Excessive intake of a Western-style diet rich in SFA and n-6 long-chain PUFA (LCPUFA) and poor in n-3 LCPUFA is one of the major factors promoting the onset of obesity( Reference Simopoulos 1 , Reference Ravussin and Kozak 2 ). In contrast, EPA (20 : 5n-3) and DHA (22 : 6n-3) acids were reported, more than just once, as having weight-reducing properties( Reference Calder 3 – Reference Todorčević and Hodson 5 ). The physiological activity of n-3 LCPUFA may depend on their specific lipid structure. Although not consensual, the TAG form seems to be more bioavailable and, therefore, better absorbed and assimilated than the ethyl esters (EE) form( Reference Bandarra, Lopes and Martins 6 ).
Adipose tissue plays a central role in regulating energy balance through its metabolic, cellular and endocrine functions. It has been classified into anabolic white adipose tissue (WAT) and catabolic brown adipose tissue (BAT)( Reference Lee, Mottillo and Granneman 7 ). However, certain differentiated adipocytes can undergo a process known by ‘browning’, switching its metabolic phenotype from typical WAT to those resembling BAT and altering between anabolic and catabolic states( Reference Lee, Mottillo and Granneman 7 ). Subcutaneous WAT and visceral WAT are metabolically distinct. Subcutaneous fat expands predominantly by hyperplasia and appears protective through enhanced adipogenic capacity( Reference Park, Lee and Cheon 8 ). In opposition, visceral fat expansion occurs mainly by hypertrophy with great infiltration of macrophages( Reference Barbatelli, Murano and Madsen 9 ). Visceral adipose tissue activity is also regulated by a great number of glucocorticoid receptors β-adrenoceptors and a lower number of insulin receptors( Reference Capurso and Capurso 10 , Reference Fruhbeck, Mendez-Gimenez and Fernandez-Formoso 11 ). Hence, visceral fat accumulation is important in clinical terms because it is more closely associated with the metabolic syndrome. Conversely, BAT constitutes a metabolically active tissue responsible for non-shivering thermogenesis and depletion of excess energy content( Reference Birerdinc, Jarrar and Stotish 12 ).
At birth, human newborns have a considerable amount of BAT in their body (1–5 % total body weight, meaning a mass of 35–200 g), which is implicated in heat generation, whereas skeletal muscle is not able to make any movements to produce heat( Reference Virtanen and Nuutila 13 ). In puberty, this function declines, and is associated with sex-dependent hormones. Recently, BAT was also found to be functionally and metabolically active in adult humans( Reference Virtanen, Lidell and Orava 14 ), and it is more developed in people exposed to continuous cold, such as outdoor workers( Reference van Marken Lichtenbelt, Vanhommerig and Smulders 15 ). In fact, in adults, BAT can amount to <100 g in average, which might seem few, but when activated it can be able to consume 3–4 kg of WAT in 1 year( Reference Virtanen, Lidell and Orava 14 ). Consequently, BAT appears to be preventive against metabolic disorders associated with obesity( Reference Smorlesi, Frontini and Giordano 16 ). In fact, the differential expression of brown adiposity phenotype may, in part, explain why some individuals are more prone to obesity than others. It actually underlies the new concept of metabolically healthy obesity.
Aquaporins (AQP) are a family of transmembrane protein channels that facilitate the permeation of water and small solutes, such as glycerol, across cell membranes( Reference Agre 17 , Reference Verkman 18 ). According to their primary sequence and permeability characteristics, AQP are divided into three sub-groups: (i) orthodox or classical AQP (AQP0, AQP1, AQP2, AQP4, AQP5, AQP6 and AQP8), primarily water selective( Reference Agre 17 , Reference Verkman 18 ); (ii) aquaglyceroporins (AQP3, AQP7, AQP9 and AQP10), permeable to small uncharged solutes, such as glycerol and urea in addition to water( Reference Agre 17 – Reference Rojek, Praetorius and Frøkiaer 19 ); (iii) unorthodox or S-AQP (AQP11 and AQP12), found in intracellular membranes, with lower sequence similarity to the other mammalian AQP and suggested as permeable to water (AQP11 and AQP12) and glycerol (AQP12)( Reference Ishibashi, Tanaka and Morishita 20 , Reference Madeira, Fernández-Veledo and Camps 21 ).
In the adipose tissue, some AQP isoforms may have a pivotal role in controlling fat accumulation( Reference Fruhbeck 22 , Reference Rodríguez, Catalan and Gomez-Ambrosi 23 ) and have been implicated in obesity and related metabolic complications( Reference da Silva, Rodrigues and Rebelo 24 ). Among the various mammalian aquaglyceroporins, AQP7 is the most representative glycerol channel and was the first to be detected in human and mouse adipose tissue( Reference Kishida, Kuriyama and Funahashi 25 , Reference Kondo, Shimomura and Kishida 26 ). Impaired glycerol transport through AQP-7 (an aquaglyceroporin) leads to glycerol retention within adipose tissue, ultimately leading to acceleration of TAG synthesis and accumulation, which has been correlated to obesity development( Reference Kishida, Kuriyama and Funahashi 25 , Reference Hibuse, Maeda and Funahashi 27 , Reference Madeira, Camps and Zorzano 28 ). However, the presence of other aquaglyceroporins in addition to AQP7 has been more recently reported(23, Reference Laforenza, Scaffino and Gastaldi 29 ). AQP3 was identified in both subcutaneous and visceral human fat depots but with a stronger expression in the stromal vascular tissue adjacent to adipose( Reference Rodríguez, Catalan and Gomez-Ambrosi 23 , Reference Rodriguez, Catalan and Gomez-Ambrosi 30 , Reference Miranda, Escote and Ceperuelo-Mallafre 31 ). Interestingly, AQP3 was the isoform with higher expression in human visceral adipose tissue followed by AQP9 and AQP7, with AQP3 gene expression being found in both fully mature adipocytes and stroma vascular fraction (SVF) cells. AQP3 is highly expressed in preadipocytes, macrophages and other cells within the SVF (leucocytes, neutrophils, erythrocytes and fibroblasts). Similar to AQP7, AQP3 is up-regulated by catecholamines, suggesting that, together with other aquaglyceroporins, it may be relevant to regulate paracellular transport of glycerol between the bloodstream and the interstitium of WAT( Reference Rodríguez, Catalan and Gomez-Ambrosi 23 , Reference da Silva, Rodrigues and Rebelo 24 ).
More recently, our group detected the expression of AQP5 in 3T3-L1 adipocytes and showed that besides a role in water transport AQP5 depletion reduces lipid droplets content and impairs adipocyte differentiation( Reference Madeira, Mósca and Moura 32 ). However, AQP5 involvement in the obesity mechanisms and the differential expression of these AQP isoforms in WAT (subcutaneous and visceral) and BAT remains unclear.
In this work, we investigated the variability of AQP (AQP3, AQP5, AQP7) gene expression across subcutaneous WAT, visceral WAT and interscapular BAT, which might be associated with adipose tissue depot’s own location and their different metabolic functions. In addition, to further investigate whether the body fat reduction properties of n-3 LCPUFA in vivo are correlated with AQP expression levels in the adipose tissue, we compared the AQPs’ transcriptional profile of WAT (subcutaneous and visceral) and BAT from hamsters fed diets containing specific n-3 LCPUFA molecular structures: fish oil (FO, rich in EPA and DHA in the TAG form) and FO–EE (rich in EPA and DHA in the EE form), v. linseed oil (LSO, rich in α-linolenic acid (ALA), 18 : 3n-3) used as control. The expression of some markers of lipid metabolism, namely adiponectin (ADIPO), leptin (LEP), GLUT4, PPARα and PPARγ, was also characterised in the different adipose tissue samples.
Methods
Ethics statement
The experimental procedures were reviewed by the Ethics Commission of CIISA/FMV and approved by the Animal Care Committee of the National Veterinary Authority (Direcção Geral de Alimentação e Veterinária, Portugal), following the appropriate European Union guidelines (2010/63/EU Directive).
Animals and diets
Besides having BAT in considerable amounts owing to hibernation, the lipid metabolism of hamsters is more similar to humans than mice and rats( Reference Yin, Carballo-Jane and McLaren 33 , Reference Dalboge, Pedersen and Hansen 34 ), thus making it possible to extrapolate results to humans.
In all, 10-week-old Golden Syrian male hamsters were purchased from Charles River (Charles River Laboratories). Hamsters were housed one per cage under a 14/10-h light/dark cycle schedule, synchronised with natural daylight, with controlled temperature of 20–24°C in a certified facility at the Faculty of Veterinary, University of Lisbon. During the first week, all animals were fed a standard diet to minimise stress and stabilise all metabolic conditions. After this period, twenty-four hamsters were assigned to three body-weight-matched groups, with eight animals each: the LSO group, rich in ALA, without EPA or DHA, taken as the control group; the FO group, a commercially available oil rich in TAG with EPA and DHA; and the FO–EE group. The final sum of EPA and DHA was identical across FO and FO–EE dietary treatments( Reference Bandarra, Lopes and Martins 6 , Reference Lopes, Bandarra and Martins 35 ). Diets were manufactured by the Experimental Diets Unit from the University of Almería in Spain. The detailed composition of the experimental diets, in terms of proximate chemical composition and fatty acids, has been published elsewhere( Reference Bandarra, Lopes and Martins 6 ). Briefly, all diets were based on AIN-93M formulation with modified lipid fractions. Each diet contained the following (g/100 g feed): casein (14·0), maize starch (46·6), maltodextrin (15·5), sucrose (10·0), cellulose (5·0), soyabean oil (4·0), l-cystine (0·18), AIN-93 mineral mix (3·5), AIN-93M vitamin mix (1·0), choline bitartrate (0·25) and tert-butylhydroquinone (0·0008).
Throughout the trial, hamsters had free access to water and food. Body weight and feed intake were recorded twice a week. After 12 weeks of feeding trial, hamsters were fasted for 12 h, weighed before and after the fasting period and euthanised by a mechanical–physical method. Hamsters were placed in a chamber and anaesthetised using a mixture of 20 % of isofluorane in propylene glycol (v/v) for 30 s( Reference Itah, Gitelman and Davis 36 ), followed by decapitation with a small animal guillotine, in certified ethical conditions to minimise animal suffering. Subcutaneous and visceral WAT from hamsters’ pelvic and retroperitoneal anatomical regions, respectively, as well as BAT from hamsters’ interscapular region were excised, weighed and stored at −80°C for subsequent gene expression determination.
Adipocyte RNA extraction and complementary DNA synthesis
Total RNA was isolated and purified from subcutaneous WAT, visceral WAT and BAT using a Qiagen RNeasy lipid tissue mini kit (Qiagen) and stored at −80°C. To exclude possible DNA contamination, on-column DNA digestion with the RNase-free DNase set (Qiagen) was performed. All procedures were based on the manufacturer’s protocol. The RNA concentration was determined spectrophotometrically at 260 nm using the NanoDrop1 ND-2000c (ThermoFisher Scientific). The ratios 260/280 and 260/230 nm were also determined to assess the purity of RNA samples and the presence of contaminants. Only samples that fulfill the established quality parameters (260/280 and 260/230 nm ratios were used as purity measurements for protein and solvent presence, respectively, considering ratios between 1·8 and 2·2) proceeded to complementary DNA (cDNA) synthesis step. To generate cDNA for quantitative PCR, 750 ng of total RNA was reverse-transcribed for 2 h at 37°C using the first-strand cDNA synthesis kit (NZYtech). Specific primers for real-time quantitative PCR were designed for seven genes (AQP3, AQP5, AQP7, ADIPO, GLUT4, PPAR α and PPAR γ). The DNA sequences of the golden hamster (Mesocricetus auratus) genes were obtained from GenBank and then submitted to the Primer3 software (http://primer3.ut.ee/) to generate primers. Primer3 parameters were set in order to obtain the best pair of primers in size (20–27 bp), melting temperature (50–65°C), % GC (50–60 %) and product size range (75–200 bp). The characterisation of the selected genes used in real-time quantitative PCR is described in Table 1.
Table 1 Gene-specific primer sequences used for real-time-quantitative PCR

Real-time quantitative PCR
Real-time quantitative PCR was performed using PowerUp™ SYBR® Green Master Mix (Life Technologies) and Xpert Fast SYBR (GRiSP) to amplify AQP (AQP3, 5 and 7) and markers of lipid metabolism (ADIPO, LEP, GLUT4, PPAR α and PPAR γ), respectively. The final reaction volume of 20 μl was prepared using 10 μl of SYBR master mix, 3 μl of template cDNA, 2 μl of forward and reverse primers and 3 μl of molecular-biology-grade water. The reaction was performed on a CFX96™ Real-Time PCR Detection System C1000 (BioRad) consisting of an initial denaturation step at 95°C for 3 min, forty-five cycles of denaturation at 95°C for 10 s and annealing/extension at 59 or 62°C (for AQP and markers of lipid metabolism, respectively) for 30 s. The relative expression levels were normalised to reference genes (glyceraldehyde-3-phosphate dehydrogenase (GAPDH) and β-actin (ACTB) for AQP3, AQP5 and AQP7; ribosomal protein L27 (RPL27) for ADIPO, LEP, GLUT4, PPAR α and PPAR γ) and calculated using a variation of the Livak method( Reference Livak and Schmittgen 37 ), corrected for variation in amplification efficiency, as described by Fleige & Pfaffl( Reference Fleige and Pfaffl 38 ).
Statistical analysis
Statistical analyses were carried out with the Statistical Analysis System software, version 9.4 (SAS Institute). All data were presented as means with their standard errors. Data were checked for normal distribution by Kolmogorov–Smirnov test and variance homogeneity using Proc MIXED with a model including the fat depot and diet as fixed effects and the repeated statement considering the group option to accommodate the variance heterogeneity. This analysis was followed by Tukey’s multiple comparisons test. Pearson’s correlation coefficients were calculated with the Proc CORR procedure to establish linear relationships among gene expression. P<0·05 was considered to be statistically significant.
Results
Effect of fat depot on gene expression levels of aquaporins and markers of lipid metabolism
In quantitative terms and comparing the transcriptional profile of AQP3, AQP5 and AQP7 in hamster’s fat tissues, the levels of AQP3 mRNA were predominant over AQP7 and AQP5 (AQP3>AQP7>AQP5) in all fat depots. A clear effect of fat depot was observed for AQP3 and some markers of lipid metabolism, such as LEP and PPAR α, meaning that, regardless of the dietary treatment, the transcriptional profile of this gene was divergent among adipose tissues. This effect of fat depot is most certainly associated with the lower transcriptional profile of AQP3 in BAT relative to subcutaneous (P<0·001) and visceral WAT (P<0·001) (Fig. 1). Identical variations were found for LEP in BAT relative to subcutaneous (P<0·001) and visceral (P<0·001) WAT, and also between WAT (P=0·007) (Fig. 2). Conversely, PPAR α is highly expressed in BAT in comparison with residual levels of mRNA expression found in both subcutaneous (P<0·001) and visceral (P<0·001) WAT (Fig. 3). The variation pattern between subcutaneous and visceral WAT is similar for some genes, including AQP3, AQP5, AQP7 and LEP (Figs 1 and 2, P>0·05).

Fig. 1 Effect of fat depot, diet and diet×fat depot interaction on the relative expression levels of aquaporin-3, aquaporin-5 and aquaporin-7 in the subcutaneous white adipose tissue (sWAT, white bars), visceral white adipose tissue (vWAT, gray bars) and brown adipose tissue (BAT, black bars) from hamsters fed linseed oil (LSO), fish oil (FO) and FO–ethyl esters (EE). Values are means, with their standard errors represented by vertical bars. a,b,c,d Mean values with unlike letters were significantly different (Tukey’s post hoc, P<0·05).

Fig. 2 Effect of fat depot, diet and diet×fat depot interaction on the relative expression levels of adiponectin and leptin in the subcutaneous white adipose tissue (sWAT, white bars), visceral white adipose tissue (vWAT, gray bars) and brown adipose tissue (BAT, black bars) from hamsters fed linseed oil (LSO), fish oil (FO) and FO–ethyl esters (EE). Values are means, with their standard errors represented by vertical bars. a,b,c,d,e Mean values with unlike letters were significantly different (Tukey’s post hoc, P<0·05).
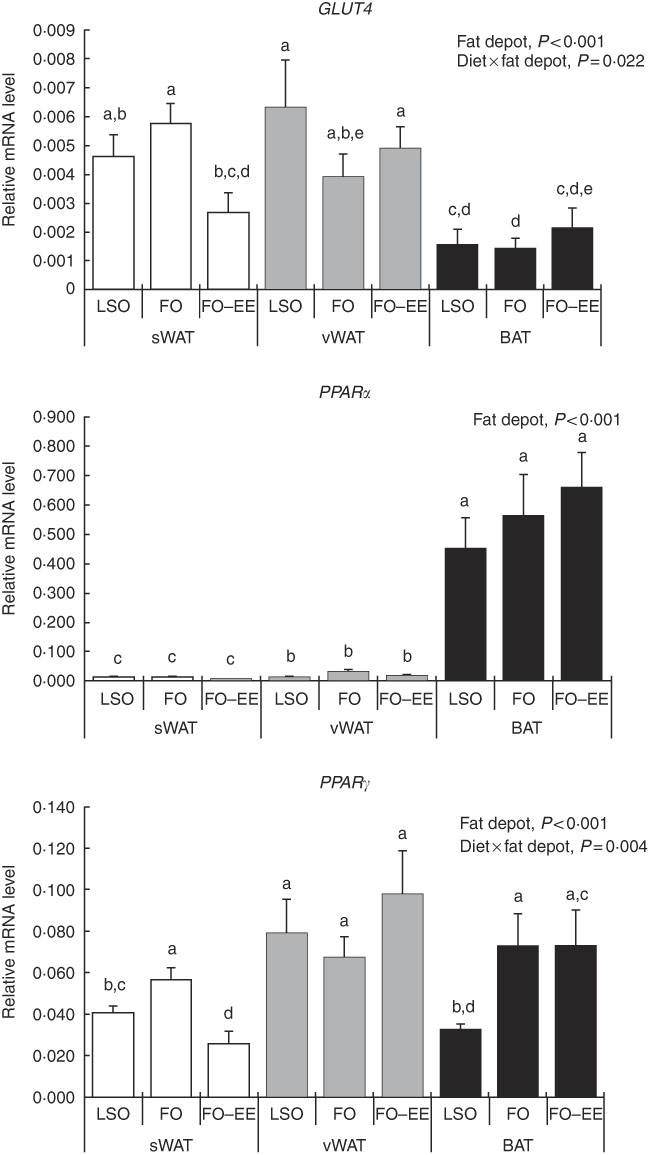
Fig. 3 Effect of fat depot, diet and diet×fat depot interaction on the relative expression levels of GLUT4, PPARα and PPAR γ in the subcutaneous white adipose tissue (sWAT, white bars), visceral white adipose tissue (vWAT, gray bars) and brown adipose tissue (BAT, black bars) from hamsters fed linseed oil (LSO), fish oil (FO) and FO–ethyl esters (EE). Values are means, with their standard errors represented by vertical bars. a,b,c,d,e Mean values with unlike letters were significantly different (Tukey’s post hoc, P<0·05).
Effect of diet on gene expression levels of aquaporins and markers of lipid metabolism
The only gene affected solely by diet was AQP7. In fact, increased mRNA levels of this AQP were found in hamsters fed FO relative to LSO (P=0·008) but not in hamsters fed FO–EE relative to LSO (P=0·050), suggesting a positive effect of EPA and DHA when combined as TAG in AQP7 gene expression (Fig. 1). No changes were detected for AQP7 gene expression between FO and FO–EE-fed hamsters (Fig. 1, P=0·249).
Interaction effect of fat depot×diet on gene expression levels of aquaporins and markers of lipid metabolim
The genes for AQP5, ADIPO, GLUT4 and PPAR γ were affected by the interaction between diet and fat, as illustrated in Figs 1–3. An interaction effect between diet and fat was found for the expression level of AQP5 (Fig. 1, P=0·031). The reason for this is that BAT presented increased mRNA levels of AQP5 than subcutaneous and visceral WAT when hamsters were fed an FO–EE diet. For ADIPO, higher mRNA levels were observed in the FO diet relative to FO–EE in subcutaneous WAT and BAT, whereas the inverse was found for visceral WAT (Fig. 2, P<0·001). GLUT4 was also affected by an interaction between diet and fat (Fig. 3, P=0·022), which is explained by decreased mRNA levels in the FO–EE diet in subcutaneous WAT, not verified in BAT and visceral WAT. For PPAR γ, hamsters fed FO–EE had lower mRNA levels than FO in subcutaneous WAT but identical in visceral WAT and BAT, justifying the interaction detected between diet and fat (Fig. 3, P=0·004).
Pearson’s correlation coefficients among genes per fat depot
Table 2 presents the Pearson’s correlation coefficients (r) for gene expression levels in subcutaneous WAT, visceral WAT and BAT. It is noteworthy that that all positive correlations found were moderate (0·7≥r≥0·3). In subcutaneous WAT, AQP3 was positively correlated with ADIPO (P<0·01) and AQP7 (P<0·01). ADIPO correlated positively with PPAR γ (P<0·01), PPAR α (P<0·01) and LEP (P<0·01). A positive correlation was observed between GLUT4 and PPAR γ (P<0·001), GLUT4 and PPAR α (P<0·05) and between PPAR α and PPAR γ (P<0·01) (Table 2). In visceral WAT, fewer correlations were found: AQP3 was positively correlated with AQP7 (P<0·05) and also with AQP5 (P<0·05) (Table 2). In BAT, AQP7 correlated positively with PPAR γ (P<0·05) and LEP (P<0·05). ADIPO was correlated with both PPAR γ (P<0·01) and LEP (P<0·05). PPAR α correlated with both GLUT4 (P<0·05) and PPAR γ (P<0·01) (Table 2).
Table 2 Pearson’s correlations coefficients among gene expression levels in subcutaneous white adipose tissue, visceral white adipose tissue and brown adipose tissue

LEP, leptin; ADIPO, adiponectin; AQP, aquaporins.
* P<0·05, ** P<0·01, *** P<0·001.
Discussion
AQP are membrane protein channels recognised as important players in controlling fluid and fat homoeostasis. Although AQP7 has been reported to be involved in obesity, the role of other AQP in fat-related metabolic disorders is still unclear. Moreover, their differential expression in WAT and BAT, as well as their modulation by dietary n-3 LCPUFA, such as EPA and DHA in distinct molecular structures, has never been investigated.
In this experimental trial, hamsters’ final body weight and weight gain did not vary among dietary groups( Reference Bandarra, Lopes and Martins 6 ), as well as adipose tissue weight from subcutaneous WAT, visceral WAT and interscapular BAT( Reference Lopes, Bandarra and Martins 35 ), as all diets contained the same amount of fat. These data agree with similar values of hamsters’ daily feed intake. The abundance of n-3 LCPUFA in human fat does not increase notably even after supplementation( Reference Baylin, Kabagambe and Siles 39 ). EPA and DHA are not particularly stored long-term in TAG; rather, they may be partitioned to oxidation pathways or storage in other lipid fractions, such as plasma phospholipids, cell phospholipids and erythrocytes, all of them having a notably higher abundance of both EPA and DHA than adipose tissue( Reference Todorčević and Hodson 5 , Reference Bandarra, Lopes and Martins 6 , Reference Lopes, Bandarra and Martins 35 ).
AQP5 is a classical water channel, whereas AQP3 is an aquaglyceroporin that facilitates permeation of glycerol in addition to water( Reference Madeira, Moura and Soveral 40 ). As expected, we found higher levels of AQP3 expression in WAT in relation to BAT, possibly owing to the main role of WAT in energy storage in the form of TAG. In BAT, AQP3 expression was slightly decreased, although not reaching statistical significance, when hamsters were fed the FO–EE diet, which probably means that the EE molecular form promotes less glycerol efflux in brown adipocytes in comparison with TAG. Interestingly, when comparing the transcriptional profile of both AQP, an inverse pattern of variation was found for AQP3 and AQP5 between FO and FO–EE diets in BAT. Although AQP5 does not contribute to glycerol fluxes, it was shown to be important for adipocyte differentiation( Reference Madeira, Mósca and Moura 32 ), and thus a complementary role of these two isoforms for intracellular TAG accumulation cannot be ruled out.
Among the various mammalian aquaglyceroporins, AQP7 is the most representative glycerol channel and was the first to be detected in the adipose tissue from human and rodents( Reference da Silva and Soveral 41 , Reference Fruhbeck, Catalan and Gomez-Ambrosi 42 ), although predominance of AQP3 in human visceral adipose tissue has been reported( Reference Rodriguez, Catalan and Gomez-Ambrosi 30 ). In the adipose tissue from hamsters the higher expression was found for AQP3, rather than for AQP7. In the overall picture, AQP7 expression was only affected by diet, with higher levels of mRNA found in the FO dietary treatment in comparison with LSO and FO–EE, suggesting that EPA and DHA when combined as TAG activate the efflux of glycerol from the adipocytes via AQP7, possibly leading to a positive decrease in lipid droplets size. Curiously, the expression of AQP7 was kept unchanged in all three adipose tissues (there was no fat depot effect), confirming its conservative role in glycerol release from adipocytes that ensure lipid homoeostasis( Reference da Silva and Soveral 41 ).
ADIPO has emerged as the most abundant adipocyte product, thereby redefining adipose tissue as a key component of the endocrine system( Reference Cao 43 ). In obesity, the circulating levels of ADIPO fall while LEP levels rise, suggesting that the regulation of these two adipocyte-derived hormones may be simultaneously influenced by common obesity-related factors( Reference Bastard, Maachi and Lagathu 44 ). In fact, ADIPO and LEP are adipokines responsible for normal adipocyte phenotype and function( Reference Cowherd, Lyle and McGehee 45 ). The regulation pattern of gene expression of ADIPO in subcutaneous and visceral WAT was similar, perhaps subjective to common control of energy balance. The same does not apply for LEP. LEP administration has been shown to down-regulate AQP7 expression in murine subcutaneous adipocytes(46). We found no correlation (positive or negative) between LEP and AQP7 mRNA levels in subcutaneous WAT, but instead a positive correlation in BAT that deserves further investigation. In BAT, both ADIPO and LEP showed lower expression, indicating that ADIPO and LEP have in WAT their major contributor. In fact, WAT is known to be the most relevant tissue that accounts for endocrine factors, such as ADIPO and LEP in the bloodstream( Reference Wang, Zhao and Lin 47 ). However, ADIPO production by BAT, although in lower amounts, is well documented( Reference Zhang, Matheny and Zolotukhin 48 , Reference Iacobellis, Di Gioia and Petramala 49 ). It is now evident that the LEP synthesis also takes place in BAT, although the physiological role of LEP produced by brown fat is still unclear.
Conversely, PPAR α was highly expressed in BAT, which certainly relates to fatty acid β-oxidation( Reference Kersten, Desvergne and Wahli 50 ) occurring in brown adipocytes as BAT is primarily a thermogenic organ. In turn, PPARγ stimulates glycerol transport and glycerol phosphorylation( Reference Guan, Li and Jensen 51 , Reference Tordjman, Khazen and Antoine 52 ). Moreover, AQP7 is a direct PPARγ target gene in adipocytes( Reference Guan, Li and Jensen 51 , Reference Kishida, Shimomura and Nishizawa 53 ). This evidence is not supported by the moderate positive correlation found between AQP7 and PPAR γ mRNA levels in BAT, rather than what would be expected to be found in WAT. Furthermore, we have demonstrated that, in subcutaneous WAT, PPAR α and PPAR γ expression correlated significantly with the expression of GLUT4, which is an essential gene in the lipogenesis pathway( Reference Cowherd, Lyle and McGehee 45 ). These findings demonstrate a relationship between PPAR expression and the expression of other genes of lipid metabolism, and support the concept that PPAR α and PPAR γ activators may regulate fatty acid metabolism in the adipose tissue.
Variations of response across fat depots (subcutaneous WAT, visceral WAT and BAT) suggest that the features of adipose tissue responsible for adipocyte cells and differentiation may not be homogeneous, both in nature and distribution( Reference Peirce, Carobbio and Vidal-Puig 54 ). Visceral fat accumulation, as supported by PPAR γ up-regulation, which is the key regulator of adipocytes differentiation, is important in clinical terms because it is more closely linked to the metabolic syndrome owing to its inflammatory features. Conversely, brown adipocytes constitute a metabolically active tissue responsible for non-shivering thermogenesis, depletion of excess energy content being less inflammatory than WAT. These features are in line with PPAR α, ADIPO and LEP gene variations. BAT is associated with metabolic health owing to its oxidative capacity and appears to be effective in the protection against metabolic disorders associated with obesity and diabetes. In addition, the structure of combined EPA and DHA as EE reduced the gene expression of ADIPO, GLUT4 and PPAR γ in subcutaneous WAT, as well as ADIPO in BAT. The only exception was ADIPO, whose mRNA levels were found to be increased in the FO–EE diet relative to FO in visceral WAT. On the basis of the current research, the physiological activity of n-3 LCPUFA and, in particular, the potential effect of a specific fatty acid depends both on its structure and administration form. There seems to be a difference in the apparent bioavailability of n-3 LCPUFA, such that the TAG form is more bioavailable than the EE form( Reference Lawson and Hughes 55 – Reference Beckermann, Beneke and Seitz 57 ). This interpretation is in line with our own findings. TAG define three fatty acid esterified (bonded) to a glycerol backbone and is the natural molecular form that makes up virtually all fats and oils in both animal and plants species, devoid of toxicological effects and more chemically stable than EE( Reference Song, Inoue and Miyazawa 58 , Reference Yoshii, Furuta and Siga 59 ). The EE form lacks the glycerol backbone needed to reassemble the TAG structure. We speculate that this could be the major obstacle to the efficient absorption of EE form EPA and DHA, impairing in due course the transcriptional profile of these genes.
Conclusions
In this study, we reported differential AQP3, AQP5 and AQP7 gene expression across subcutaneous WAT, visceral WAT and interscapular BAT, which may reflect adipose tissue depot’s own location and metabolic function. We also found different patterns of AQP and markers of lipid metabolism expression between WAT (subcutaneous and visceral) and BAT when hamsters were fed specific n-3 LCPUFA molecular structures: FO (rich in EPA and DHA in the TAG form) and FO–EE (rich in EPA and DHA in the EE form). Among the AQP isoforms, AQP7 stands out as the more promising target for developing new anti-obesity drugs owing to its conservative role across WAT and BAT.
Acknowledgements
The authors are grateful to Susana V. Martins for technical assistance in primer use and validation.
This study was supported by the Spanish Ministry of Science and Innovation through ‘Structured lipids: novel dietary strategies for improving human health’ grant (AGL/25807/2011) and by Fundação para a Ciência e a Tecnologia, Portugal, through projects UID/CVT/00276/2013 and 2016 INOV-20 to CIISA, and UID/DTP/04138/2013 to iMed.ULisboa. I. V. d. S. is the recipient of a PhD fellowship (PD/BD/113634/2015) and M. S. M. of a post-doctoral fellowship (SFRH/BPD/97432/2013). P. A. L. is a post-doctoral researcher from Portugal 2020 project (08/SI/3399/2015).
P. A. L. and M. S. M. performed the animal experiment and tissue sampling. I. V. d. S. and R. M. performed the laboratory work. M. S. M. was responsible for statistics. P. A. L. and G. S. were responsible for interpretation of the results and preparation of the manuscript. J. A. M. P. was responsible for the study design. All the authors read and approved the findings of the study.
The authors declare that there are no conflicts of interest.