrane (Ref. Reference Fu49).
Table 1. A summary of physical methods

In the non-thermal irreversible electroporation process, microsecond electrical pulses induce the formation of irreversible micropores in the cell membrane lipid bilayer, causing cell death through loss of cell homeostasis, while preserving the tissue scaffold and large blood vessels (Refs Reference Golberg and Yarmush50, Reference Phillips, Maor and Rubinsky51, Reference Chang, Zhou and Rubinsky52). Mechanical agitation and sonication are other approaches used in combination with chemical or biological decellularisation agents as the penetration of agents into tissue is enhanced and the removal of cellular components is facilitated by improving mass transfer in the system (Refs Reference Garreta24, Reference Yusof, Sha'ban and Azhim53). In addition to conventional physical methods, supercritical carbon dioxide (SC-CO2) has been proposed as a promising method for decellularisation by means of bursting the cells with high pressure and removing nuclear fragments from the tissues (Refs Reference Seo, Jung and Kim54, Reference Huang55). As the critical pressure (7.4 MPa) and temperature (31 °C) of CO2 to reach supercritical phase are relatively mild, processing of various biological materials has been reported (Refs Reference Ozdemir, Sendemir-Urkmez and Yesil-Celiktas56, Reference Senyay-Oncel, Kazan and Yesil-Celiktas57, Reference Gil-Ramírez58). SC-CO2 shows the properties of both liquids and gases, diffuse through solids like a gas and dissolve substances like liquid allowing a low viscosity and high transport characteristics for simple and short decellularisation protocols. Additionally, SC-CO2 has been reported to enhance protein stability (Ref. Reference Senyay-Oncel and Yesil-Celiktas59), which would highly contribute to maintaining the cytocompatibility. However, the addition of a polar solvent is necessary to remove charged molecules such as DNA and phospholipids (Refs Reference Huang55, Reference Guler60). Although SC-CO2 have been successfully used in the decellularisation of dense tissues such as heart, liver, cartilage and soft tissues such as adipose tissue, which is structurally similar to the brain, it has not been used for decellularisation of CNS structures. In addition to its use in decellularisation, the recent study conducted by our group has shown that supercritical fluid technology can also be used in the sterilisation of decellularised materials (Ref. Reference Yaldiz61). In this study, SC-CO2 with/without ethanol, ultraviolet irradiation and ethylene oxide sterilisation techniques were applied to sodium dodecyl sulphate (SDS)-decellularised sheep brain cortical slices to determine the most effective and mild sterilisation technique. The results showed that SC-CO2 entrained with 6% ethanol sterilised decellularised brain tissues by yielding better results with respect to preservation of ECM proteins (Ref. Reference Yaldiz61).
The majority of the physical methods are frequently applied in combination with chemical or enzymatic methods as complete removal of cellular debris from tissue is not achieved. Until now, several types of chemicals have been used in decellularisation, including acids, bases, hypotonic and hypertonic solutions, detergents, alcohols and other solvents (Table 2). Detergents are amphipathic molecules, containing a hydrophilic head group and a hydrophobic carbon tail. Detergents effectively penetrate the phospholipid bilayer by spontaneous formation of spherical micellar structures in aqueous solutions under optimised temperature and concentration. Overall, detergents used for decellularisation can be divided into three groups: non-ionic (i.e., Triton X-100), ionic (i.e., SDS) and zwitterionic (i.e., 3-([3-colamidopropyl] dimethylammonio)-1-propanesulfonate (CHAPS)). SDS, as the most effective agent, dissolves cell and nuclear membranes by breaking down lipid–lipid and lipid–protein interactions. However, it also leads to the denaturation of structural and functional proteins by disrupting protein–protein interactions and remove GAGs and growth factors from the ECM (Refs Reference Liu62, Reference Alshaikh63). Compared to ionic detergents, zwitterionic detergents exhibit better ECM preservation (Refs Reference Petersen64, Reference Petersen65). Triton-X-100 breaks down DNA–protein, lipid–lipid and lipid–protein interactions and may cause the reduction of GAG content in dECM while preserving protein–protein interactions (Ref. Reference Vavken, Joshi and Murray66). In recent years, detergents have been used in combination to investigate their synergistic effects on decellularisation efficiency. The combined detergent decellularisation protocol showed effective decellularisation with minimal effects on tissue strength and structure in comparison to using detergents individually, which lead to detrimental effects on tissue structure and integrity or ineffective decellularisation (Refs Reference Wu67, Reference Laker, Dohmen and Smit68).
Table 2. A summary of chemical methods

Acids and bases decellularise tissues by catalysing hydrolytic degradation of cellular components and decomposing nucleic acids. Bases are particularly used to remove hair from the dermis sample before starting the process of decellularisation (Ref. Reference Hrebikova, Diaz and Mokry69). But the pretreatment is advised to be carried out with caution as the mechanical properties of the ECM are significantly altered. Peracetic acid is widely preferred to remove residual nucleic acids with the minimal negative impact on the ECM (Ref. Reference Monibi70). As another group of treatment agents, hypertonic and hypotonic solutions induce osmotic shock in tissues, leading to the breakdown of the cell membrane. To achieve the maximum osmotic effect, tissues are usually immersed alternately in hyper and hypotonic solutions for several cycles (Ref. Reference Crapo, Gilbert and Badylak41). Alcohols lyse cells by causing dehydration and breaking down the cell membrane. However, alcohols (i.e., ethanol) are used as tissue fixatives in histology, which might result in poor decellularisation. Furthermore, alcohols might alter the mechanical properties of the ECM by causing excessive shrinkage and hardening (Ref. Reference Yaldiz61).
Various enzymatic (i.e., nucleases, proteases, collagenases, lipases, dispases) and non-enzymatic (i.e., chelating agents) agents are used in biological methods (Table 3). To complete tissue decellularisation by eliminating cell remnants of the ECM, enzymatic agents are often applied after physical and chemical treatments (Refs Reference Baiguera37, Reference Ribatti71). Among these agents, deoxyribonuclease and ribonuclease degrade DNA and RNA, respectively.
Table 3. A summary of biological methods

Proteases such as trypsin cleave the interactions between proteins by hydrolysing peptide bonds and accelerate the decellularisation process (Ref. Reference Rahman72). On the other hand, it can cause a change in the quantification of GAGs to disrupt ECM structure (Ref. Reference Xu73). Chelating agents such as ethylenediaminetetraacetic acid are often coupled with enzymes (i.e., trypsin) and used in breaking down cell–ECM protein adhesions (Ref. Reference Haduong74).
Despite, the significance of brain–ECM microenvironment for the development of in vitro disease models, mostly synthetic or gelatinous protein mixtures secreted by Engelbreth-Holm-Swarm mouse sarcoma cells have been utilised. Such models have limited utility and lack physiological relevancy. Decellularisation of CNS structures can address this challenge by providing high biomimicry. In general, multiple freeze–thaw cycles are used as a pretreatment (Refs Reference Baiguera37, Reference Granato75) of CNS tissues, which is usually followed by treatment with chemical and biological agents in a mechanical agitator (Refs Reference Beachley25, Reference Dequach76, Reference Medberry77, Reference Lin78) (×Fig. 2A). Most decellularisation studies reported significant reductions in DNA quantity and no residual nuclei in H&E and DAPI images in dbECM and dscECM (Refs Reference Crapo42, Reference Arslan, Efe and Sezgin Arslan79, Reference Hong80, Reference Seo81) (Fig. 2B and C). Human brain tissues decellularised by Triton-X-100 have been shown to exhibit porous, fibrous structures (Fig. 2D) and contain ECM components such as collagen, hyaluronic acid and GAGs (Ref. Reference Koh82).

Fig. 2. (A) Image of native brain (left) and dbECM (right) (Reprinted with permission from Lin et al., 2017. Copyright (2016) Elsevier) (Ref. Reference Lin78). (B) (a) After H&E staining, cell nuclei are visible in (left) native spinal cord but not in (right) dscECM, (b) cell nuclei are visible in (left) native brain but not in (right) dbECM, (c) and (d) DAPI staining shows similar results (Reprinted with permission from Crapo et al., 2012. Copyright (2012) Elsevier) (Ref. Reference Crapo42). (C) DNA quantification shows lower concentrations of DNA in dbECM (17 ± 4 ng DNA/mg) compared to native brain (1675 ± 105 ng DNA/mg) (Reprinted with permission from Hong et al., 2020. Copyright (2019) Elsevier) (Ref. Reference Hong80). (D) Scanning electron microscopy image (SEM) of dbECM hydrogel (Reprinted with permission from Koh et al., 2018. Copyright (2018) Springer Nature) (Ref. Reference Koh82). (E) GAG quantification shows lower concentrations of GAG in dbECM (0.7 ± 0.1 μg GAGs/mg) compared to native brain (1.6 ± 0.2 μg GAGs/mg) (Reprinted with permission from Hong et al., 2020. Copyright (2019) Elsevier) (Ref. Reference Hong80). (F) Comparison of mechanical properties of CASPERised brains and native brain (Reprinted with permission from Lee et al., 2019. Copyright (2019) American Chemical Society) (Ref. Reference Lee83). (G) Immunofluorescence staining of the CASPERised brain with wisteria floribunda agglutinin (WFA, red) and collagen type IV (green) (Reprinted with permission from Lee et al., 2019. Copyright (2019) Elsevier) (Ref. Reference Lee83).
It is worth to note that brain and spinal cord are the most fragile parts of the body and contain lower amounts of fibrous protein compared to non-neuronal tissues. Thus, the number of freeze–thaw cycles have to be optimised which might otherwise cause disruption of the ECM ultrastructure. As such, chemical and biological agents may breakdown the lipid–protein and protein–protein interactions, resulting in the removal of proteins and GAGs (Refs Reference Hong80, Reference Seo81) (Fig. 2E). Consequently, the presence of low levels of structural and adhesion proteins as well as GAGs adversely affects cell adhesion and leads to loss of physical strength of dECM. Optimisation of consecutive decellularisation methods is required to elicit process conditions maximizing the concentrations of specific ECM components. The content of dECM is suggested to be improved by addition of various biomaterials such as collagen I, fibronectin, or synthetic polymers or the employment of crosslinking agents. However, both cases have disadvantages of increase in processing time and costs as well as an alteration in biocompatibility of the material which might alter cell-ECM interactions. Therefore, there is a need to develop innovative methods that allow efficacious decellularisation of CNS structures by avoiding excessive physical applications or long-term treatment with chemical and biological agents at high concentrations.
For instance, more recently, a novel decellularisation procedure called CASPER (Clinically and Experimentally Applicable Acellular Tissue Scaffold Production for Tissue Engineering and Regenerative Medicine) is developed, which is inspired by tissue clearing techniques such as CLARITY and ACT to prevent excessive destructive effects of chemical decellularisation methods (Ref. Reference Lee83). Briefly, the procedure consists of three main steps, including the immersion of unfixed organs in a hydrogel monomer solution containing acrylamide and bis-acrylamide, the polymerisation of the hydrogel monomer by increasing the temperature to 37 °C, and subsequently the incubation of samples with a 2–4% SDS solution to remove cells from the organs. The inclusion of the infusion and hydrogel polymerisation steps before the chemical treatment with SDS prevented excessive damage to the ECM and produced a highly porous CASPERised mouse brain matrix with an intact structure (Fig. 2F). In addition, immunofluorescence staining showed that lectin (wisteria floribunda agglutinin) and collagen structures were preserved during the process of decellularisation (Fig. 2G).
Post-processing of decellularised tissues
Almost every tissue and organ such as small intestine (Refs Reference Parmaksiz, Elcin and Elcin84, Reference Ji85), oesophagus (Refs Reference Koch86, Reference Luc87), liver (Refs Reference Damania88, Reference Shimoda89), lung (Refs Reference O'Neill90, Reference Stabler91), dermis (Refs Reference Won92, Reference Ventura93), etc., have been decellularised until now. Historically, dECMs have been first used as patches/sheets or whole organs without a further breakdown in the dECM microstructure (Refs Reference Badylak94, Reference Wainwright95, Reference Elkins96). Although many solid dECMs products have received FDA approval and demonstrated promising results in preclinical and clinical studies (Ref. Reference Heath97), the majority have limitations concerning clinical utility. One of the important reasons is the implantation of scaffolds into tissue through invasive surgeries. This procedure may cause trauma in the area of interest and delay healing. Furthermore, solid scaffolds are not able to completely fill the defect areas. To facilitate the applicability of dECMs and widen the spectrum of the applications, injectable soluble dECMs have been developed by processing solid dECMs (Ref. Reference Freytes98). Injectable dECM are generally prepared through two approaches. The first approach is based on the generation of soluble dECM powder form, where lyophilised dECM is mechanically ground into a fine powder and then solubilised form is utilised. The second approach is based on the generation of dECM hydrogel form. For that purpose, the particulate ECM is digested by the acid-pepsin solution and then re-equilibrated to neutral pH and salt. Solubilised ECM can self-assemble into a nanofibrous hydrogel at physiological temperature (37 °C). It is worth to mention that during the solubilisation process, pepsin can disrupt the collagen fibre and completely change the composition, structural integrity and mechanical properties (Refs Reference Saldin99, Reference Mendibil100). ECM hydrogels can be used in many applications such as regenerative medicine, tissue engineering, 2D and 3D cell cultures, bioprinting, and electrospinning. In addition, ECM hydrogels serve as attractive substrates for 3D organoid culture to promote proliferation and differentiation of SCs as the in vivo microenvironment of SC niche can be accurately recapitulated (Refs Reference Giobbe101, Reference Vermeulen102).
Innovative applications of decellularised CNS structures for regenerative medicine
The role of dECM in tissue regeneration and repair process is of prime importance. In this context, it is possible to use biocompatible and biodegradable solid or soluble dECM formulations. One of the first studies on brain decellularisation focused on implantation of decellularised scaffolds to chick embryo chorioallantoic membrane to examine the angiogenic response induced by dbECM scaffolds. After implantation, the results showed that dbECM scaffolds induced a strong angiogenic response, comparable to fibroblast growth factor-2 (FGF-2), an angiogenic cytokine (Ref. Reference Ribatti71) (Fig. 3A). Different studies also showed that dbECM scaffolds support sustained ex vivo growth of different types of cells such as murine neural SCs (NSCs) (Ref. Reference De Waele103) (Fig. 3B) and Neuro2a cells (Ref. Reference Granato104) (Fig. 3C). In addition to the dbECM, decellularised spinal cord and cerebellar scaffolds have also used in tissue engineering applications. For instance, decellularised scaffolds from spinal cord with preserved 3D structure and composition were implanted in rats and the density of CD4+ and CD8+ cells that infiltrated the scaffolds after implantation was examined by immunohistochemical analysis. The results showed that dscECM was invaded by CD4+ and CD8+ cells to a much lesser extent than an allologous spinal cord graft, possibly due to an effective decellularisation protocol allowing the removal of myelin and cells that might have initiated an immune response after implantation (Ref. Reference Guo105). To evaluate the effect of the dscECM as an allograft on functional improvement in spinal cord injury-induced adult rats, dscECM scaffold was implanted alone and seeded with human umbilical cord (UC) blood-derived MSCs (hUCB-MSCs). The behavioural analysis indicated that, dscECM scaffold and dscECM scaffold + hUCB-MSCs provided significant locomotor recovery improvement. The scaffold supported hUCB-MSCs proliferation, as well as the migration of host neural cells into the graft. Furthermore, it is observed that myelinated axons could grow into the graft, promoting axonal regeneration at lesion sites (Ref. Reference Liu106). More recently, a decellularised cerebellum has been produced exhibiting in vitro and in vivo biocompatibilities. As cerebellum-derived ECM maintained a native microenvironment, seeded NSCs have been able to differentiate into neurons and astrocytes expressing cell-specific proteins, III-tubulin and glial fibrillary acidic protein, respectively (Fig. 3D). To assess immunogenicity, the dscECM scaffold was implanted subcutaneously on the dorsal side and intracranially into the frontal lobes of rats. After 4 weeks of implantation, low numbers of CD4+ or CD8+ monocytes were observed in all subgroups (Ref. Reference Zhu107).

Fig. 3. (A) Macroscobic images of the dbECM are surrounded by allantoic vessels after 12 days of incubation (Reprinted with permission from Ribatti et al., 2003. Copyright (2003) Elsevier) (Ref. Reference Ribatti71). (B) Confocal imaging of a 3D maze-like cellular structure in 3D dbECM section (Reprinted with permission from deWaele., 2015. Copyright (2014) Elsevier) (Ref. Reference De Waele103). (C) (a) Internal region of dbECM 24 h after recellularisation with Neuro2a cell. Scale = 50 μm. (b) Neuro2a cells cultures inside dbECM for 72 h. Marker for mature neuron (Tubulin beta 3; TUBB3; green) and nuclear staining (DAPI; blue) (Reprinted with permission from Granato et al., 2020. Copyright (2019) Elsevier) (Ref. Reference Granato104). (D) (a) Immunofluorescence staining for III-tubulin (b) and GFAP (Reprinted with permission from Zhu et al., 2015. Copyright (2015) Elsevier) (Ref. Reference Zhu107). (E) (a) PC12 cell migration rate in dbECM (b) and dubECM (Reprinted with permission from Crapo et al., 2012. Copyright (2012) Elsevier) (Ref. Reference Crapo42). (F) N1E-115 cell extension following 7 days culture in B-ECM in 3D cube (Reprinted with permission from Medberry et al., 2013. Copyright (2012) Elsevier) (Ref. Reference Medberry77). (G) Filamentous actin (F-actin) staining of pdGCs in pdECM and collagen matrices (Reprinted with permission from Koh et al., 2018. Copyright (2018) Springer Nature) (Ref. Reference Koh82). (H) (a) Immunocytochemical images of hippocampal (green) and (b) cortical neurons (green) in the hydrogels, stained for Tuj-1 for neurites and DAPI for nuclei. (c) H&E staining of the injured spinal cord tissue sample (control) and after implantation for 8 weeks with dbECM hydrogels. (d) H&E staining shows the change in cavity size (Reprinted with permission Hong et al., 2020. Copyright (2019) Elsevier) (Ref. Reference Hong80). (I) (a) The cell survival rate under the treatment of dbECM, bFGF and bFGF + dbECM. (b) The biocompatibility of dbECM with bFGF in rat brain after implantation for 15 days (Reprinted with permission from Lin et al., 2017. Copyright (2016) Elsevier) (Ref. Reference Lin78). (J) Growth of primary cortical rat neurons in 3D hydrogel shown by β-III tubulin staining for neurons (Reprinted with permission from Sood et al., 2016. Copyright (2015) American Chemical Society) (Ref. Reference Sood110). (K) Immunostaining of encapsulated primary cortical neurons in 3D scaffolds (β-tubulin staining (green) for neurites and DAPI staining (blue) for cell nuclei) (Reprinted with permission from Seo et al., 2020. Copyright (2019) American Chemical Society) (Ref. Reference Seo81). (L) (a) Schematic representation of a Michael-addition reaction used to form a hydrogel network. (b) Images of human primary astrocyte morphology in the different hydrogel conditions (Reprinted with permission from Galarza et al., 2020. Copyright (2020) WILEY) (Ref. Reference Galarza111).
To facilitate applicability of dECMs and widen the range of their applications in CNS studies, a method was developed to process decellularised porcine brain into a solubilised form (Ref. Reference Dequach76). In this study, the hydrogel was used as a coating material for culture of neurons, where human iPSC-derived neurons plated on the hydrogel expressed neuronal markers and showed neuronal morphology. Additionally, the solubilised brain matrix was reported to self-assemble into nanofibrous hydrogel upon injection in vivo. This milestone study demonstrated the feasibility of the solubilised brain matrix for cell culture studies as a coating material and tissue engineering applications as a hydrogel scaffold. To prepare the ideal ECM hydrogel form for CNS repair, both neuronal and non-neuronal tissues were prepared and evaluated in vitro and/or in vivo (Refs Reference Crapo28, Reference Crapo42, Reference Medberry77). In a study, the addition of solubilised optic nerve, brain and spinal cord ECMs to PC12 neuronal cultures resulted in induced proliferation, migration and differentiation in vitro (Ref. Reference Crapo42). On the contrary, solubilised urinary bladder ECM (non-CNS ECM) showed an inhibitory effect on PC12 cell migration rather than acting as a chemoattractant over the same concentration (Ref. Reference Crapo42) (Fig. 3E). Moreover, CNS ECM has been reported to induce differentiation from NSCs into neurons expressing bIII-tubulin in two-dimensional culture, whereas neurite extension in three-dimensional culture (Ref. Reference Crapo28). In another study, both CNS and non-CNS ECM (urinary bladder) hydrogels have been reported to increase the number of N1E-115 cells expressing neurites; however, only brain ECM increased neurite length (Ref. Reference Medberry77) (Fig. 3F). More recently, dbECM, dscECM and decellularised UC hydrogels have been shown to promote the migration of human MSCs and differentiation of NSCs, as well as axonal outgrowth in vitro (Ref. Reference Kočí108). However, only ducECM hydrogel could significantly improve the proliferation of tissue-specific UC-derived MSCs when compared with CNS ECMs (Ref. Reference Kočí108). All these results have shown that CNS ECMs provide tissue-specific effects on cell lines. Indeed, hydrogels prepared from dbECM have shown promising results in brain tissue remodelling and repair following traumatic brain injury (Ref. Reference Wu109).
Hydrogels derived from dECM have also been used in in vitro 3D tumour models. Such in vitro 3D models are of prime importance to determine the effect of tumour-specific ECM on the propagation mechanism of cancer cells. For instance, a 3D in vitro tumour model was developed by using a hydrogel derived from patient tissue-ECM (pdECM) to investigate the invasive characteristic of glioblastoma multiforme (GBM). In this study, the results showed that the disseminated GBM cells exhibited significantly different morphologies within the pdECM and collagen matrix (Fig. 3G) (Ref. Reference Koh82).
Subsequent to injection, the host response to hydrogel is critical in terms of success or failure in tissue-repair applications. Macrophages are vital in the host response to implanted biomaterials and have been reported as predictors of downstream tissue remodelling events. For instance, one of the studies has shown that porcine bECM hydrogel expressed a predominant M2-like macrophage phenotype, which is pro-remodelling and anti-inflammatory (Ref. Reference Dziki112). In another study, 3D hydrogels derived from adult porcine brain dECM powder and collagen I solution have been evaluated for neurite outgrowth of cortical and hippocampal neurons and reported to promote macrophage polarisation towards M2 phenotype for in vitro SCI model. Also, these brain dECM hydrogels were used as injectable biomaterials for in vivo rat SCI model, and shown to modulate the macrophages in the injured spinal cord for neuroregeneration (Ref. Reference Hong80) (Fig. 3H).
The delivery of therapeutics such as bioactive molecules, drugs and growth factors via 3D brain dECM scaffolds or brain dECM hydrogel-based nano/micro particular system is an important approach to stimulate cell-tissue growth for the regenerative medicine application and the treatment of CNS diseases. bFGF is a member of the FGFs and regulates important biological functions such as stimulating angiogenesis and neuroprotection (Ref. Reference Yafai113). However, bFGF has a short-half life in free form. To overcome this, CNS-derived rat brain dECM scaffold containing bFGF was used to enhance and extend the neuroprotective effect of bFGF on Parkinson's disease targeted therapy. The biocompatibility and neuroprotective effect of bFGF doped dECM were evaluated in vitro PC-12 cell culture model and in vivo rat model (Fig. 3I). In vivo study showed that the combination of dbECM and bFGF may be a promising and safe therapeutic strategy for Parkinson's disease as neural recovery was promoted (Ref. Reference Lin78). In a similar study, bFGF-dscECM scaffold complex was encapsulated into a heparin modified poloxamer (HP) solution to prepare a temperature-sensitive hydrogel (bFGF-dscECM-HP) (Ref. Reference Xu114). An in vitro cell survival study showed that the bFGF-dscECM-HP hydrogel promoted the proliferation of PC12 cells more in comparison to the bFGF solution. Glial scars were reported to be inhibited by bFGF-ASC-HP hydrogel and contributed to recovery through differentiation of the NSCs and regeneration of nerve axons. In another study, neurotrophin-3 that supports adhesion, proliferation and differentiation of rat BMSCs was cross-linked with dscECM to construct a sustained-release system, where 35 days of neurotrophin-3 release was achieved (Ref. Reference Xing115).
Within the scope of regenerative medicine applications, 3D composite hydrogel systems are one of the most commonly used decellularised brain tissues to mimic functional, structural and biochemical properties of native brain tissue, which might be further developed for neuroregeneration. A 3D bioengineered composite hydrogel model of cortical brain tissue was developed consisting of fibrous mesh-like dECM from foetal porcine brain, collagen I solution, silk scaffold and embryonic day 18 primary neurons, to provide a framework for studying axonal ingrowth of cortical neurons in vitro (Ref. Reference Sood110) (Fig. 3J). In a similar study, a 3D composite hydrogel mixing porcine brain dECM and collagen I, with/without encapsulated primary embryonic cortical neurons was reported to recapitulate the brain tissue microenvironment through increased neuronal differentiation/outgrowth and neuron-to-brain dECM interactions. Subsequent to microfibril alignment by stretching and releasing of the hydrogel-based chip to obtain anisotropically organised brain dECM, neurons incubated in this 3D platform were reported to exhibit enhanced neurite outgrowth and development compared to only collagen gels (Ref. Reference Seo81) (Fig. 3K). In another study, a spinal cord anatomy-inspired hyaluronic acid-ECM composite hydrogel was formulated with the core ECM derived from grey matter regions of the brain, whereas the shell from white matter regions (Ref. Reference Beachley25) and primary mouse ESCs were encapsulated in the hydrogel. The ESCs have been reported to survive, spread in the scaffold and differentiate into neuronal lineages, expressing nestin. To enhance the mechanical strength of ECM scaffolds and prevent rapid degradation by host enzymes such as collagenase and connective tissue proteases after a certain period of time, crosslinking has been applied in various studies. For instance, genipin-crosslinked rat dscECM enabled the structural integrity of the scaffolds to be preserved for 14 days in vitro (Ref. Reference Jiang116). In another study, a rat brain dECM incorporated genipin-crosslinked gelatin electrospun scaffold was developed to provide a suitable microenvironment for cell adhesion, proliferation, survival and differentiation potential towards neural precursor cells for nervous tissue regeneration (Ref. Reference Baiguera37). As a carboxyl- and amine-reactive cross-linker, EDC was also utilised to enhance the properties of dscECMs and provide adhesion and differentiation of rat BMSCs into neuron-like cells (Ref. Reference Xing117). With an innovative approach, a 3D synthetic brain hydrogel containing human brain dECM peptide mixture, enriched with crosslinked hyaluronic acid by linear PEG-dithiol was developed and functionalised with brain-specific integrin binding/matrix metalloprotease degradable peptide cocktail, which was shown to control astrocyte star-shaped morphologies and maintenance of astrocyte quiescence compared to collagen hydrogels (Ref. Reference Galarza111) (Fig. 3L).
The microfluidic-based organ-on-a-chip systems that mimic in vivo microenvironment by incorporating dECM may be useful to understand the extrinsic factors involved in the regulation of cell fate and function, and also for fast and cost-effective personalised drug screening studies (Ref. Reference Yesil-Celiktas118). With this regard, a patient-specific ex vivo glioblastoma-on-a-chip model was developed with a highly biomimetic ecosystem, which consisted of bioprinted patient-derived tumour cells and vascular endothelial cells with dbECM bioink, and yielded important cues for recapitulating the pathological features of glioblastoma, thus allowing for patient-specific drug susceptibility to be identified (Ref. Reference Yi119). The results suggested that tumour-on-a-chip model containing dbECM bioink was an option for modelling personalised cancer treatments and for drug screening to guide clinical decisions that may overcome refractory cancers. In another study, human brain dbECM-based cell culture system was used to facilitate plasmid-transfection-based direct conversion of primary mouse embryonic fibroblasts into induced neuronal cells. The results showed that microfluidic system combined with the 3D dbECM hydrogel was able to closely mimic in vivo conditions for neuronal reprogramming (Ref. Reference Jin120). As for drug screening, a 3D biomimetic macro-porous scaffold was fabricated by incorporating hyaluronic acid, porcine brain ECM and growth factors to create a patient-derived xenograft model by using primary glioma SCs (GSCs). The platform has been reported to regenerate primary gliomas and used for screening novel siRNA nanotherapeutics to inhibit the tumorigenic potential of GSCs with current clinical drug, temozolomide and an anticancer phytochemical, nanocurcumin as a control (Ref. Reference Jeena121). For potential drug screening/toxicity assays, human brain organoids are clinically relevant and scalable models. In a recent study, porcine brain dECM hydrogels provided similar gene expression and differentiation of ESCs similar to those grown in matrigel outcomes and suggested as an alternative scaffold for human cerebral organoid formation (Ref. Reference Simsa122).
Challenges and future prospects
The combination of decellularisation techniques with complex biology has enabled the development of advanced applications in regenerative medicine and neural tissue engineering. Current decellularisation methods have been successful to a certain extend. For instance, chemical and biological methods might lead to degradation of organ-specific ECM components, whereas physical methods such as supercritical CO2 and electroporation can yield insufficient and non-homogeneous decellularisation when applied alone. Thus, a combination of different techniques can be consecutively applied to preserve the ECM niche. Along with the decellularisation method, the sterilisation method also affects the biochemical composition, ultrastructure and mechanical properties of decellularised matrix-based biomaterials. Thus, comprehensive investigation of appropriate sterilisation techniques for dbECM and dsECM-based biomaterials is required. Studies conducted to date have shown that dbECM and dscECM-based biomaterials are biocompatible, angiogenic, able to regulate various cell behaviours such as differentiation and migration. Furthermore, these biomaterials provide in vitro and in vivo controlled release of growth factors, promote neural recovery and neuroprotection by exhibiting low immunogenicity subsequent to in vivo implantation. In the next years, the use of dbECM and dscECM-based hydrogels with 3D printing technology may accelerate the development of therapeutic solutions. Although the low viscosity and poor mechanical properties of dbECM and dscECM-based hydrogels limit their use in 3D bioprinting technology, the combination with well-known synthetic or natural hydrogels may enable the printing. Given the significance of brain- and spinal cord-ECM microenvironment, development of therapeutic solutions will be expedited by post-processing of dECM and eventually, new opportunities are envisaged for the treatment of neuronal diseases and injuries.
Author contributions
BY: conceptualisation, writing – original draft, visualisation. PSM: conceptualisation, writing – review and editing, visualisation. OYC: conceptualisation, review and editing, supervision, project administration, funding acquisition. No datasets were generated or analysed during the current study.
Financial support
This study was supported by The Scientific and Technological Research Council of Turkey (TUBITAK) under grant number 119M578 and Research Fund of Ege University as an International Research Cooperation Project under grant number FUA-2020-22187.
Declaration of competing interest
The authors declare that they have no known competing financial interests or personal relationships that could have appeared to influence the work reported in this paper.
Conflict of interest
None.