Folate is required for C1 metabolism and is thus essential for important biological pathways including DNA and RNA biosynthesis and methylation reactions. The functional effects of folate within the C1 network involve close interaction with vitamin B12, vitamin B6 and riboflavin. This review will address why these B vitamins are key in human health and how optimal status can be achieved. To illustrate the role of these metabolically interrelated vitamins through the lifecycle, case studies highlighting their health impacts at early, middle and late life will be reviewed. The significant public health challenges in achieving optimal nutritional status, and thus potentially preventing folate-related disease, will also be considered.
Functional role of folate and metabolic interaction with other B vitamins
Folates function as cofactors within C1 metabolism (Fig. 1). This involves the transfer and utilisation of C1 units in a network of pathways required for DNA and RNA biosynthesis, amino acid metabolism and methylation processes. In order to function effectively within this network, folate interacts closely with vitamin B12, vitamin B6 and riboflavin(Reference Bailey, Stover and McNulty1). Reduced folates enter the C1 cycle as tetrahydrofolate (THF) which acquires a carbon unit from serine in a vitamin B6-dependent reaction to form 5,10 methyleneTHF. This cofactor form, in turn, is either converted to 5 methylTHF or serves as the C1 donor in the synthesis of nucleic acids, where it is required by thymidylate synthetase in the conversion of deoyxuridine (deoxyuridine monophosphate) to deoxythymidine (deoxythymidine monophosphate) for pyrimidine biosynthesis, or is converted to other folate cofactor forms required for purine biosynthesis. Methylenetetrahydrofolate reductase (MTHFR) is a riboflavin-dependent enzyme that catalyses the reduction of 5,10 methyleneTHF to 5 methylTHF. Once formed, 5 methylTHF is used by methionine synthase for the vitamin B12-dependent conversion of homocysteine to methionine and the formation of THF. Methionine is activated by ATP to form S-adenosylmethionine (SAM), which then donates its methyl group to more than 100 methyltransferases for a wide range of substrates such as DNA, hormones, proteins, neurotransmitters and membrane phospholipids, all of which are regulators of important physiological processes(Reference Bailey, Stover and McNulty1). SAM is typically referred to as ‘the universal methyl donor’ as it is used for a great number of methylation reactions; its generation in tissues is dependent on an adequate supply of folate and vitamin B12. In summary, effective folate functioning requires essential metabolic interactions with vitamins B12, B6 and riboflavin, and therefore, the sub-optimal status of one or more of these B vitamins, or polymorphisms in folate genes, can impair C1 metabolism, even if folate intakes are adequate.

Fig. 1. (Colour online) Overview of B vitamins in C1 metabolism. DHF, dihydrofolate; DHFR, dihydrofolate reductase; DMNT, DNA methyltransferase; dTMP, deoxythymidine monophosphate; dUMP, deoxyuridine monophosphate; MTHFR, methylenetetrahydrofolate reductase; SAH, S-adenosylhomocysteine; SAM, S-adenosylmethionine; THF, tetrahydrofolate.
Health impacts of folate and related B vitamins through the lifecycle
Folate and the related B vitamins have essential roles in human health. Although the preventative role of folate in neural tube defects (NTD) has been the major focus of public health efforts worldwide, international research is addressing other roles of folate in early, middle and late life and indeed the intergenerational effects of maternal folate status during pregnancy in relation to health outcomes in the offspring during childhood and beyond.
Case study 1: Maternal folate before and during pregnancy with effects on offspring health
Given that folate is essential for cell division and tissue growth, this vitamin plays a particularly important role in pregnancy and fetal development. Over 25 years ago, it was proven beyond doubt that maternal folic acid supplementation in early pregnancy could protect against NTD(2,Reference Czeizel and Dudas3) including spina bifida, anencephaly and related defects. NTD are the most common major malformations of the central nervous system and occur as a result of the failure of the neural tube to close properly in the first few weeks of pregnancy, leading to the death of the fetus or newborn, or to various disabilities involving the spinal cord. The conclusive evidence of the role of folate in preventing NTD has led to folic acid recommendations for women of reproductive age which are in place worldwide(4,5) . As will be discussed later in this review, however, achieving optimal folate status for preventing NTD can be challenging for individuals and populations. Of note, rates of NTD in Ireland are among the highest in the world. A recent report from the Food Safety Authority of Ireland shows that there was a large decrease in the incidence rate of NTD in Ireland from the early 1980s (about 3·5 per 1000 births) to the mid-1990s; however, the rate has remained relatively stable since then at about one per 1000 births (about eighty cases per annum), similar to the UK.
Apart from its well-established role in preventing NTD in very early pregnancy, folate has other essential roles throughout pregnancy, with impacts in early life and beyond. The importance of folate for pregnancy was in fact recognised in historical reports of the discovery of human folate deficiency dating back to the early 1930s, when a fatal anaemia of pregnancy first described in India was proven to be responsive to treatment with food sources of the vitamin(Reference Wills6). Clinical folate deficiency is now known to cause megaloblastic anaemia, a condition characterised by immature, enlarged blood cells (reflecting impaired DNA synthesis), which is reversible with folic acid treatment(Reference Chanarin7). In the absence of maternal folic acid supplementation, folate-related anaemia of pregnancy has been reported to occur in up to 24 % of unsupplemented pregnancies in parts of Asia, Africa and South America and in up to 5 % of those in well-nourished populations(Reference Chanarin7). Erythrocyte and serum folate concentrations typically decrease throughout pregnancy(Reference Hall, Pirani and Campbell8), while folic acid supplementation prevents this decline(Reference McNulty, McNulty and Marshall9) and can thus prevent the occurrence of megaloblastic anaemia of pregnancy(Reference Fletcher, Gurr and Fellingham10,Reference Blot, Papiernik and Kaltwasser11) . As folate is required for the remethylation of homocysteine to methionine, plasma homocysteine is invariably elevated with low folate status(12). Elevated homocysteine, in turn, is associated with increased risk of a number of pregnancy complications including NTD(Reference Mills, Lee and Conley13,Reference Felkner, Suarez and Canfield14) , preeclampsia(Reference Cotter, Molloy and Scott15,Reference Cotter, Molloy and Scott16) , placental abruption(Reference Goddijn-Wessel, Wouters and van de Molen17), recurrent early pregnancy loss(Reference Nelen, Blom and Steegers18), low birth weight and intrauterine growth retardation(Reference Vollset, Refsum and Irgens19).
As reviewed elsewhere(Reference Caffrey, McNulty and Irwin20), in addition to protecting against the development of megaloblastic anaemia in the mother, there is emerging evidence linking maternal folate during pregnancy with offspring neurodevelopment and cognitive function in childhood. Evidence in this area is predominantly based on observational studies reporting significant associations between maternal folate concentrations, or use of folic acid supplements by mothers in pregnancy, and the cognitive performance of children at critical stages of childhood(Reference Julvez, Fortuny and Mendez21–Reference Polańska, Muszyński and Sobala23). However, an intervention study from this centre, the Folic Acid Supplementation in the Second and Third Trimesters trial(Reference McNulty, McNulty and Marshall9), provided a unique opportunity to follow-up the children of trial participants and found direct evidence of beneficial effects of folic acid provided to mothers during pregnancy on the cognitive performance of their children at age 3 and 6 years (i.e. as measured by validated tools, the Bayley Scales of Infant and Toddler Development and Weschler Preschool and Primary Scale of Intelligence, respectively)(Reference Pentieva, McGarel and McNulty24,Reference McGarel, Pentieva and Strain25) . Thus maintaining optimal maternal folate throughout pregnancy, well beyond the early period known to be protective against NTD, may be beneficial for brain development and functioning in the child.
The biological mechanism linking maternal folate with the offspring brain is likely to involve folate-mediated epigenetic changes related to brain development and function. DNA methylation, the most widely studied epigenetic mechanism for gene regulation, is dependent upon the supply of methyl donors provided by folate and related B vitamins via SAM(Reference Bailey, Stover and McNulty1). Folate deficiency could thus lead to aberrant gene expression with consequential health outcomes(Reference James, Sajjadi and Tomar26). As reviewed elsewhere, most epigenetic studies in human subjects have used a candidate gene approach to link maternal status of folate, or reported usage of folic acid supplements, with DNA methylation in offspring genes involved in folate biology and neurodevelopmental processes(Reference Caffrey, McNulty and Irwin20). The only randomised trial to date to investigate the effect of maternal folic acid was carried out at this centre and showed that folic acid supplementation through the second and third trimester of pregnancy resulted in significant changes in DNA methylation in cord blood of LINE-1 and candidate genes related to brain development, IGF2 and BDNF (Reference Caffrey, Irwin and McNulty27). Likewise, animal studies found that folic acid supplementation throughout pregnancy significantly increased brain folate concentrations in the newborn pups, while brain global DNA methylation decreased(Reference Ly, Ishiguro and Kim28). The findings offer a potential biological basis to link maternal folate status with neurodevelopment of the offspring, but this requires further investigation using a genome-wide approach to more thoroughly explore the role of DNA methylation in mediating these effects.
Apart from maternal folate status during pregnancy, the child's folate status also will have important health impacts. Folate biomarkers in children are reported to decline progressively with age in British and American children, possibly indicating that folate requirements of older children are higher to sustain the increased metabolic demands for growth from childhood to adolescence(Reference Kerr, Livingstone and Bates29,Reference Pfeiffer, Hughes and Lacher30) . Such findings have not been taken into consideration in setting current folate recommendations for adolescents, but should perhaps be considered by future panels tasked with evaluating the evidence to support dietary reference values for folate in this population sub-group.
Case study 2: Methylenetetrahydrofolate reductase, riboflavin and blood pressure in the middle-aged adult
Optimal B-vitamin intake may be particularly important for sub-populations with impaired folate metabolism owing to genetic characteristics, most notably the 677C→T variant in the gene encoding the enzyme MTHFR. Since this common folate polymorphism was first described over 20 years ago, it has been linked with several adverse health outcomes, including stroke(Reference McNulty, Strain and Hughes31). Although the health concerns in relation to this polymorphism have predominantly focused on the well-described homocysteine phenotype, arguably of greater relevance to public health is the more recent emergence of a blood pressure phenotype, and a modulating role of riboflavin (MTHFR cofactor), in determining the risk of hypertension(Reference McNulty, Strain and Hughes31).
Hypertension is a significant health concern because it is the leading risk factor contributing to mortality worldwide, accounting for 17 % of all deaths (9·4 million each year), most notably from CVD, with 45 % of deaths from heart disease and 51 % of deaths from stroke estimated to result from hypertension(Reference Lim, Vos and Flaxman32,33) . Reducing blood pressure is however proven to decrease CVD(Reference Mozaffarian, Benjamin and Go34) and it is estimated that lowering of systolic blood pressure by as little as 2 mmHg can decrease cardiovascular risk by as much as 10 %(Reference Lewington, Clarke and Qizilbash35). The risk of hypertension increases with age and occurs as a result of a complex interaction of lifestyle, dietary and environmental factors. Genetic factors are also implicated in the development and progression of hypertension. Of note, a region near the gene encoding the folate-metabolising enzyme MTHFR has been identified by genome-wide association studies among a small number of loci associated with blood pressure in European, American and Asian populations(Reference Newton-Cheh, Johnson and Gateva36,Reference Ehret, Munroe and Rice37) . Epidemiological studies have also linked this folate gene with blood pressure, with the 677C→T polymorphism in MTHFR estimated to increase the risk of hypertension by up to 87 %(Reference Yang, Fan and Zhi38), and CVD by up to 40 %(Reference Holmes, Newcombe and Hubacek39). However, there is large geographical variability in estimates of the excess risk of CVD owing to this polymorphism(Reference McNulty, Strain and Hughes31), suggesting the involvement of a gene–environment interaction. Folate only was previously considered as the relevant environmental factor, but evidence from our centre suggests that riboflavin may be a more important modulating factor via a novel effect on blood pressure(Reference McNulty, Strain and Hughes31).
Riboflavin (vitamin B2) plays an important role in folate recycling within C1 metabolism where it acts in the form of FAD as a cofactor for MTHFR, the enzyme which catalyses the conversion of 5,10 methyleneTHF to 5 methylTHF; the latter cofactor then serves as the methyl donor in the B12-dependent re-methylation of homocysteine to methionine (by methionine synthase). The role of riboflavin within the C1 metabolic network is often overlooked in human health, but it is most evident in individuals with the homozygous mutant 677TT genotype in MTHFR, resulting in a thermolabile enzyme with reduced activity(Reference Frosst, Blom and Milos40). The decreased activity of the variant MTHFR enzyme was demonstrated in molecular studies to be the result of a reduced affinity for its FAD cofactor(Reference Yamada, Chen and Rozen41,Reference Pejchal, Campbell and Guenther42) . In human studies, individuals with the MTHFR 677TT genotype typically present with elevated plasma homocysteine(Reference Frosst, Blom and Milos40), along with low folate concentrations(Reference Molloy, Daly and Mills43). The homocysteine phenotype is known to be most pronounced if the TT genotype occurs in combination with poor nutritional status of either folate(Reference Jacques, Bostom and Williams44) or riboflavin(Reference McNulty, McKinley and Wilson45,Reference Hustad, Midttun and Schneede46) . Furthermore, riboflavin supplementation results in a marked lowering of homocysteine concentrations in individuals with the TT genotype, but not in those with CC or CT genotypes(Reference McNulty, Dowey and Strain47), suggesting that enhancing riboflavin status may stabilise the variant enzyme and thus restore MTHFR activity in vivo.
Recent studies indicate that riboflavin interacts with MTHFR to influence blood pressure and hypertension risk(Reference McNulty, Strain and Hughes31). We have been studying the modulating effect of riboflavin on blood pressure in hypertensive patients (with and without overt CVD) pre-screened for MTHFR genotype and published three randomised trials to date showing that the blood pressure phenotype is highly responsive to lowering by intervention with riboflavin(Reference Horigan, McNulty and Ward48–Reference Wilson, McNulty and Ward50). In the first of these trials, we investigated a cohort of over 400 premature CVD patients with a mean age of 53 years (and 47 years at the time of the event)(Reference Horigan, McNulty and Ward48). At baseline, patients with the variant TT genotype had significantly higher systolic and diastolic blood pressure compared to their age-matched counterparts with CC or CT genotypes, with mean systolic/diastolic blood pressure (mmHg) of 131/80, 133/83 and 143/86 for CC, CT and TT genotypes, respectively. Of greater note, riboflavin supplementation (1·6 mg/d for 16 weeks) resulted in significant blood pressure-lowering in patients with the TT genotype, but no effect in those with CC or CT genotypes(Reference Horigan, McNulty and Ward48). When these high-risk patients were followed up 4 years later, those with TT genotype were again found to be hypertensive, despite marked changes in the number and type of antihypertensive drugs being prescribed (following changes in clinical guidelines for hypertension since the initial investigation), and target blood pressure was again achieved only in response to riboflavin(Reference Wilson, Ward and McNulty49). In a third trial, we demonstrated that the responsiveness of blood pressure to riboflavin intervention in the genetically at-risk group was not confined to high-risk CVD patients but was also achievable in hypertensive adults without overt CVD(Reference Wilson, McNulty and Ward50). At baseline, despite being prescribed multiple classes of antihypertensive drugs, over 60 % of participants with this genotype had failed to reach target blood pressure (≤140/90 mmHg), but riboflavin supplementation for 16 weeks (when antihypertensive drugs remained unchanged) resulted in a significant decrease in blood pressure(Reference Wilson, McNulty and Ward50).
These randomised trials show that riboflavin has a novel and genotype-specific role in lowering blood pressure (by an average of 6–14 mmHg in systolic blood pressure), which occurs independently of antihypertensive medication(Reference Horigan, McNulty and Ward48–Reference Wilson, McNulty and Ward50). Furthermore, antihypertensive therapy as currently prescribed (typically, polytherapy with two or more drugs used in combination) appears to be associated with poorer blood pressure control in patients with the MTHFR 677TT genotype, while the achievement of target blood pressure can be greatly enhanced with supplemental riboflavin at the dietary range of 1·6 mg/d. Given recent calls for more personalised approaches to lower blood pressure and thus improve cardiovascular health(Reference Mozaffarian, Benjamin and Go34), there are clinical and public health implications arising from the finding that the under-recognised blood pressure phenotype associated with this common folate polymorphism is modifiable by riboflavin. For hypertensive patients with the MTHFR 677TT genotype, supplementation with riboflavin could offer a personalised, non-drug treatment to lower blood pressure. For sub-populations worldwide, enhancing riboflavin status could prevent or delay the development of high blood pressure in those with this genetic risk factor. Mechanistic studies are however required to elucidate the precise link between C1 metabolism and blood pressure and to understand the biological perturbation leading to higher blood pressure in the TT genotype and how riboflavin corrects it. Randomised trials investigating the role of this novel gene–nutrient interaction in hypertension through the lifecycle are also required. In any case, at this time while other genetic factors appear to play a role in the development of hypertension(Reference Newton-Cheh, Johnson and Gateva36,Reference Ehret, Munroe and Rice37) , the common 677C→T polymorphism in MTHFR is the only genetic factor linked with hypertension that offers a personalised management option, via optimising riboflavin, the MTHFR cofactor.
Case study 3: Effects of folate and related B vitamins on the brain in the older adult
Dementia and depression are recognised as major disorders of ageing with profound human(51) and economic(Reference Prince, Comas-Herrera and Knapp52–Reference O'Shea and Kennelly54) costs. Dementia affects an estimated 46·8 million people worldwide, with projections that this will increase to over 131 million people by 2050(Reference Prince, Comas-Herrera and Knapp52). Cognitive function typically declines with age, but in some cases, the decline occurs at a greater rate than expected, ranging in severity from relatively minor slips in performing activities, through to subjective cognitive decline, mild cognitive impairment (MCI) and dementia(Reference Calder, Carding and Christopher55). Activities of daily living are profoundly affected in dementia, whereas in MCI, although cognitive decline is greater than expected for an individual's age and education level, there is no notable interference in activities of daily life, albeit 50 % of those with MCI can be predicted to develop dementia within 5 years(Reference Gauthier, Reisberg and Zaudig56). Depression is the most frequent psychiatric disease, and late-life depression is reported more commonly in females, affecting an estimated 28 % of females compared with 22 % of males, over the age of 65 years(Reference Craig and Mindell57). In addition, depression has been shown to increase cognitive dysfunction, while poorer cognitive health can also predispose older adults to depression(Reference Panza, Frisardi and Capurso58,Reference Brailean, Aartsen and Muniz-Terrera59) , suggesting a bi-directional relationship between the two conditions. A recent comprehensive report identified a model of modifiable risk factors for dementia across the lifespan, highlighting the potential for effective prevention through early interventions that target these risk factors(Reference Ly, Ishiguro and Kim28). In this context, as recently reviewed extensively in this journal(Reference Moore, Hughes and Ward60), nutrition can play an important preventative role, with emerging evidence linking certain dietary patterns (particularly the Mediterranean diet) or specific dietary components, including n-3 PUFA, polyphenols, vitamin D and particularly B-vitamins, with a reduced risk of dementia and depression.
Folate, vitamin B12 and vitamin B6 play important roles in the nervous system at all ages, from neural development in early life through to the maintenance of mental health and cognitive function in older age. Thus B-vitamin deficiencies can manifest with significant neurological and neuropsychiatric disturbances. Historically, deficiencies of folate(Reference Carney61,Reference Reynolds, Preece and Bailey62) and vitamin B12(Reference Strachan and Henderson63,Reference Shorvon, Carney and Chanarin64) have been linked with psychiatric illness and poorer mental wellbeing. The reported neuropsychiatric effects of deficiency of either nutrient include cognitive decline, depression and peripheral neuropathy, although the latter is more commonly found in vitamin B12 deficiency(Reference Reynolds65). Folate and vitamin B12 are required for the activity of methionine synthase within C1 metabolism and therefore the synthesis of SAM, which in turn provides methyl groups for numerous central nervous system methylation reactions involving neurotransmitter and membrane phospholipid synthesis and myelin methylation(Reference Selhub, Bagley and Miller66,Reference Bottiglieri, Laundy and Crellin67) . Thus, with folate or vitamin B12 deficiency, the reduction in tissue levels of SAM may contribute to cognitive dysfunction by impairing these methylation processes. Reduced tissue concentration of SAM may be linked to depression through perturbing monoamine (serotonin, dopamine and noradrenaline) synthesis and methylation(Reference Smith and Refsum68). Additionally, vitamin B6 plays an essential role in transamination and decarboxylation reactions, which in turn are involved in neurotransmitter synthesis, metabolism and release; deficiency of vitamin B6 is associated with deficits in nerve conduction(Reference Carney, Ravindran and Rinsler69). The metabolism of homocysteine requires folate along with vitamin B12, and to a lesser extent vitamin B6; when the status of these vitamins is low or deficient, plasma homocysteine concentration will invariably be elevated. Homocysteine concentrations are typically higher in patients with Alzheimer's disease (the most common form of dementia), and are strongly related to the rate of cognitive decline in patients with MCI and Alzheimer's disease(Reference Smith and Refsum68,Reference Porter, Hoey and Hughes70) . Thus, there has been considerable interest in plasma homocysteine as a potential risk factor for cognitive dysfunction, but it remains unconfirmed whether homocysteine is a true disease risk factor or merely a marker. In any case, however, homocysteine measurement provides a sensitive functional biomarker of folate and related B-vitamin status, and plasma homocysteine shows significant lowering in response to B-vitamin intervention(Reference Bailey, Stover and McNulty1).
Lower status of folate, vitamin B12 and /or vitamin B6 (or a higher concentration of the related metabolite homocysteine) is associated in observational studies with cognitive dysfunction(Reference Smith and Refsum68). Observational data supporting a role for these nutrients, or any nutritional factor, can however be complicated by the fact that poor diet may be both a cause and a consequence of impaired cognitive function. Only randomised trials can confirm whether a causative relationship exists. Although several randomised controlled trials have investigated the potential benefits of B-vitamin supplementation on cognitive function, many of these were of insufficient duration to provide clear evidence, while others intervened in patients with confirmed Alzheimer's disease where a beneficial effect is highly unlikely. One well-designed randomised trial of healthy older adults in New Zealand reported no benefit of high-dose combined folic acid/vitamin B12/vitamin B6 for 2 years on any cognitive function parameter examined(Reference McMahon, Green and Skeaff71), whereas another similar study from the Netherlands showed that supplementation with folic acid alone for 3 years significantly improved a number of cognitive parameters including memory, information-processing speed and sensorimotor function(Reference Durga, van Boxtel and Schouten72). An important difference between these two studies was baseline folate status which was far lower in the Dutch trial(Reference Durga, van Boxtel and Schouten72), suggesting that any benefit of folic acid on cognitive function arises through correction of sub-optimal folate status, whereas providing additional folic acid to those with already optimal status may have no effect on cognition. More recent randomised trials, showing that intervention with B vitamins prevented cognitive decline in free-living older adults with depressive symptoms, or improved cognitive performance in participants with MCI, provide convincing evidence that low B-vitamin status may be causatively linked with cognitive dysfunction in ageing. Research in this area has been very substantially underpinned by the work of Smith and co-workers on the VITACOG trial which showed that B-vitamin intervention not only improved cognitive performance(Reference De Jager, Oulhaj and Jacoby73), but also slowed the rate of global and regional brain atrophy as determined using MRI in participants with MCI(Reference Smith, Smith and de Jager74,Reference Douaud, Refsum and de Jager75) . Not all studies support the role of B vitamins, however, and one notable meta-analysis concluded that there was no beneficial effect of either folic acid or vitamin B12 on cognition in older adults(Reference Clarke, Bennett and Parish76). The latter report has however been widely criticised by experts in this area, primarily owing to the inclusion criteria used to select the trials for investigation in the meta-analysis(Reference Garrard and Jacoby77,Reference Smith, De Jager and Refsum78) .
Apart from memory deficits and cognitive dysfunction, depressive symptoms are well described in both folate and vitamin B12 deficiency(Reference Reynolds65). Furthermore, folate deficiency can affect the duration and clinical severity of depression, and is associated with poorer response to antidepressant medication(Reference Reynolds65). Low folate status was associated with a significantly greater risk of depression in one meta-analysis of observational studies(Reference Gilbody, Lightfoot and Sheldon79). Likewise, low concentrations of vitamin B12(Reference Kim, Stewart and Kim80–Reference Robinson, O'Luanaigh and Tehee82) and vitamin B6 (required as a cofactor in the metabolism of tryptophan and serotonin)(Reference Merete, Tucker and Falcon83,Reference Skarupski, Tangney and Li84) have also been linked with depression. Randomised trials, investigating the role of B-vitamin supplements alone or as an adjunct to anti-depressant medications, have however produced conflicting results. The balance of available evidence in this area would appear to suggest that folate and vitamin B12 may have roles in the longer-term management of depression(Reference Taylor, Carney and Goodwin85,Reference Almeida, Ford and Flicker86) .
Overall, there is good evidence to suggest that optimal B-vitamin status has protective effects on cognitive function, and potentially against depressive symptoms, in ageing(Reference Moore, Hughes and Ward60). To address the gaps in the evidence base in this area, however, appropriately designed randomised trials are required, targeting participants with low B-vitamin status and thus most likely to benefit from optimising B-vitamin status to achieve better cognitive and mental health in ageing.
Considerations and challenges in achieving optimal status of B vitamins
Folate
Folic acid refers to the synthetic form of the B vitamin known as folate, whereas the natural folate forms are found in plant, animal and human tissues. Food folates occur naturally in richest supply in green leafy vegetables, asparagus, beans, legumes, liver and yeast. Folic acid is found in the human diet only in fortified foods and supplements but is readily converted to the natural cofactor forms of folate after its ingestion(Reference McNulty, Pentieva and Bailey87). There are however chemical differences between folic acid and the natural folate forms which have important nutrition consequences. Folic acid is a fully oxidised molecule and is a monoglutamate, meaning that it contains just one glutamate moiety in its structure. Naturally occurring food folates on the other hand are a mixture of reduced folate forms (predominantly 5 methyTHF) and typically found as polyglutamates, containing a variable number of glutamate residues(Reference McKillop, McNulty and Scott88). As reduced molecules, natural food folates are inherently unstable outside living cells and tend to have poor bioavailability(Reference McNulty, Pentieva and Bailey87). In addition to their limited bioavailability, food folates (particularly green vegetables) can be unstable during cooking, and this will substantially reduce the folate content of the food before it is even ingested(Reference McKillop, Pentieva and Daly89). Folic acid is more stable and more bioavailable compared to an equivalent amount of the vitamin eaten as naturally occurring food folates(Reference McNulty, Pentieva and Bailey87). As a result of the poor stability and limited bioavailability of folate from natural food sources, achieving optimal folate status is challenging for individuals and populations.
It is important to appreciate that the absence of folate deficiency (i.e. megaloblastic anaemia) does not necessarily mean that folate status is optimal in terms of maintaining health and preventing folate-related disease such as NTD. Thus in many developed countries, folate deficiency may be relatively rare, but sub-optimal folate status is commonly encountered(Reference Bailey, Stover and McNulty1). Folate status and response to intervention are routinely assessed by measuring folate concentrations in serum/plasma or in erythrocytes(Reference Tighe, Ward and McNulty90,Reference Duffy, Hoey and Hughes91) . Serum folate reflects recent dietary intake and is the earliest indicator of altered folate exposure(Reference Gibson92). Erythrocyte folate parallels liver concentrations (accounting for about 50 % of total body folate) and is thus considered to represent tissue folate stores(Reference Wu, Chanarin and Slavin93). Compared with serum folate, erythrocyte folate responds slowly to changes in dietary folate intake and is a better indicator of folate intake over the previous 3–4 months, when circulating folate is incorporated into the maturating red cells(Reference Gibson92,Reference Shane94) . On the basis that homocysteine metabolism requires an adequate supply of folate, the measurement of plasma homocysteine provides a functional biomarker of folate status and is invariably found to be elevated with low or deficient folate status.
Folate intakes and recommendations in the USA and certain other countries are now expressed as dietary folate equivalents, a calculation which was devised to take into account the greater bioavailability of folic acid from fortified foods compared to naturally occurring dietary folates(95,96) . Specifically for the prevention of NTD, women are recommended to take 400 µg/d folic acid as a supplement from preconception until the end of the first trimester of pregnancy(4,5) . Folic acid supplementation is a highly effective means to optimise folate status in women if the recommendations are followed(Reference Cuskelly, McNulty and Scott97,Reference McNulty, Pentieva and Marshall98) . However, it is not an effective public health strategy for populations because in practice very few women take folic acid as recommended before and in early pregnancy and this means that maternal biomarker status of folate is often found to be suboptimal in achieving concentrations known to be protective against NTD(Reference McNulty, Pentieva and Marshall98,Reference Daly, Kirke and Molloy99) . Fortification of food with folic acid, like supplementation, is very effective in optimising dietary intake and biomarker status of folate in those who choose to eat fortified food products(Reference Hoey, McNulty and Askin100,Reference Hopkins, Gibney and Nugent101) . However, fortification has the advantage over supplementation that it can also be highly effective for populations. When folic acid fortification is undertaken on a population-wide basis (via a policy of mandatory fortification), it results in significant increases in folate biomarker concentrations and marked reductions in NTD(Reference Bailey, Stover and McNulty1). Thus reported rates of NTD have declined by between 27 and 50 % in the USA, Canada and Chile in response to the mandatory folic acid fortification of food(Reference Williams, Mai and Mulinare102–Reference Cortés, Mellado and Pardo104). In contrast, in the UK, Ireland and other European countries, policy to prevent NTD (i.e. based on folic acid supplementation) has had little or no impact in preventing NTD, despite active health promotion campaigns over many years(105,106) . This is primarily because the neural tube closes by day 28 post-conception and therefore the timing of folic acid usage by women is critical. For many women, the early period of pregnancy when folic acid is protective against NTD may have passed before folic acid supplements are started. This has resulted in an unacceptably high rate of NTD in European countries, recently estimated to be 1·6 times higher than in regions of the world with mandatory folic acid-fortification policies in place(Reference Khoshnood, Loane and De Walle107). Of particular concern are reports that the incidence of NTD in Ireland is increasing in recent years(Reference McDonnell, Delany and O'Mahony108).
Over eighty countries worldwide to date (including the USA, Canada and Australia) have passed regulations for the mandatory fortification of staple foods with folic acid in order to prevent NTD. Other countries including the UK and Ireland have delayed decisions to introduce mandatory fortification on the basis of concerns relating to possible health risks. Once ingested, folic acid is reduced by dihydrofolate reductase and methylated, then released into the systemic circulation as 5 methylTHF. However, the capacity of dihydrofolate reductase in human subjects to efficiently metabolise folic acid is limited and thus exposure to high oral doses can result in the appearance of unmetabolised folic acid in plasma(Reference Bailey, Stover and McNulty1). On the basis that the latter is not a normal constituent of plasma or other tissues, concerns have arisen regarding potential adverse health effects of unmetabolised folic acid in the circulation arising through high folic acid exposures from supplements and fortified foods. Traditionally this related to the potential risk that long-term exposure to high-dose folic acid might mask the anaemia of vitamin B12 deficiency in older people and allow the associated irreversible neurological symptoms to progress, but this is no longer considered to be a public health issue(Reference Bailey, Stover and McNulty1). A more recent concern has arisen from reports that the presence of unmetabolised folic acid in plasma in elderly people with low vitamin B12 status is associated with worse cognitive performance compared to those with low B12 status and no detectable folic acid in the circulation(Reference Morris, Jacques and Rosenberg109); some subsequent studies have not been able to confirm such findings and therefore this issue remains somewhat controversial(Reference Bailey, Stover and McNulty1). Other evidence suggested that folic acid doses in excess of 1 mg/d could potentially promote the growth of undiagnosed colorectal adenomas in those with pre-existing lesions(Reference Cole, Baron and Sandler110). However, one recent meta-analysis (involving 50 000 individuals) concluded that folic acid supplementation neither increased nor decreased site-specific cancer within the first 5 years of treatment(Reference Vollset, Clarke and Lewington111). One recent study from this centre investigated the effects on circulating unmetabolised folic acid concentrations, in pregnant women and their newborns, of folic acid supplements at a dose of 400 µg/d continued beyond the period of pregnancy currently recommended (i.e. to the end of trimester 1). On the basis that folic acid intervention improved maternal and neonatal folate status(Reference McNulty, McNulty and Marshall9), but did not cause higher concentrations of unmetabolised folic acid(Reference Pentieva, Selhub and Paul112), it was concluded that there was no adverse impact from the exposure of pregnant women to 400 µg/d supplemental folic acid, over and above typical intakes through fortified foods. It remains to be confirmed however whether plasma unmetabolised folic acid arising from higher folic acid intakes is a cause for concern. In the meantime, given the uncertainty regarding the long-term effects of exposure to high-dose folic acid, it is important to avoid population-wide chronic exposures to folic acid at levels higher than are necessary, with evidence that beneficial effects are likely to be achievable at low intakes(Reference Tighe, Ward and McNulty90).
In summary, it is unlikely that there are adverse effects associated with the presence of unmetabolised folic acid in the circulation at the generally low concentrations arising through food fortification. Indeed an expert international panel tasked with reviewing all aspects of folate biology and biomarkers recently concluded that it was ‘not aware of any toxic or abnormal effects of circulating folic acid’ even from much higher exposures than those obtained by food fortification(Reference Bailey, Stover and McNulty1). The risk–benefit debate surrounding food fortification with folic acid is continuing among scientists and policymakers, but the balance of evidence at this time appears to indicate that the proven benefits of folic acid fortification would more than outweigh any potential risks.
Vitamin B12
Low status of B12 is associated with a higher risk of cognitive dysfunction, CVD and osteoporosis(Reference Porter, Hoey and Hughes70,Reference Hughes, Ward and Hoey113) . There are however important public health considerations in relation to vitamin B12. First, the ability to achieve optimal B12 status in practice can be problematic for older people even with high dietary B12 intakes. Secondly, accurately assessing vitamin B12 status, and thus detecting deficient and low status, is difficult.
A severe form of vitamin B12 deficiency arises in pernicious anaemia. This is an autoimmune gastritis characterised by profound B12 malabsorption owing to loss of intrinsic factor, leading to haematological signs (megaloblastic anaemia) and irreversible neurological disease which is fatal if untreated(Reference Stabler114). Once diagnosed, clinical deficiency is readily treatable with regular B12 injections delivered intramuscularly. A much more subtle depletion of vitamin B12 arises as a result of food-bound B12 malabsorption from mild atrophic gastritis which diminishes gastric acid production (i.e. hypochlorhydria). This in turn reduces B12 absorption from food because of the essential role of gastric acid in the release of B12 from proteins in the food matrix(Reference Carmel115). Food-bound B12 malabsorption commonly occurs in older adults, reported to affect up to 20 %(Reference Stabler114), and leads to sub-clinical deficiency, where there is metabolic evidence of deficiency but without the classical haematological or neurological signs(Reference Carmel116). Thus the low B12 status found in older adults is primarily the result of food-bound B12 malabsorption related to atrophic gastritis rather than inadequate dietary intake(Reference Hughes, Ward and Hoey113). Similarly, the use of proton pump inhibitors or other gastric acid suppressant drugs can lower B12 status through malabsorption owing to hypochlorhydria(Reference Hughes, Ward and Hoey113).
Accurate assessment of vitamin B12 status in order to identify and correct low or deficient status is problematic(Reference Hughes and McNulty117). Up to four biomarkers are used to assess B12 status, both direct (total B12 and holotranscobalamin) and functional (homocysteine and methylmalonic acid), but each of these has limitations. Serum total vitamin B12 is the standard test used in clinical settings, with deficiency typically identified as B12 concentrations <148 pmol/l. On the basis that 80 % of total vitamin B12 concentrations is metabolically inert, the measurement of holotranscobalamin has attracted much interest in recent years because it represents the metabolically active fraction of B12 available for cellular processes. Holotranscobalamin shows promise as a reliable biomarker of vitamin B12 status, but the influence of confounding factors needs to be more fully explored(Reference Hughes and McNulty117). Measurement of metabolites of vitamin B12-dependent reactions can also provide useful functional indicators of B12 status. With B12 depletion, the activity of B12-dependent enzyme methionine synthase will be impaired leading to an elevation of total homocysteine that can be readily measured in plasma. Plasma homocysteine is however not specific to vitamin B12 as it is influenced by folate, other B vitamins and non-nutrient factors including renal function, limiting its use as a biomarker of B12 status(Reference Hughes and McNulty117). Vitamin B12 depletion also leads to reduced activity of methylmalonyl CoA mutase and an accumulation of the by-product methylmalonic acid which can be measured in plasma. Measurement of methylmalonic acid, unlike homocysteine, provides a specific biomarker for vitamin B12. Serum methylmalonic acid is invariably elevated in patients with B12 deficiency and it also provides a useful biomarker of B12 status in population-based studies(Reference Valente, Scott and Ueland118).
In summary, accurate assessment of vitamin B12 is problematic and there is no consensus as to the best biomarker to use in clinical or research settings. It is now recommended that more than one biomarker be used to accurately diagnose B12 deficiency, and recently approaches that identify deficient status using combinations of two or more biomarkers have emerged(Reference Hughes and McNulty117). Ensuring that vitamin B12 deficiency is diagnosed and treated in patients, and B12 status optimised in older populations generally, should be prioritised in order to ensure that any adverse health consequences of deficient and low B12 status are prevented.
Riboflavin and its metabolic interaction with vitamin B6
Riboflavin, in its cofactor forms FMN and FAD, is essential for numerous oxidation–reduction reactions and plays a fundamental role in the metabolism of energy, certain drugs and toxins and in supporting cellular antioxidant potential(Reference McCormick and Shills119,Reference Powers120) . Riboflavin-dependent metabolism involves interaction with a number of other nutrients. Riboflavin deficiency in animals is associated with impaired iron absorption, whilst riboflavin supplementation in human subjects was shown to enhance circulating Hb concentrations and improve the response of iron deficiency anaemia to iron therapy(Reference Powers, Hill and Mushtaq121). In addition, riboflavin involves close metabolic interaction with vitamin B6, in that it is required (as FMN) for the generation of pyridoxal 5′ phosphate (PLP; the active vitamin B6 coenzyme form) in tissues from pyridoxine phosphate by pyridoxine-phosphate oxidase. Animal studies show that pyridoxine-phosphate oxidase activity is sensitive to changes in dietary riboflavin intake, with evidence that riboflavin deficiency can alter PLP levels(Reference Rasmussen, Barsa and McCormick122). In human subjects, research from our centre demonstrated the metabolic dependency of vitamin B6 on riboflavin by showing that supplementing older adults with riboflavin not only improved biomarker status of riboflavin, but also led to a significant increase in blood PLP concentrations(Reference Madigan, Tracey and McNulty123).
As described earlier in this review, emerging evidence points to a novel role of riboflavin as an important modulator of blood pressure specifically in genetically at-risk individuals owing to the 677C→T polymorphism in MTHFR (Reference McNulty, Strain and Hughes31). The precise biological mechanism explaining this novel gene–nutrient interaction in blood pressure is unclear at this time, however, MTHFR activity in people with the TT genotype appears to be particularly sensitive to riboflavin status(Reference McNulty, McKinley and Wilson45). One could speculate that people with the variant TT genotype who have optimal riboflavin status may have a higher capacity to replace inactivated enzyme than TT genotype individuals with low riboflavin status. Alternatively, a higher riboflavin status may prevent the FAD cofactor from leaving the active site or may allow its quick replacement, thus stabilising the variant form of the enzyme. Overall the evidence indicates that these genetically at-risk adults have higher riboflavin requirements in order to sustain normal MTHFR activity although this remains to be specifically demonstrated(Reference McNulty, McKinley and Wilson45,Reference McNulty, Dowey and Strain47) .
On the limited evidence available, riboflavin deficiency is a significant problem in developing countries(Reference Whitfield, Karakochuk and Liu124). Across the developed world also, sub-optimal riboflavin status may be widespread, but this is largely undocumented as biomarker status is rarely measured in population-based studies(Reference McAuley, McNulty and Hughes125). The UK is in fact one of the very few countries worldwide to have included a riboflavin biomarker as part of its rolling National Diet and Nutrition Survey survey(126). Much more recently Ireland, in its National Adult Nutrition Survey, has measured biomarker status of riboflavin for the first time on a population-wide basis and the results are in close agreement with those of National Diet and Nutrition Surveys(126,Reference Kehoe, Walton and Hopkins127) .
In the British and Irish population-wide nutrition surveys, biomarker status of riboflavin was measured using erythrocyte glutathione activation coefficient, widely accepted as the gold-standard measure of status(Reference Hoey, McNulty and Strain128). This coefficient is expressed as the ratio of the activity of the enzyme glutathione reductase in lysed red cells with and without addition of the cofactor FAD. Erythrocyte glutathione activation coefficient, therefore, is a measure of glutathione reductase enzyme saturation with its riboflavin-derived cofactor; a low coefficient is generally considered to be normal, while higher values are indicative of suboptimal riboflavin status, although there is no universal agreement as to the cut-off points indicative of deficient and low status. Advantages of the erythrocyte glutathione activation coefficient assay include stability and high sensitivity to small degrees of cofactor desaturation, while the lack of accessibility of this assay worldwide and very specific pre-analysis processing (including the need for washed erythrocytes) makes this assay unfeasible in many settings(Reference Hoey, McNulty and Strain128). Concern has been expressed regarding the large proportion of adults, as assessed in both the British and Irish population-based surveys, showing low biomarker status of riboflavin using erythrocyte glutathione activation coefficient. The functional significance of such findings is unclear however since in general, with the exception of younger women, mean dietary riboflavin intakes of British and Irish adults compared favourably with dietary reference ranges(126,Reference Kehoe, Walton and Hopkins127) . Elsewhere in the world (including Canada and USA), the situation is much less clear as riboflavin biomarkers are not measured (by any method) in nutrition surveys.
In summary, on the limited available evidence, sub-optimal riboflavin status appears to be a more widespread problem than is generally recognised across the world because of the current reliance on dietary data only in nutrition surveys, without corresponding information on riboflavin biomarker status. There is a need to measure riboflavin biomarkers in population surveys, and to demonstrate the functional, gene–nutrient and health effects of riboflavin across the range of values, from deficient to optimal biomarker status.
Conclusions
The health impacts, challenges and research priorities in relation to folate and related B vitamin status at key stages of lifecycle were reviewed using case studies to illustrate the roles of these vitamins in early, middle and late life (Table 1). To summarise:
Table 1. Health impacts and challenges in relation to folate and related B vitamins at key stages of the lifecycle
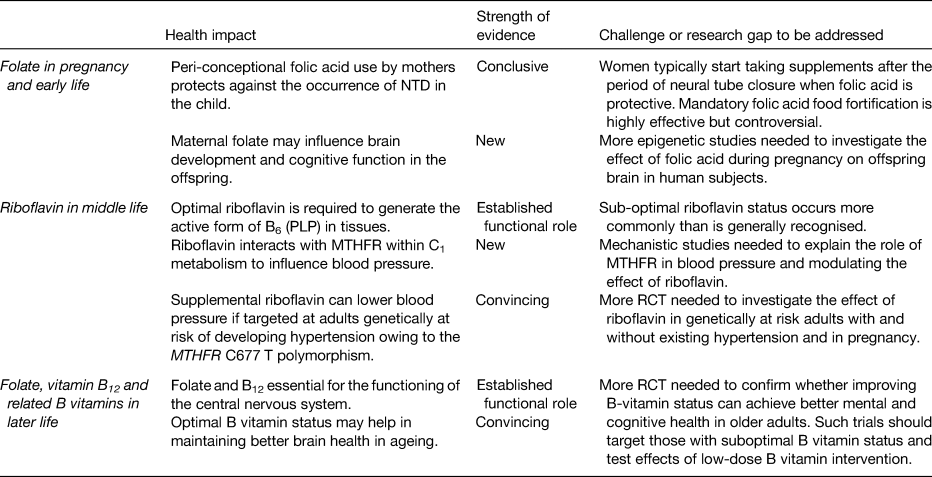
NTD, neural tube defects; MTHFR, methylenetetrahydrofolate reductase; PLP, pyridoxal 5′ phosphate; RCT, randomised cotrolled trials.
Optimal maternal folate status prevents megaloblastic anaemia in mothers during pregnancy and protects against the occurrence of NTD in the child. Folic acid, the vitamin form found in fortified foods and supplements, provides a highly bioavailable source of folate. Folic acid supplementation as a policy to prevent NTD has however proven to be largely ineffective in the UK, Ireland and elsewhere in Europe primarily because women typically start taking folic acid after the period of neural tube closure (3rd to 4th week of pregnancy) when folic acid is protective. Apart from preventing NTD, the human in utero environment may influence the offspring brain health in the longer term via DNA methylation, which is dependent on an adequate supply of folate, but this aspect requires further investigation.
Optimal riboflavin status is required for the generation of the active form of B6 (PLP) in tissues, with some evidence that riboflavin may be the more limiting nutrient in human subjects with low PLP concentrations. Suboptimal riboflavin may be a widespread problem in all populations worldwide, but this is not well recognised because riboflavin status biomarkers are rarely measured. A novel gene–nutrient interaction, involving riboflavin and MTHFR, has emerged in recent years, with important effects on blood pressure. Emerging evidence shows that targeted riboflavin supplementation could offer a personalised, non-drug treatment to lower blood pressure and improve blood pressure control in hypertensive patients with the variant MTHFR 677TT genotype. In a wider public health context, sub-populations worldwide with this genotype may benefit from optimising riboflavin status to prevent or delay the development of high blood pressure in middle age. This in turn could potentially reduce the risk of stroke; however, large clinical trials are required to investigate the effects on stroke and other disease end-points.
Folate and vitamin B12 are particularly important in later life because they are essential for the brain to support numerous central nervous system methylation reactions involving neurotransmitter and membrane phospholipid synthesis and myelin methylation. Subclinical deficiencies of these and related B vitamins are implicated in cognitive decline and depression in older adults, while optimal B vitamin status may help in maintaining better brain health in ageing. Well-designed randomised trials are required to confirm whether improving B-vitamin status can achieve better mental and cognitive health in older adults. Any intervention with B vitamins is likely to be most effective before overt signs of neuropsychiatric disease has occurred and in people with low B-vitamin status. Given some concerns regarding the potentially harmful effects of folic acid at high exposure levels in older people with low vitamin B12 status, future trials should investigate the effects of intervention at low-dose intakes.
In conclusion, there are important health impacts of folate and metabolically related B vitamins through the lifecycle. There are also significant public health challenges to be overcome in order to achieve optimal status, and thus potentially prevent folate-related disease at a population level. For governments worldwide considering the relevant policy issues, there is a need for a balanced approach, and an emphasis on maintaining an optimal status of all relevant B vitamins throughout life.
Financial Support
The research described in this review was supported in part by: governmental funding from the Irish Department of Agriculture, Food and the Marine and Health Research Board (under the Food Institutional Research Measure initiative) and from the Northern Ireland Department for Employment and Learning (under its Strengthening the All-Island research base initiative); and from DSM Nutritional Products (post-doctoral research post for C. F. H. received). None of these entities were involved in the writing of the present paper.
Conflicts of Interest
None.
Authorship
H. McN. drafted the manuscript; M. W., L. H., C. F. H. and K. P. critically revised the manuscript for important intellectual content. All the authors have read and approved the final manuscript.