Seasonal cycles of energy metabolism
Variation across the seasons in food consumption and in energy expenditure related to locomotor activity and thermogenesis is extremely common in mammals that have evolved in non-equatorial latitudes. This results in annual cycles in body composition and body weight, that integrate with other seasonal cycles, for example in reproductive activity, hibernation and coat growth. These cyclical changes in behaviour and in energy metabolism result from a combination of innate circannual rhythmicity and direct responses to the annual change in photoperiod, and thereby ensure that physiological adaptations occur in anticipation of altered energetic demands and changing food supply. The sensory pathways that detect annual changes in photoperiod and convey this information to the neuroendocrine axes that control seasonal cyclicity are well characterised in mammals, being dependent upon changes in the nocturnal secretion of melatonin from the pineal gland(Reference Ebling1). However, the mechanisms that subsequently control changes in appetite and energy expenditure appear to be separate from well-characterised hypothalamic mechanisms, such as the neuropeptide Y and pro-opiomelanocortin-derived peptide pathways that are known to be crucial in the short-term regulation of food intake and in the compensatory responses to acute energy restriction(Reference Ebling and Barrett2).
The Siberian hamster has emerged as an excellent model species for investigating the mechanisms underlying seasonal neuroendocrine cycles. It can be maintained in conventional laboratory environments, and simply by changing the ambient lighting conditions from a ‘summer’ long day of 16 h light:8 h dark to a ‘winter’ short day of 8 h light:16 h dark, a complete range of winter adaptations can be initiated (Fig. 1(Reference Reddy, Cronin and Ford3, Reference Ebling, Arthurs and Turney4)). These include a 25 % reduction in voluntary food intake (Fig. 1), about 30 % loss of body weight reflecting catabolism of intra-abdominal white fat, plus inactivation of the reproductive axis resulting in azoopermia in males and an anoestrus state in females, moulting of the coat to a winter pelage (Fig. 1), and eventually torpor. Despite these profound behavioural and physiological changes, analysis of hypothalamic gene expression including neuropeptide Y, pro-opiomelanocortin-derived peptide and orexin failed to detect major seasonal changes in expression(Reference Reddy, Cronin and Ford3, Reference Mercer, Lawrence and Moar5, Reference Rousseau, Atcha and Cagampang6). The most profound changes in gene expression occurred not in neuronal areas but in ependymoglial cells that occupy the floor and ventrolateral walls of the third ventricle(Reference Barrett, Ivanova and Graham7). These cells are akin to radial glia found much earlier in development, that were classified by Horstmann in 1954 as tanycytes (reviewed in(Reference Goodman and Hajihosseini8)). Many lines of evidence now suggest that tanycytes act as the principal mediators of the seasonal programming of metabolic and reproductive functions to allow adaptive physiological responses to environmental challenges(Reference Lewis and Ebling9).
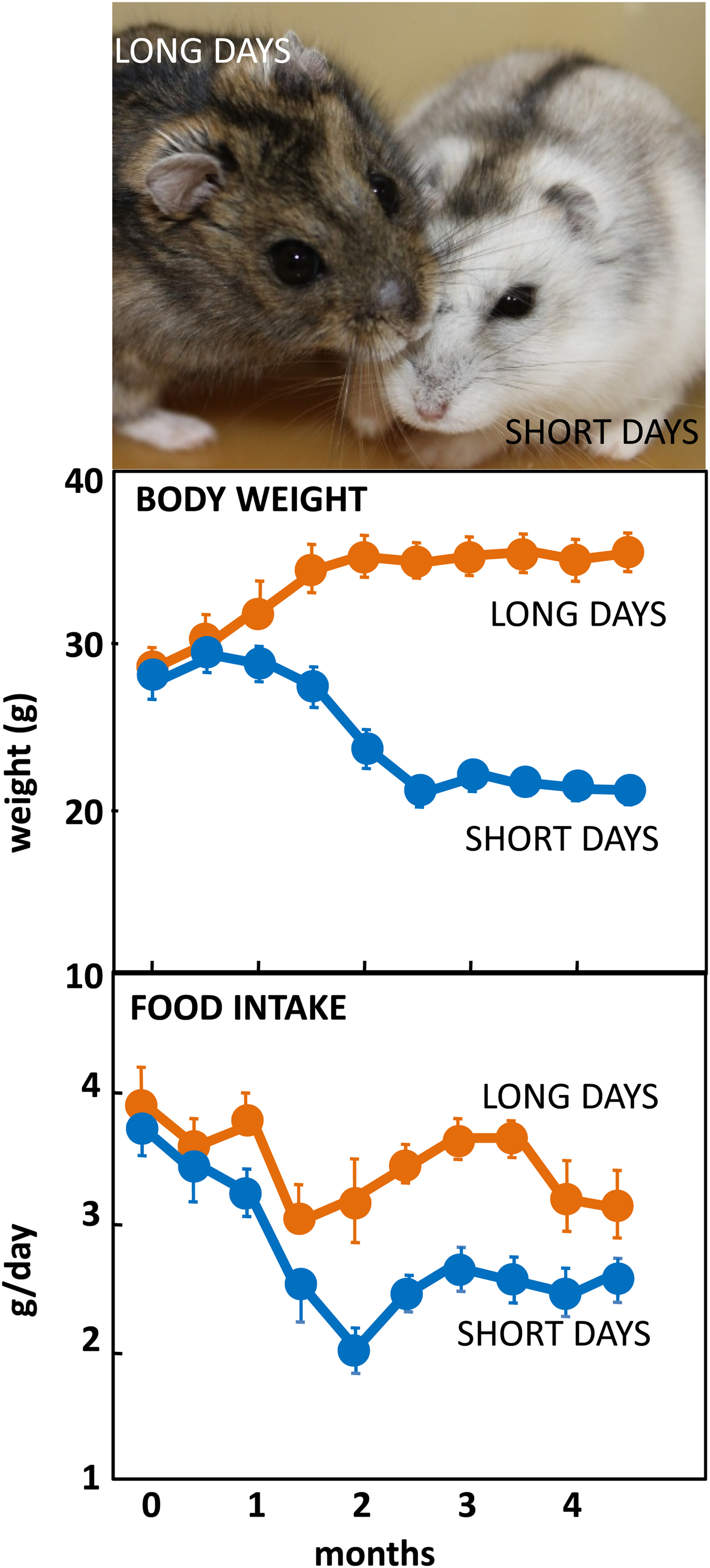
Fig. 1. (Colour online) Top: Adult male Siberian hamsters in the agouti summer pelage after exposure to long days, or having moulted to a white pelage after prolonged exposure to short days. Photograph© Dr Perry Barrett. Middle: Body weight in adult male hamsters maintained in long days or short days, and bottom: daily food intake in the same cohort of hamsters. Data redrawn from(Reference Ebling, Arthurs and Turney4).
Tanycytes as critical mediators of adaptive seasonal cyclicity
Evidence for a role of tanycytes in controlling seasonal neuroendocrine function was first obtained in studies of the control of reproduction by gonadotrophin-releasing hormone (GnRH) neurons. Electron microscopic analysis revealed structural rearrangements of GnRH neuron terminals between the breeding and anoestrus seasons in adult ewes(Reference Xiong, Karsch and Lehman10). GnRH nerve endings are embedded by tanycytic end-feet, raising the possibility that a plastic relationship between tanycytes and GnRH neuron terminals may exist that underlies seasonal changes in pituitary gonadotrophin (luteinising hormone, follicle stimulating hormone) release(Reference Prevot, Bellefontaine and Baroncini11).
Akin to the GnRH gating scenario, tanycytes appear to regulate the bioavailability of thyroid hormones (Fig. 2). Within the median eminence at the base of the hypothalamus, the axonal terminals of thyrotropin-releasing hormone-producing neurons converge with the processes of tanycytes which thus regulate their access to the portal blood(Reference Goodman and Hajihosseini8). This elegant machinery is believed to be modulated by photoperiod-dependent changes in the secretion of melatonin by the pineal gland. It is clear that a major site of action of the photoperiod-encoding hormone melatonin is the pituitary stalk surrounding the median eminence, the pars tuberalis, as this tissue expresses a dense abundance of melatonin receptors across all seasonal mammalian species(Reference Morgan and Hazlerigg12). As daylength changes through the seasons, the nocturnal secretion of melatonin changes, which, in turn, regulates the expression of thyrotropin-stimulating hormoneβ gene encoding thyrotropin-stimulating hormone in the pars tuberalis (Reference Hanon, Lincoln and Fustin13). Studies on sheep, photoperiodic Fischer 344 rats and Siberian hamsters have demonstrated that tanycytes express the thyrotropin - stimulating hormone receptor, and that binding of thyrotropin stimulating hormoneβ to this receptor on tanycytes induces the expression of deiodinase 2 gene expression (Dio2). This gene encodes the brain-specific form of the enzyme which converts the inactive form of thyroid hormone (T4: thyroxine) into the biologically active form (T3: tri-iodothyronine). In long photoperiods, the short duration of nocturnal melatonin secretion at night results in increased thyrotropin - stimulating hormoneβ production in the pars tuberalis, resulting in increased thyroid hormone bioavailability in the surrounding hypothalamus(Reference Nishiwaki-Ohkawa and Yoshimura14). Conversely, short-day photoperiod exposure reduces local availability of T3 by upregulating deiodinase 3 (Dio3) mRNA in tanycytes, encoding the brain-specific form of the enzyme that converts T4 and T3 to inactive precursors(Reference Barrett, Ebling and Schuhler15).
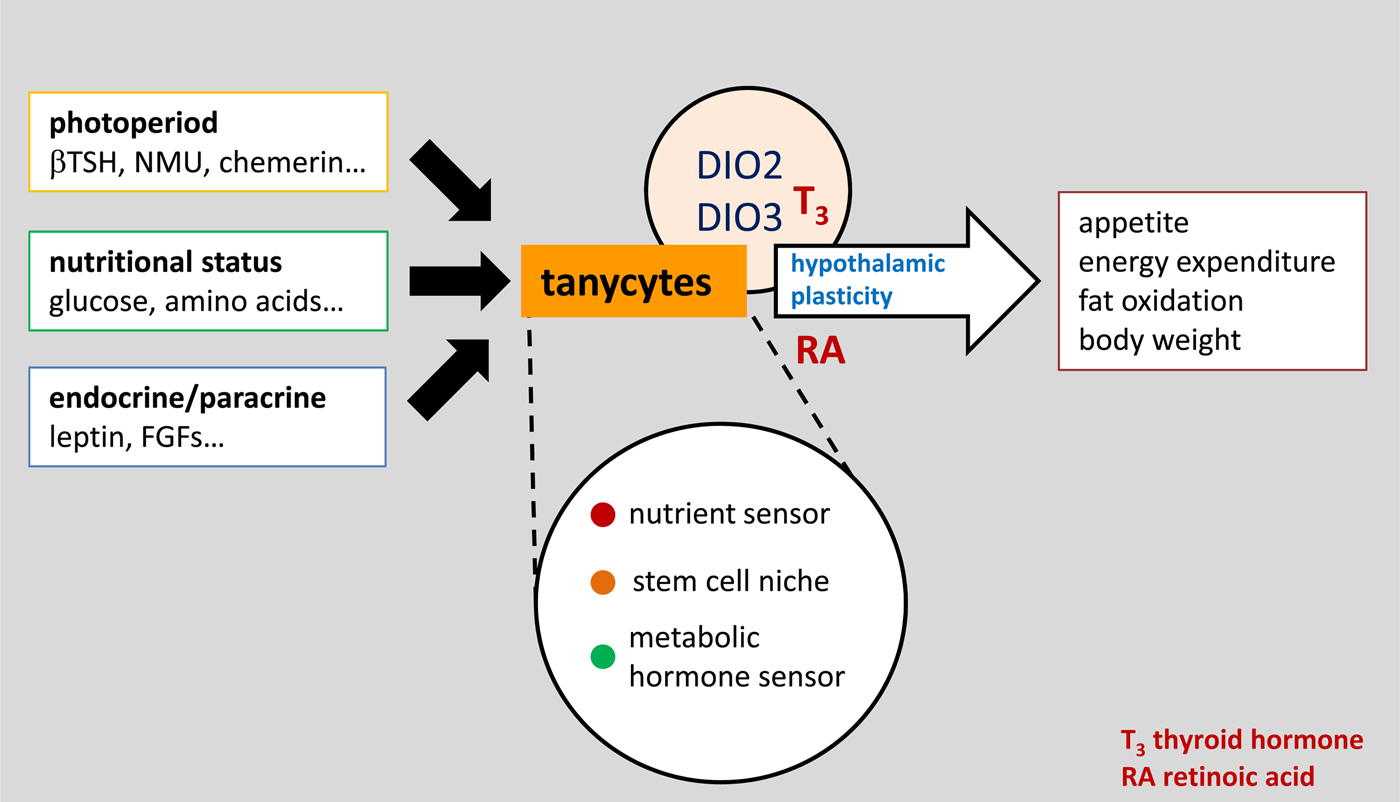
Fig. 2. (Colour online) A conceptual view of tanycytes as a key integrator of external and internal signals that influence the structure and function of the surrounding hypothalamus, and therefore regulate physiology and behaviour via pathways that are initially important in the development of the brain, including both thyroid hormone and retinoic acid. βTSH, β thyroid stimulating hormone; NMU, neuromedin U; FGF, fibroblast growth factor.
Seasonal changes in Dio2 and/or Dio3 gene expression in tanycytes have been observed across a wide range of species, but it is experimental studies in the Siberian hamster that provide the most convincing evidence that changes in local hypothalamic thyroid hormone (T3) availability are sufficient to cause changes in appetite and energy expenditure(Reference Barrett, Ebling and Schuhler15, Reference Murphy, Jethwa and Warner16). This has been achieved by using stereotaxic surgery to place microimplants releasing T3 directly into the mediobasal hypothalamus in order to maintain the high local concentrations that would be characteristic of the long day state when Dio2 expression is high and Dio3 expression is low. Such implants block the winter hypophagia and body weight loss induced by transferring hamsters from long to short days(Reference Barrett, Ebling and Schuhler15), and conversely, when placed into hamsters already in the short-day (winter) state they increase food intake and induce weight gain(Reference Murphy, Jethwa and Warner16). Although it may seem paradoxical that high levels of thyroid hormone promote an anabolic state, it should be remembered that this action is entirely local to the hypothalamus as the systemic thyroid axis is not affected by these intrahypothalamic implants. Indeed, if there were to be any feedback consequences, then one might expect high hypothalamic levels to suppress central activation of the thyroid axis, though this was not observed in the hamster studies.
Subsequent detailed analysis of annual patterns of Dio2 and Dio3 expression suggest that changes in thyroid hormone signalling are unlikely to be the only driver of seasonal changes in appetite and metabolism. It is likely that tanycytes respond to a number of other signals from the pars tuberalis that change seasonally, including chemerin(Reference Helfer, Ross and Thomson17) and neuromedin U(Reference Helfer, Ross and Morgan18), and given the large number of other genes that change seasonally in tanycytes there are surely other output signals, perhaps the best characterised being retinoic acid signalling pathways(Reference Shearer, Goodman and Ross19). Regardless of the input and output signals, tanycytes seem to be a key node integrating photoperiodic information derived from the pars tuberalis and communicating with neuroendocrine mechanisms. This conclusion is supported by evidence that tanycytes receive many other types of metabolic, hormonal and paracrine input signals that ultimately influence neuroendocrine function, as discussed later (Fig. 2).
Tanycytes regulate transport of metabolites into the hypothalamus
The identification of anorexigenic and orexigenic neurons in the hypothalamic arcuate nucleus and subsequent recognition of their role in the regulation of feeding has prompted considerable debate regarding how circulating metabolites reach this structure(Reference Langlet20). Due to their strategic proximity to fenestrated capillaries, early studies suggested that tanycytes might act as a conduit for peripheral metabolites into the brain. While the idea that tanycytes could somewhat contribute to the regulation of energy homeostasis has been purely speculative for a number of years, emerging evidence suggests that these cells are critical components of the hypothalamic network controlling energy balance (Fig. 2). For instance, in a series of seminal observations, Rodriguez et al.(Reference Rodriguez, Blázquez and Pastor21) demonstrated that when the tracer horse radish peroxidase is injected into the cerebrospinal fluid, it rapidly diffuses to the hypothalamic parenchyma but not to the median eminence. This finding led to the suggestion that median eminence tanycytes, known as β2 tanycytes, could have direct access to circulating plasma, allowing passive and active transport of blood-borne molecules. This hypothesis has been supported by a recent study in which peripheral administration of the anorexigenic hormone leptin was shown to activate its receptor on median eminence tanycytes before being internalised and released into the cerebrospinal fluid(Reference Balland, Dam and Langlet22). Western blot analysis confirmed that once inside the cerebrospinal fluid, leptin diffuses to the hypothalamic parenchyma through the walls of the third ventricle to bind to arcuate nucleus neurons and regulate food intake. However, further studies revealed that tanycytes might not be the only cell type to regulate leptin uptake in the mediobasal hypothalamus as Djogo et al.(Reference Djogo, Robins and Schneider23) demonstrated that the maintenance of leptin receptor positive dendrites requires median eminence oligodendrocyte precursor cells. In addition, the hypothesis that median eminence fenestrated capillaries could represent a direct vascular input for passive diffusion of signals into the arcuate nucleus has also been proposed(Reference Ciofi24).
In this context, while Langlet et al.(Reference Langlet, Levin and Luquet25) have shown that blood-borne molecules are unable to directly reach the arcuate nucleus in the absence of active transport through tanycytes, the same study also demonstrated that tanycytes can undergo morphological changes according to oscillating physiological states to modulate vascular permeability. Indeed, fasting-induced hypoglycaemia has been shown to promote an increase in vascular endothelial growth factor expression in tanycytes, resulting in greater contact between metabolic signals and arcuate nucleus neurons(Reference Langlet, Levin and Luquet25). Thus, it seems plausible that during an energy imbalance, the active but indirect transport of metabolites into the arcuate nucleus is replaced by passive but direct access through an increase in the fenestration of the capillaries(Reference Langlet20). Moreover, simultaneous to vascular plasticity, tanycytes have been found to reorganise the tight junctions at their apical pole to confine blood-borne molecules to the arcuate nucleus during starvation(Reference Langlet20). This mechanism is thought to maintain brain homeostasis by preventing the diffusion of blood-borne molecules beyond the median eminence under fasting conditions. Notably, changes in tanycyte barrier plasticity have also been described for the orexigenic hormone ghrelin, whereby feeding status was found to dynamically regulate ghrelin entry into the arcuate nucleus parenchyma in a nutrient-dependent manner(Reference Schaeffer, Langlet and Lafont26). Taken together, these observations point to the possibility that tanycytes detect and rapidly respond to changes in the concentrations of specific nutrients, although the precise mechanisms of nutrient transport and sensing in the hypothalamus are yet to be fully elucidated.
Nutrient sensing properties of tanycytes
The location of tanycytes at the interface of the hypothalamic parenchyma and the cerebrospinal fluid and their proximity to key hypothalamic nuclei involved in the control of energy balance are suggestive of a role in nutrient sensing (Fig. 2). As mentioned earlier, Langlet et al.(Reference Langlet, Levin and Luquet25) convincingly showed that variations in glucose levels induce morphological changes in tanycytes in fasting mice. This finding reconciles with previous studies in which α1, α2 and β1 tanycytes were found to respond to elicit waves of calcium mobilisation in response to changing concentrations of glucose in acutely prepared brain slices(Reference Frayling, Britton and Dale27). Moreover, Frayling et al.(Reference Frayling, Britton and Dale27) demonstrated that tanycytes exhibit large ATP-dependent Ca2+ waves following focal application of exogenous glucose, leading to extracellular diffusion of ATP, activation of P2Y1 receptors and further propagation of the Ca2+ wave through adjacent tanycytes. Interestingly, further evidence for the glucosensing properties of tanycytes was provided by studies in primary cultures of hypothalamic cells that were enriched in tanycytes. Indeed, Orellana et al.(Reference Orellana, Saez and Cortes-Campos28) also showed that glucose responses in cultured β-tanycytes were accompanied by secretion of ATP, activation of P2Y1 receptors and widespread increase in intracellular Ca2+. While these findings provide direct evidence for the glucosensitivity of tanycytes, the understanding of the molecular mechanisms by which these cells detect glucose remains elusive.
Based on the observation that tanycytes express Glut2, parallels have been drawn between the glucosensing properties of pancreatic β cells and tanycytes(Reference García, Millán and Balmaceda-Aguilera29). Indeed, one proposed model for the glucosensitivity of tanycytes predicts that glucose is transmitted into cells through GLUT-2 and converted to glucose-6-phosphate by glucokinase. This molecule is then thought to enter the Krebs cycle to generate an increase in intracellular ATP. This, in turn, causes K-ATP channels to close, leading to an increase in intracellular Ca2+. While this model has been highly influential, the rapidity of the glucose-evoked response in tanycytes calls into question the pancreatic β cell paradigm. In addition, tanycytes have also been shown to respond with Ca2+ waves to non-metabolisable analogues of glucose, such as 2-deoxyglucose, and methyl glucopyranoside(Reference Frayling, Britton and Dale27). Thus, it appears likely that additional glucosensing mechanisms may be involved. In this context, a recent study from Benford et al.(Reference Benford, Bolborea and Pollatzek30) showed that depletion of the sweet taste (Tas1r2/Tas1r3) receptor in the mouse hypothalamus increases the proportion of glucose-insensitive tanycytes in acute brain preparations. Importantly, this study crucially showed that the majority of tanycytes in rodents sense glucose via the sweet taste receptor, although a large proportion of them exhibited alternative glucosensing mechanisms. Finally, it cannot be excluded that tanycytes are not directly implicated in the central detection of glucose and that other cell types, such as astrocytes, sense changes in plasma glucose levels and transmit this information to tanycytes(Reference Yi, Habegger and Chowen31).
Similar to glucosensing, new evidence suggests that hypothalamic tanycytes can also detect amino acids. Using Ca2+ imaging, Lazutkaite et al.(Reference Lazutkaite, Soldà and Lossow32) have recently showed that two receptors previously found in taste cells, namely the Tas1r1/Tas1r3 heterodimer and mGluR4, mediate amino acid detection mechanisms in rodent tanycytes via a process similar to taste cells in the tongue. Moreover, microelectrode ATP biosensing in acute brain slices of rats indicated that tanycytes respond to a range of essential and non-essential amino acids through an increase in intracellular Ca2+ followed by the release of ATP. In turn, extracellular diffusion of ATP results in the activation of P2X and P2Y receptors which then produces a wave of ATP that is suggested to propagate to the hypothalamic parenchyma. Although this finding warrants further investigation, the mechanistic insights provided by Lazutkaite et al.(Reference Lazutkaite, Soldà and Lossow32) offer potential for direct tanycyte-to-neuron signalling. Importantly, previous studies showed that both high-fat and low-fat diet reduce neurogenesis in the arcuate nucleus while increasing cell proliferation in the median eminence(Reference Lee, Yoo and Pak33), pointing to the possibility that tanycyte amino acid sensing informs the hypothalamus about changes in nutritional states to maintain normal levels of neurogenesis through direct tanycyte-to-neuron signalling. Consistent with this hypothesis, diet-induced obese mice have been found to express increased levels of ciliary neurotrophic factor mRNA in tanycytes, suggesting that dietary signals might alter hypothalamic neurogenesis through increased ciliary neurotrophic factor signalling(Reference Severi, Perugini and Mondini34).
Hypothalamic tanycytes as stem cells
Although a large body of evidence supports the notion that new cells can be generated in the adult hypothalamus, much debate exists regarding the location and identity of the stem cells. Due to their ability to incorporate bromodeoxyuridine (BrdU), early studies indicated that tanycytes might represent progenitor cells in the postnatal hypothalamus. Indeed, Kokoeva et al.(Reference Kokoeva, Yin and Flier35) demonstrated that direct intracerebroventricular infusion of BrdU in rodents results in the emergence of BrdU-labelled cells in the hypothalamic parenchyma. Moreover, later studies showed that a subset of these newly generated cells differentiated not only into neurons but also into astroglial cells, reinforcing the notion that these specialised glial cells are an important neurogenic niche(Reference Kokoeva, Yin and Flier36). However, while BrdU labelling proved instrumental in elucidating the neurogenic properties of tanycytes, these studies failed to identify the exact subtype and location of these stem cells. Nonetheless, early morphological observations offered tentative evidence for α-tanycytes as potential neural stem cells(Reference Rodriguez, Blázquez and Pastor21). This finding was later confirmed by Robins et al.(Reference Robins, Stewart and McNay37). Using a glutamate aspartate transporter (GLAST)CreERT2 conditional driver, this group demonstrated that GLAST-expressing α-tanycytes constitutively give rise to β1-tanycytes, although long-time point analysis revealed that the major parenchymal contributions were to the astroglial lineage. In contrast, β-tanycytes have been suggested to give rise to a predominantly neuronal progeny which integrates into the arcuate and ventromedial nuclei(Reference Haan, Goodman and Najdi-Samiei38). Furthermore, microdissection analysis of the third ventricle revealed that GLAST+ve α-tanycytes exhibit neurospherogenic capacity whereas GLAST−ve β-tanycytes do not form neurospheres(Reference Robins, Stewart and McNay37). While these findings suggest that subpopulations of tanycytes contribute differently to hypothalamic cell-turnover, the observation that unidentified cells within the parenchymal region of GLAST::CreERT2 mice express the GLAST reporter confound the interpretation of the absolute parenchymal contribution of α-tanycytes(Reference Rizzoti and Lovell-Badge39).
Regardless of the ventricular or parenchymal origin of hypothalamic neural stem cells, cell lineage tracing studies have now firmly established the existence of active neurogenesis among hypothalamic tanycytes. However, new evidence suggests that in addition to dietary intake, photoperiodic stimuli can also modulate tanycyte cell division in the adult hypothalamus. Indeed, Migaud et al.(Reference Migaud, Batailler and Pillon40) have shown that the transition from long to short days increases the proliferative capacity of tanycytes in the adult sheep hypothalamus as measured by expression of the neural stem cell marker vimentin. Importantly, this finding is in line with previous observations in Syrian hamsters, where short-day photoperiod exposure was found to significantly increase BrdU labelling in the hypothalamus(Reference Huang, De Vries and Bittman41). In contrast, Barrett et al.(Reference Barrett, Ivanova and Graham7) demonstrated that short days down-regulate an intermediate filament protein known as nestin in hypothalamic tanycytes of Siberian hamsters, suggesting decreased proliferative activity during this photoperiod. In addition, no clear seasonal variations have been observed in the proliferative capacity of tanycytes in Soay rams(Reference Hazlerigg, Wyse and Dardente42). While discrepancies in the immunohistochemical procedure or photoperiod treatment may account for conflicting results in the earlier studies, photoperiod-induced changes in thyroid hormone availability have been proposed to influence the proliferative activity of hypothalamic progenitor cells(Reference Lewis and Ebling9).
Thus, it is tempting to speculate that inter-species differences in the seasonal regulation of thyroid hormone secretion could underlie discrepancies in photoperiod-dependent variations in tanycyte neurogenic activity. However, it must be emphasised that although a large body of evidence supports the notion that tanycytes are critical regulators of seasonal reprogramming in animal models, no studies have investigated whether these cells have a similar function in primates and human subjects. Tanycytes in the adult human hypothalamus express a number of markers of neural stem or progenitor cells, including doublecortin(Reference Batailler, Droguerre and Baroncini43), SOX2 and vimentin(Reference Pellegrino, Trubert and Terrien44). One recent study used post-mortem human tissue to investigate whether tanycytes undergo plastic changes in relation to ageing(Reference Koopman, Taziaux and Bakker45). In this study, immunohistochemical analysis of vimentin revealed qualitative differences in the morphological organisation of tanycytes between infant/prepubertal and adult subjects(Reference Koopman, Taziaux and Bakker45), offering compelling evidence that the structure and functionality of human tanycytes is highly dynamic and changes throughout life, in line with studies of age-related tanycyte plasticity in rodents(Reference Zoli, Ferraguti and Frasoldati46). The authors note that their findings reconcile with previous observations at the ultrastructural level of tanycyte end feet ensheathing GnRH terminals(Reference Prevot, Bellefontaine and Baroncini11), and discuss the contribution of plasticity in tanycytes contributing to altering GnRH secretion as oestrogen negative feedback declines at menopause. However, there is a growing consensus that human subjects display seasonal variation in their physiology, affect and susceptibility to disease, particularly when large datasets are analysed (for review see(Reference Stevenson, Visser and Arnold47)). Information on the time of year of death is not reported in the studies of human tanycyte plasticity(Reference Koopman, Taziaux and Bakker45), so further research is needed to elucidate whether alterations in tanycyte morphology and function might be associated with long-term changes in metabolic and reproductive functions in human subjects.
Conclusions
Due to the rising incidence of obesity, investigation of the central control of appetite and energy balance has received increasing attention in recent years. However, the study of the fundamental mechanisms involved in the control of energy balance has been mostly restricted to a few, conventional laboratory species. Recently, the photoperiod-dependent cyclicity of reproductive and metabolic physiology of Siberian hamsters has made them an attractive model system to understand the biology of appetite control. Studies on these seasonal mammals have revealed that the rheostatic mechanisms that mediate long-term control of energy balance are distinct from the homeostatic mechanisms that regulate short-term changes in energy intake and energy expenditures. Moreover, these studies have uncovered hypothalamic tanycytes as key contributors to long-term changes in energy balance, highlighting a number of mechanisms that could govern rheostatic adaptations to seasonal variations. Indeed, long-term cycles in ingestive behaviour could be mediated by plastic changes in hypothalamic tanycytes that in turn regulate local hypothalamic thyroid hormone availability. While the mechanisms by which these changes determine seasonal neuroendocrine transitions remain unclear, new evidence of direct tanycyte-to-neuron signalling and photoperiod-dependent changes in proliferative capacity further confirms the central role of tanycytes in seasonal reprogramming. We do not know yet whether tanycytes exert similar functions in human subjects, but given their widespread importance in seasonal vertebrates, we should be optimistic that greater understanding of tanycyte function can be translated to human metabolic dysfunction, and may identify novel targets amenable to dietary or pharmacological manipulation.
Acknowledgements
M. T. participated in writing this review as a MSci Neuroscience student at the University of Nottingham.
Financial Support
Studies in the authors’ laboratory were funded by the Biotechnology and Biological Sciences Research Council (BBSRC UK) via project grants BB/M021629/1 and BB/M001555/1.
Conflict of Interests
None.
Authorship
M. T. wrote the first draft of the manuscript. F. J. P. E. expanded and revised the manuscript. Both authors have read and approved the final manuscript.