Creatine is a key molecule for the maintenance of cellular ATP levels in cells such as those in the muscle and brain, which have high activities of creatine kinase. Creatine and phosphocreatine together with the enzyme creatine kinase act as buffers for ATP in cells. In addition, phosphocreatine is a carrier of ‘high-energy’ phosphate between sites of ATP synthesis and utilisation(Reference Wyss and Wallimann1). Creatine and phosphocreatine are spontaneously converted to creatinine at a rate of about 1·7 % per d, and this is lost in the urine(Reference Wyss and Kaddurah-Daouk2). Therefore, this quantity of creatine must be replaced for the maintenance of constant levels of creatine in the body. Creatine can be obtained from the diet by ingestion of meat and dairy products and through endogenous synthesis from amino acids.
Creatine is synthesised de novo from the metabolism of the amino acids arginine, glycine and methionine. The enzymes involved in its synthesis are arginine:glycine amidinotransferase (AGAT) and guanidinoacetate N-methyltransferase (GAMT). AGAT catalyses the transfer of the amidino group of arginine onto the N of glycine, giving ornithine and guanidinoacetate (GAA). GAMT is then responsible for the transfer of a methyl group from S-adenosylmethionine (SAM) onto the NE nitrogen of GAA, which gives creatine(Reference Komoto, Yamada and Takata3). In vertebrates, the highest activities of GAMT can be found in the liver, pancreas and brain, while the highest activities of AGAT have been measured in the kidney, pancreas and brain(Reference Van Pilsum, Stephens and Taylor4).
We have recently shown that the rat kidney can produce GAA in sufficient quantities to replace creatinine lost in the urine(Reference Edison, Brosnan and Meyer5). In addition, we have shown that the liver has a maximum capacity to methylate GAA that is far greater than that required to replace the daily loss of creatine(Reference da Silva, Nissim and Brosnan6). However, the actual hepatic methylation of GAA is limited by the delivery of GAA. What is then the function of the creatine synthetic enzymes in other tissues, such as the brain and pancreas? Creatine synthesis in the brain has been thoroughly examined by Braissant & Henry(Reference Braissant and Henry7), who suggested that the adult rat brain may be able to produce enough creatine to satisfy its own requirements. Although the pancreas has been reported to have the activities of the enzymes responsible for creatine synthesis(Reference Van Pilsum, Stephens and Taylor4), any possible role of this organ in creatine synthesis is not yet clear.
Sorenson et al. (Reference Sorenson, Stout and Brelje8) have shown that AGAT is present only in the mitochondria of pancreatic acini. When the pancreas is perfused with arginine and glycine, ornithine is released into the perfusate. These investigators concluded that ornithine and GAA, the second product of AGAT, could be transported to the liver by the portal vein. They did not look for GAMT in the pancreas, although Van Pilsum et al. (Reference Van Pilsum, Stephens and Taylor4) did find some evidence for low activity in the pancreas of a number of species.
In the present study, we determined whether the pancreas is capable of synthesising creatine from amino acids. Sorenson et al. (Reference Sorenson, Stout and Brelje8) reported that the catalytic activity and immunoreactivity of AGAT can be found in the acinar cells, which make up approximately 80 % of the cells in the pancreas(Reference Kempen, de Pont and Bonting9). Thus, we studied both the intact pancreas and isolated pancreatic acini. Since there is a known down-regulation of AGAT activity in the kidney on supplementation of a diet with creatine(Reference Guthmiller, Van Pilsum and Boen10), we also examined whether creatine could regulate the activity of enzymes involved in creatine synthesis in the pancreas.
Experimental methods
Animals
Male Sprague–Dawley rats, body weight between 250 and 350 g, were used for the experiments. All the rats were fed ad libitum and kept under a 12 h light–12 h dark cycle in a climate-controlled room. The control rats were fed the American Institute of Nutrition diet 93 for growth and lactation. For the creatine supplementation study, the experimental group was fed an American Institute of Nutrition diet 93 for growth and lactation containing 0·4 % creatine, by weight, instead of an equivalent amount of maize starch. Both the dietary treatment groups were fed for a period of 14 d. All the procedures were approved by the Memorial University of Newfoundland Institutional Animal Care Committee and were in accordance with the Guidelines of the Canadian Council of Animal Care.
Tissue collection
The rats were anaesthetised with sodium pentobarbital, intraperitoneal, at a dose of 65 mg/kg body weight. The pancreases of the rats were removed for the isolation of acini and measurement of GAMT activity as described in detail below. The kidneys and a portion of the pancreases were freeze-clamped in Al tongs pre-cooled in liquid N2 and stored at − 80 °C for the analysis of metabolites, mRNA and AGAT activity.
Isolation of pancreatic acini
Pancreatic acini were isolated according to a modification of a previously published method(Reference Blinman, Gukovsky and Mouria11). Immediately after removal from the rats, the pancreases were placed in a Petri dish where any visible adipose tissue was dissected and separated from the pancreatic tissue. The pancreases were injected with 5 ml of Solution A (Krebs–Henseleit solution containing 1·3 mm-calcium chloride, 10 mm-glucose, 2·1 mm-lactate, 0·15 mm-pyruvate, 0·2 % w/v fatty acid-free bovine serum albumin, and 135 U/ml collagenase (type 4)) and placed in a 50 ml Erlenmeyer flask, together with 25 ml of Solution A. The air in the flask was replaced with 95 % O2/5 % CO2 by gassing for 30 s, and the flask was stoppered and placed in a shaking water-bath at 37 °C for 15 min. A continuous stream of 95 % O2/5 % CO2 gas was fed through the stopper throughout the isolation procedure. After 15 min, the collagenase solution was removed, fresh Solution A (with collagenase) was added and the flask was shaken for another 15 min. This wash was repeated once more. Solution A was then removed and 10 ml of Solution B was added to the flask. Solution B had the same composition as Solution A, but with 4 % bovine serum albumin and without collagenase. The pancreases were gently teased apart by repetitive pipetting through plastic pipettes with decreasing bore sizes. The suspension was passed through a 250 μm filter and centrifuged at 200 g for 2 min. The supernatant was removed and the acini were re-suspended in fresh Solution B. This wash was repeated three times. Finally, the acini were re-suspended in Krebs–Henseleit medium containing 0·2 % bovine serum albumin. Viability was assessed by means of trypan blue exclusion.
Incubations were initiated by the addition of aliquots of the pancreatic acini suspension into pre-warmed (37 °C) 25 ml Erlenmeyer flasks containing Krebs–Henseleit medium and substrates. Acini were incubated at near-physiological concentrations of amino acid substrates (0·2 mm-arginine and 0·2 mm-glycine) or at high concentrations (2·0 mm-arginine and 2·0 mm-glycine). Methionine (0·5 mm) was used for all the incubations. The air inside each Erlenmeyer flask was replaced with 95 % O2/5 % CO2 by gassing for 20 s and sealing with a rubber stopper. Incubations were carried out at 37°C for 40 min in triplicate. Incubations were stopped by the addition of 100 μl of ice-cold 30 % (w/v) perchloric acid. Precipitated protein was pelleted at 10 000 g for 10 min at room temperature. Later, 700 μl of the supernatants were removed and neutralised with 20 μl of universal indicator, 40 μl of 50 % (w/v) K2CO3 and 20 % (w/v) KOH until the pH was between 6 and 8. After allowing the supernatants to stand in ice for 15 min, precipitated salts were removed by centrifugation at 10 000 g for 5 min at room temperature. The supernatants were then assayed for creatine as described below. Aliquots of cells that were not subjected to de-proteinisation were assayed for GAMT and AGAT activities as described below.
Enzyme activities
GAMT activity was assayed using fresh tissue according to a previously described method(Reference da Silva, Nissim and Brosnan6). AGAT activity was assayed using fresh or frozen tissue as described previously(Reference Van Pilsum, Taylor and Zakis12). Total protein in all the tissue homogenates was assayed using the Biuret method.
Real-time PCR
Frozen pancreatic tissue ( − 80 °C) was ground into a fine powder in a ceramic mortar and pestle pre-cooled with liquid N2. Approximately 100 mg of powdered tissue were placed in a sterilised microcentrifuge tube containing 500 μl of ice-cold TRIzol reagent and homogenised immediately. Total RNA was extracted using the standard TRIzol extraction protocol provided by Invitrogen. RNA quality was assessed by separation on an agarose gel and also by measuring absorbance at 260 and 280 nm using a NanoDrop spectrophotometer system (Thermo Fisher Limited). Primers were designed using the Primer3Plus online software and obtained from Integrated DNA Technologies. PCR was carried out with a Roche LightCycler using LightCycler3 software (Roche Applied Science). The AGAT forward primer was ‘CTGTGCAGCTGAAGACAAGG’ and the reverse primer was ‘CTGTGAATGGTGGGACACAG’. The GAMT forward primer was ‘ACTCATGCTTTCCGTTTGCT’ and the reverse primer was ‘AGGCACCTGAGTCTCCTCAA’. Target RNA were normalised to 18S ribosomal RNA with the forward primer ‘GTGATCCCCGAGAAGTTTCA’ and the reverse primer ‘CTGCTTTCCTCAACACCACA’.
Guanidinoacetate, creatine, phosphocreatine, ATP, S-adenosylmethionine and S-adenosylhomocysteine measurement
GAA and creatine were assayed by the HPLC method of Buchberger & Ferdig(Reference Buchberger and Ferdig13). HPLC was carried out using a Waters 600E solvent delivery system and a Waters 717 autosampler (Waters Corporation). Phosphocreatine and ATP were assayed by the enzymatic method of Lowry & Passonneau(Reference Lowry and Passonneau14). SAM and SAH were assayed as described previously(Reference Jacobs, Stead and Devlin15).
Western blotting
AGAT protein was detected using an affinity-purified anti-AGAT rabbit polyclonal antibody raised against the sequence RPDPIDWSLKYKTPDFE, amino acids 142–159 of rat AGAT (accession no. P50442-1) (Open Biosystems). The secondary antibody was a horseradish peroxidase-conjugated anti-rabbit IgG (Bio-Rad), visualised using the Immun-Star Chemiluminescence System (Bio-Rad). Band detection was carried out using an enhanced luminol system (Immun-Star, Bio-Rad, Inc.) and analysed with an Alpha Innotech ChemiImager 4400 (Alpha Innotech). Results were normalised to β-actin.
Reagents
All the reagents were purchased from Sigma Aldrich with the exception of collagenase, obtained from Worthington, and perchloric acid, obtained from Fisher Scientific. All the reagents used for PCR were purchased from Invitrogen.
Statistical analyses
Statistical analyses were carried out using the Prism GraphPad software version 3.02 (Prism Software Corporation). Data were compared using unpaired Student's t tests with the exception of the data obtained during the incubations of the isolated pancreatic acini, which were analysed using paired Student's t tests. A P value < 0·05 was considered to be significant.
Results
Regulation of creatine synthetic enzymes by dietary creatine
We examined the pancreatic activities and mRNA and protein abundance of key enzymes required for creatine synthesis in the pancreas of rats fed the creatine-free or creatine-supplemented diets. The average weights of the pancreas were 1·38 (se 0·21) and 1·47 (se 0·12) g (n 5 each) for the control and creatine-supplemented diet-fed groups, respectively. There was no significant difference between the dietary treatment groups with regard to the weight of the pancreas.
The enzyme activity and relative mRNA expression of AGAT and GAMT in the pancreas are shown in Fig. 1 along with the protein abundance of AGAT. Creatine supplementation reduced pancreatic AGAT activity by 34 %, while its protein and mRNA levels remained unchanged. Pancreatic GAMT activity and mRNA levels were not affected by dietary creatine. We were unable to measure GAMT protein levels by Western blotting due to the lack of a suitable antibody. For comparison, renal AGAT activity and protein and mRNA abundance are shown in Fig. 2. A much greater effect of dietary creatine on renal AGAT activity was observed, which was reduced by 83 % in rats fed the creatine-supplemented diet. The relative expression of AGAT mRNA and protein in the kidneys of the creatine-supplemented diet-fed rats was also reduced by 47 and 60 %, respectively.
Fig. 1 Effect of dietary creatine on pancreatic arginine:glycine amidinotransferase (AGAT) and guanidinoacetate N-methyltransferase (GAMT). (a) AGAT enzyme activity, (b) integrated density values from AGAT Western blotting, (c) relative expression of AGAT mRNA in the pancreas, (d) GAMT enzyme activity and (e) relative expression of GAMT mRNA in the pancreas. Creatine-free diet-fed group (□) and creatine-supplemented diet-fed group (). Data for mRNA target genes were normalised to 18S ribosomal RNA. Values are means (n 5), with their standard errors represented by vertical bars. * Mean values were significantly different between the dietary treatment groups (P< 0·05).
Fig. 2 Effect of dietary creatine on renal arginine:glycine amidinotransferase (AGAT). (a) Enzyme activity, (b) integrated density values from AGAT Western blotting and (c) relative expression of AGAT mRNA in the kidney. Creatine-free diet-fed group (□) and creatine-supplemented diet-fed group (). Data for mRNA target genes were normalised to 18S ribosomal RNA. Values are means (n 5), with their standard errors represented by vertical bars. * Mean values were significantly different between the dietary treatment groups (P< 0·05).
Effect of dietary creatine supplementation on metabolites in the pancreas
We examined the pancreatic content of several metabolites in rats fed the creatine-free or creatine-supplemented diets. The concentrations of these are given in Table 1. The pancreatic content of GAA, creatine and phosphocreatine was 5-fold, 3-fold and 2-fold higher, respectively, in the creatine-supplemented diet-fed rats than in the control rats. The concentration of SAM in the pancreas of the creatine-supplemented diet-fed rats was only 65 % of that observed in the control rats. The concentration of SAH as well as the SAM:SAH ratio in the pancreas was not statistically different between the dietary treatment groups.
Table 1 Metabolite concentrations in the pancreas of rats fed the creatine-free and creatine-supplemented diets (Mean values with their standard errors, n 5)
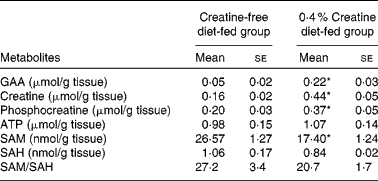
GAA, guanidinoacetate; SAM, S-adenosylmethionine; SAH, S-adenosylhomocysteine.
* Mean values were significantly different between the dietary treatment groups (P< 0·05).
Creatine synthesis in pancreatic acini
The activities of AGAT and GAMT in pancreatic acini are summarised in Table 2. It can be seen that GAMT activity was only 2·3 % of AGAT activity. The rates of GAA production in the isolated pancreatic acini are shown in Fig. 3. Pancreatic acini produced significant quantities of GAA in the presence of physiological concentrations of methionine (0·5 mm), glycine (0·2 mm) and arginine (0·2 mm). In the presence of high concentrations of arginine and glycine (2 mm of each), GAA production in the pancreatic acini was approximately 8-fold higher than that observed in the presence of near-physiological concentrations of these amino acids. The pancreatic acini were capable of synthesising about the same amount of creatine and GAA at physiological substrate concentrations, although the rates of creatine synthesis were much lower than those of GAA synthesis at higher substrate concentrations. The capacity for creatine and GAA production in pancreatic acini, therefore, is evenly matched at physiological concentrations, but the capacity for GAA production is greater than that for GAA methylation (creatine production) at elevated substrate concentrations.
Table 2 Activities of arginine:glycine amidinotransferase (AGAT) and guanidinoacetate N-methyltransferase (GAMT) in the isolated pancreatic acini (Mean values with their standard errors, n 5)
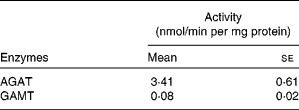
Fig. 3 Guanidinoacetate (GAA) and creatine production by acini isolated from the rat pancreas. (a) GAA production and (b) creatine production at time zero (□), after 40 min of incubation with 0·2 mm-glycine and 0·2 mm-arginine (), and after 40 min of incubation with 2 mm-glycine and 2 mm-arginine (
). Values are means (n 5), with their standard errors represented by vertical bars. * Mean values was significantly different between the 40 min incubations (P< 0·05).
Discussion
Significant AGAT activity and GAA production were observed in the pancreatic acini. This was in agreement with the immunohistochemical localisation of AGAT protein exclusively in the pancreatic acini(Reference Sorenson, Stout and Brelje8) and the determination of AGAT activity(Reference Van Pilsum, Stephens and Taylor4) in the pancreas. AGAT has high K m values for arginine (approximately 1–3 mm) and glycine (approximately 2–3 mm)(Reference Wyss and Kaddurah-Daouk2). This was consistent with the increase in GAA production when the concentrations of these amino acids were increased from 0·2 to 2 mm. The sensitivity to arginine and glycine levels suggests that the transport of these substrates into pancreatic acini is not limiting for the synthesis of GAA in the pancreas and hence that plasma substrate concentrations influence GAA synthesis. However, creatine production in the pancreatic acini did not increase at higher substrate concentrations. The low activity of GAMT relative to the activity of AGAT may account for this observation. Thus, from the rates of GAA and creatine production and the enzyme activities measured in the pancreatic acini, we concluded that the capacity for GAA synthesis in the pancreas is much higher than that for creatine synthesis.
To gain a better insight into the potential impact that the pancreas might have on total body creatine synthesis in rats, we made some calculations of pancreatic creatine and GAA production. We calculated the rate of creatine synthesis in the presence of 0·2 mm-arginine, 0·2 mm-glycine and 0·5 mm-methionine of 4·4 μmol of creatine/d per 250 g rat tissue. This compares with the measured loss of creatinine of 55 μmol/d per 250 g rat tissue, which is equivalent to the daily renal GAA production(Reference Edison, Brosnan and Meyer5). Thus, the pancreas could produce as much as 8 % of the creatine that is lost per d. A similar calculation of GAA production yielded a rate of pancreatic GAA production of 8·3 μmol of GAA/d per 250 g rat (15 % renal GAA production). From the rates of GAA and creatine production and the enzyme activities measured in the pancreatic acini, we concluded that the capacity for GAA synthesis in the pancreas is much higher than that for creatine synthesis. Given that pancreatic venous blood drains into the hepatic portal vein and flows through the liver, it is possible that the pancreas releases GAA into the plasma to be used for creatine synthesis in the liver.
Phosphocreatine has been shown to be present in isolated perfused rat pancreas(Reference Matsumoto, Kanno and Seo16) and to be dynamic, changing during acetylcholine-induced secretion of zymogens. ATP is known to be required for the secretion of zymogens by the exocrine pancreas(Reference Petersen17); ATP levels are thought to be maintained by creatine kinase phosphorylation of ADP from phosphocreatine(Reference Matsumoto, Kanno and Seo16).
Although the cells of the endocrine pancreas only represent a small fraction of the total cells, phosphocreatine is also important during secretion by these cells. Substantial creatine kinase activity has been found in islets isolated from mouse pancreas(Reference Panten, Zünkler and Scheit18), and phosphocreatine has been shown to increase the response of the KATP channels in rat pancreatic islets(Reference Krippeit-Drews, Bäcker and Düfer19). KATP channels are integral to the mechanism of the release of insulin from the β-cells of the pancreas. A recent study carried out by Rocić et al. (Reference Rocić, Lovrencić and Poje20), which supports the role of the creatine kinase system in β-cells, has shown that the provision of creatine in the presence of various concentrations of glucose significantly increases ATP levels. In addition, Rocić et al. (Reference Rocić, Lovrencić and Poje20) have shown that insulin secretion is increased by the provision of creatine, independent of the presence of added glucose, which suggests that changes in pancreatic creatine levels alone may impact insulin secretion by the β-cells. Marco et al. (Reference Marco, Calle and Hedo21) have shown that creatine, GAA and arginine (at quite high concentrations) can significantly increase the secretion of glucagon and insulin in isolated pancreatic mouse islets. The mechanism through which guanidino compounds affect the release of hormones from pancreatic islets remains to be elucidated. However, there is good evidence from studies using inside-out patch clamps of mouse pancreatic β-cells that the addition of phosphocreatine prevents the opening of KATP channels similar to the effect of added ATP, suggesting that phosphocreatine is involved in the regulation of insulin secretion through the modulation of ATP and ADP levels(Reference Schulze, Düfer and Wieringa22).
Dietary creatine supplementation increased creatine and phosphocreatine content in whole pancreas. Thus, the pancreatic cells must be capable of transporting creatine. We predict that pancreatic islet cells would have SLC6A8, the well-known Na+-dependent creatine transporter. Pancreatic acini should have a creatine efflux transporter other than SLC6A8, since the Na gradient is in the wrong direction. A similar non-SLC6A8 transporter must be present in hepatic cells(Reference Speer, Neukomm and Murphy23). It is possible that high creatine concentrations in the plasma could enter the pancreas by reversal of this postulated transporter. The resultant increase in phosphocreatine concentrations presumably reflects the action of the reversible creatine kinase. It is not known whether such an increase affects exocrine and/or endocrine secretion.
The concentrations of GAA were increased in the pancreas of the creatine-supplemented diet-fed rats. This observation would appear to be at odds with the decreased AGAT activity observed in these rats, but an increase in the concentrations of GAA was also observed in the kidney (control group: 0·089 (se 0·009); creatine-treated group: 0·111 (se 0·012) μmol/g, n 6, P< 0·01). Tissues that produce GAA would need a transporter to move it from the cells into the plasma. No transporter has been recognised yet, but it is possible that high creatine concentrations in the plasma may inhibit the movement of GAA out of the cells so that it accumulates, even at the lower AGAT activity observed in the pancreas and kidney. If so, this is additional evidence that pancreatic acini release GAA into the plasma.
We observed a high ratio of SAM:SAH in the pancreas, indicating that there is a high methylation potential in this organ. The pancreatic SAM:SAH ratio was 6-fold higher than that reported by us for the liver(Reference Jacobs, Stead and Brosnan24). There is also evidence that the pancreas has a very active methionine cycle. Recently, Wilson et al. (Reference Wilson, van den Borne and Calder25) have shown, in a study using metabolic tracers, that among a comprehensive list of organs, the pancreas exhibits the highest production of homocysteine from methionine (per gram of tissue). Creatine supplementation appeared to have an effect on pancreatic methylation potential as the concentrations of SAM were significantly lower in the pancreas of the creatine-supplemented diet-fed rats. Since the activities of the methyltransferases would be decreased in the presence of lower SAM concentrations(Reference Clarke, Banfield, Carmel and Jacobsen26), these data suggest that dietary creatine supplementation may have an impact on methylation in the pancreas.
The effect of dietary creatine on AGAT expression and activity in the pancreas appears to be different from that observed in the kidney. We found that dietary creatine reduces AGAT activity in the pancreas by about 34 %. In addition, we found that dietary creatine supplementation did not have an effect on AGAT mRNA and protein expression in the pancreas. This is quite different from what we observed in the kidney. Dietary creatine supplementation significantly decreased renal AGAT activity and protein and mRNA expression. This confirms the finding of the study carried out by McGuire et al. (Reference McGuire, Gross and Van Pilsum27), who have shown that both renal AGAT activity and mRNA expression are significantly reduced by 74 and 63 %, respectively, by dietary creatine supplementation. Our data indicate that the pre-translational mechanism of regulation that responds to dietary creatine in the kidney may not occur in the pancreas.
In summary, we showed that pancreatic acini have activities of both AGAT and GAMT enzymes and are capable of producing GAA and creatine de novo. The capacity for GAA synthesis at elevated substrate concentrations is greater than that for creatine synthesis. We predict that some GAA may be secreted into the portal vein by the pancreatic acini. In addition, we showed the different effects of dietary creatine supplementation on AGAT activity and expression in the pancreas and kidney, indicating that the mechanisms of the regulation of AGAT activity in the pancreas may be differ from those in the pancreas.
Acknowledgements
The present study was funded by grants from the Canadian Institutes for Health Research (to J. T. B. and M. E. B.). R. P. d. S. was supported by a fellowship from the School of Graduate Studies at Memorial University of Newfoundland. The Canadian Institutes for Health Research had no role in the design and analysis of the study or in the writing of this article.
The authors' contributions were as follows: R. P. d. S., J. T. B. and M. E. B. designed the research; R. P. d. S. and K. C. conducted the research; R. P. d. S. analysed the data; R. P. d. S. and M. E. B. wrote the paper and had primary responsibility for the final content. All authors read and approved the final manuscript.
The authors have no conflicts of interest to disclose.