1. Introduction
In 2023, the world took a critical look at progress toward collectively delivering on the Paris Agreement, with the first Global Stocktake, completed at COP28. At this key moment in international climate negotiations, science is already clear that a course-correction from current greenhouse gas (GHG) emission trends is needed. As we move deeper into this crucial decade for action on climate change, it is vital that the latest science on climate change and its impacts be readily available and accessible for all those involved in international negotiations, national policymaking, private sector decision-making, and civil society mobilization. At the heart of the United Nations Framework Convention on Climate Change (UNFCCC) process, the Intergovernmental Panel on Climate Change (IPCC) is responsible for assessing the science underpinning the levers and scale of needed climate action. Through periodic, extensive assessments of the scientific literature, the IPCC is the most authoritative voice on the state of knowledge on climate change. The latest cycle of the IPCC concluded this year with the Sixth Assessment Report (AR6) (IPCC, 2021, 2022a, 2022b, 2023). Through these reports, the IPCC informs stakeholders not only about the current scientific understanding on climate change, associated impacts, risks, and solution space, but also the pace of climate actions under the international pledges and agreements.
Given the thematic breadth and procedural demands of the IPCC assessments, each cycle takes several years to complete. For example, more than 8 years passed between the release of the AR5 Synthesis Report (SYR) and the AR6 SYR. One obvious limitation of this setup is that in the multi-year periods between the release of major IPCC reports, negotiators and decision-makers are in need of an authoritative source of scientific advances, which otherwise remain less visible and accessible to them. Furthermore, while the AR6 SYR was published this year (2023), the cut-off point for the scientific literature assessed by the three work groups was more than 2 years before that. The ‘10 New Insights in Climate Science’ series (https://10insightsclimate.science/), a collaboration between Future Earth, The Earth League, and the World Climate Research Programme, contributes to fill this gap each year. Starting in 2017, 3 years after the publication of the AR5 SYR, and every year since, the ‘10 New Insights’ report has been published to highlight essential advances in climate change research from the prior year, from both natural and social sciences, and with high policy relevance. The aim of this international collaboration is to provide a timely and curated set of synthesized science-based ‘insights’ for negotiators, decision-makers, and other stakeholders, to stay up-to-date and grounded in the latest peer-reviewed research. Since 2020, the report has been developed on the basis of a peer-reviewed academic paper (Martin et al., Reference Martin, Alcaraz Sendra, Bastos, Bauer, Bertram, Blenckner, Bowen, Brando, Brodie Rudolph, Büchs, Bustamante, Chen, Cleugh, Dasgupta, Denton, Donges, Donkor, Duan, Duarte and Woodcock2021, Reference Martin, Boakye, Boyd, Broadgate, Bustamante, Canadell, Carr, Chu, Cleugh, Csevar, Daoudy, de Bremond, Dhimal, Ebi, Edwards, Fuss, Girardin, Glavovic, Hebden and Zhao2022; Pihl et al., Reference Pihl, Alfredsson, Bengtsson, Bowen, Broto, Chou, Cleugh, Ebi, Edwards, Fisher, Friedlingstein, Godoy-Faúndez, Gupta, Harrington, Hayes, Hayward, Hebden, Hickmann, Hugelius and Zelinka2021), providing a more solid foundation and strengthening the messages.
Every year, there is a plethora of relevant reports on different aspects of climate change and climate action, including the State of the Global Climate (WMO, 2023b), the Emissions Gap Report (UNEP, 2022a), Adaptation Gap Report (UNEP, 2022b), the Global Carbon Budget (Friedlingstein et al., Reference Friedlingstein, O'Sullivan, Jones, Andrew, Gregor, Hauck, Le Quéré, Luijkx, Olsen, Peters, Peters, Pongratz, Schwingshackl, Sitch, Canadell, Ciais, Jackson, Alin, Alkama and Zheng2022), and United in Science (2022). Generally, these well-recognized reports are focused on updating diagnostic indicators that are familiar to those involved in or following climate negotiation. The ‘10 New Insights’ report links recent research advances to those categories, but also seeks to inform stakeholders about a wider range of topics across the full landscape of climate change research and their interactions, including many that are likely to be less familiar to the climate negotiations audience, though not less relevant. The ‘10 New Insights’ 2023 report is also intended as a resource for researchers interested in having a better understanding of developments in different areas of climate change research beyond their domain of expertise, and their policy implications.
We define a ‘New Insight’ as a recent development or advance in a particular area of climate change research. The insight can be the result of discrete new evidence or analyses that significantly updates previous understanding with regard to processes or patterns of climate change, including impacts and the possible means for addressing the climate emergency. Insights can also be ‘emerging developments’ (i.e. novel topics and research questions) gathering recognition in the field and important issues on the horizon of climate change research. In this latter case, the advance might be more conceptual in nature. To be considered recent, these developments or advances must be anchored in peer-reviewed literature published in 2022 and 2023.
This year we highlight the looming inevitability of overshooting the 1.5°C global warming limit target (insight 1), a situation that will not only mean an increase in risks, but also significant uncertainty. In this context, we call attention to the fast-shrinking carbon budget and emphasize the inescapable necessity of a rapid and managed fossil fuel phase-out (insight 2). Given the significance of carbon dioxide removal (CDR) to these two issues, we outline the challenges for up-scaling, accounting, and governance of CDR (insight 3). We cast light on key uncertainties regarding the future of natural carbon sinks in land and oceans as warming levels rise (insight 4). This adds to the urgency for decarbonizing our economy and being clear-sighted about the realistic role of CDR methods.
We stress the intertwinedness of the crises of biodiversity loss and climate change, and the need for joint governance and synergistic approaches to confront them (insight 5). Impacts and vulnerabilities continue to increase, and this year we feature science advances regarding compound events (insight 6) and the acceleration of mountain glacier loss (insight 7). Much confusion persists regarding the complex relationship between climate change and human mobility; we covered several aspects of this relationship in 2022, and this year we devote one insight to immobility in the face of climate risks (insight 8). Insight 9 synthesizes recent advances to conceptualize and evaluate justice in adaptation planning and the key role of locally led adaptation (LLA) efforts. Finally, insight 10 centers on the call from researchers to integrate just transitions in food system transformations as a necessary condition for realizing their mitigation potential while assuring food security and nature conservation.
2. Method
The process for selecting a set of 10 insights for 2023 started with an open call for input distributed primarily across the partners' institutional networks, reaching experts globally. Contributions were collected through an online questionnaire, in which the main question was: What is a key recent advance in climate change research that you think should be highlighted for policymakers? The respondents were also asked to provide peer-reviewed references, published in 2022 and 2023, that support the suggested ‘key research advance’. The call for input was open from January 23 to February 20, and we received 167 entries from 131 individual respondents. The entries were screened based on predefined inclusion criteria, with each individual entry screened by two team members, at least. Discrepancies were further discussed among the project team to reach a final decision. When necessary, project coordinators completed one additional round of screening and made a final decision. Seventy-one entries met the inclusion criteria. After merging closely related entries, the list was reduced to 43 themes and was coded using a thematic framework based on prior ‘10 New Insights’ reports. This list was complemented with a literature scan of impactful papers in climate change research published in the same period (2022 and the first months of 2023), which yielded 23 additional themes. The final list of 66 themes was then evaluated in a three-stage process by a group of 24 very well-established international researchers on climate change from different disciplines, who constitute our Editorial Board. First, each editorial board member selected 4–20 themes considered most relevant (1–5 in each of the four broad categories: the Earth system, impacts, action needed, and barriers). Second, building on the outcomes of the individual prioritization of themes, in a virtual workshop the editorial board members collectively revised the priority themes, leading to a preliminary set of close to 10 candidate insights. Finally, each of the candidate insights was examined more deeply, providing the input for a second workshop of the editorial board, in which a final list of the 10 insights was decided on, through deliberation. Once the 10 insights were outlined, 10 international groups, of four–six experts each, were formed. Each group was tasked with synthesizing the key messages from recent academic literature and their policy implications. (In the Supplementary material, we provide (1) a flow diagram of the process described above, (2) the questionnaire used for the open call, (3) brief characterization of the respondents to the questionnaire, (4) inclusion criteria for entries, (5) complementary literature scan, and (6) the list of resulting themes, and a brief account of the relative contribution to the final set of 10 insights obtained from the open call for input and from the complementary literature scan.)
3. Results
3.1 Insight 1. Overshooting 1.5°C is fast becoming inevitable, greatly increasing risks as mitigation action is delayed
The IPCC AR6 found that global warming is likely or very likely to exceed 1.5°C relative to the pre-industrial era in the near term (before 2040) under all but the very low GHG emission scenarios (SSP1–1.9). Few pathways remain that avoid a 1.5°C overshoot; pathways with no or limited overshoot require emissions to peak before 2025 and be cut by 43% by 2030 relative to 2019 levels (IPCC, 2022b, Ch3, p. 329), representing a 6% decrease each year. Research published after the release of the AR6 indicates that overshooting 1.5°C is all but inevitable in the near term, based on assessments of:
(a) The remaining carbon budget (RCB) (Friedlingstein et al., Reference Friedlingstein, O'Sullivan, Jones, Andrew, Gregor, Hauck, Le Quéré, Luijkx, Olsen, Peters, Peters, Pongratz, Schwingshackl, Sitch, Canadell, Ciais, Jackson, Alin, Alkama and Zheng2022) and emission trends: fossil carbon dioxide (CO2) emissions in 2022 were 1% higher than that in 2021. The RCB for 1.5°C is currently estimated to be 250 Gt CO2 (50% likelihood) (Forster et al., Reference Forster, Smith, Walsh, Lamb, Lamboll, Hauser, Ribes, Rosen, Gillett, Palmer, Rogelj, von Schuckmann, Seneviratne, Trewin, Zhang, Allen, Andrew, Birt, Borger and Zhai2023), and will be used in 6–7 years based on current annual GHG emissions.
(b) New evidence on the geophysical warming commitment inherent in the climate system: Global climate modeling indicates that there is a 42% probability that the world is committed to peak global warming of at least 1.5°C based on past emissions alone (Dvorak et al., Reference Dvorak, Armour, Frierson, Proistosescu, Baker and Smith2022).
(c) The most recent emission reduction pledges put forward by countries: Modeling indicates the Paris Agreement target of 1.5°C will be exceeded shortly after 2030 even under the most ambitious emission pledge scenarios (Meinshausen et al., Reference Meinshausen, Lewis, McGlade, Gütschow, Nicholls, Burdon, Cozzi and Hackmann2022).
(d) The carbon lock-in and inertia of the global energy sector, responsible for about three-quarters of current emissions (IEA, 2023a): Proposed and existing large fossil fuel projects would produce emissions of up to twice the carbon budget for 1.5°C (Kühne et al., Reference Kühne, Bartsch, Tate, Higson and Habet2022) (see insight 2 on fossil fuel phase-out).
If overshooting 1.5°C is all but inevitable, it is essential that policymakers and citizens are informed appropriately about the factors that determine the peak temperature and duration of the overshoot, and the risk implications during the time of overshoot. There is real risk that temperatures might not be brought down, mainly due to the scale of net-negative emissions required, which may not be feasible, and/or their costs and impacts seen as unacceptable (see insight 3). The risks of overshooting 1.5°C will inform the urgency and scale of near-term adaptation and mitigation efforts. In that regard, every fraction of a degree of warming greatly matters (Box 1).
Box 1. Definition of 1.5°C overshoot
The IPCC (2021, Annex VII, p. 2251) defines temperature overshoot as the exceedance of a specified level of global warming, followed by an eventual return. Global warming, which the Paris Agreement aims to limit this century to 1.5°C, refers to increases in global mean surface temperature with respect to the pre-industrial era (1850–1900), averaging over a period long enough to remove interannual variations (e.g. 20 or 30 years). Breaching 1.5°C in any given year is expected to become more frequent in the upcoming decades (WMO, 2023a).
Warming is near-linearly related to cumulative CO2 emissions (IPCC, 2021, TS, p. 55 and Ch5, p. 742). Hence, the peak warming during overshoot is determined by the extent to which global CO2 emissions accumulated over time exceed the carbon budget for 1.5°C. Scenarios assuming continuation of current policies project a warming of 2.6°C (1.9–3.7°C) (Meinshausen et al., Reference Meinshausen, Lewis, McGlade, Gütschow, Nicholls, Burdon, Cozzi and Hackmann2022) and 2.1–2.4°C (van der Ven et al., Reference van der Ven, Mittal, Gambhir, Lamboll, Doukas, Giarola, Hawkes, Koasidis, Köberle, McJeon, Perdana, Peters, Rogelj, Sognnaes, Vielle and Nikas2023) by the end of the century. Assessments of emission trajectories that assume all countries fulfill their short- and long-term climate pledges project 1.9–2.0°C (Meinshausen et al., Reference Meinshausen, Lewis, McGlade, Gütschow, Nicholls, Burdon, Cozzi and Hackmann2022) and 1.7–1.8°C warming (van der Ven et al., Reference van der Ven, Mittal, Gambhir, Lamboll, Doukas, Giarola, Hawkes, Koasidis, Köberle, McJeon, Perdana, Peters, Rogelj, Sognnaes, Vielle and Nikas2023) by 2100. This shows that current pledges are insufficient to avoid overshooting 1.5°C, while current policy actions are even insufficient for keeping warming below 2°C.
Recent studies converge on the importance of implementing current pledges up to 2030 to limit peak warming closer to 1.5°C, as well as ratcheting ambition in the long term (Meinshausen et al., Reference Meinshausen, Lewis, McGlade, Gütschow, Nicholls, Burdon, Cozzi and Hackmann2022; van der Ven et al., Reference van der Ven, Mittal, Gambhir, Lamboll, Doukas, Giarola, Hawkes, Koasidis, Köberle, McJeon, Perdana, Peters, Rogelj, Sognnaes, Vielle and Nikas2023). Although early mitigation presents near-term challenges, it is less costly over the long term (see insight 3 on CDR). Postponing mitigation until after 2030 comes with higher and persistent feasibility concerns, notably due to volatility and uncertainty caused by higher climate impacts (Brutschin et al., Reference Brutschin, Pianta, Tavoni, Riahi, Bosetti, Marangoni and Ruijven2021), particularly in the non-Organization for Economic Cooperation and Development (non-OECD) countries (Bauer et al., Reference Bauer, Keller, Garbe, Karstens, Piontek, von Bloh, Thiery, Zeitz, Mengel, Strefler, Thonicke and Winkelmann2023).
Achieving net-zero CO2 emissions is necessary for containing the peak warming level. The world's ability to bring temperature down to specific goals after overshoot depends on removing more CO2 from the atmosphere than is emitted: achieving net-negative CO2 emissions. If achieved (and this is uncertain due to unresolved, fundamental questions regarding CDR; see insight 3), there may still be a delay of several years before the climate cools, due to lags in the carbon cycle and thermal response (IPCC, 2021, Ch4, p. 624 and Ch5, p. 775).
Warming reversal may also be delayed if overshoot triggers the release of GHGs from natural carbon sinks in ways not yet modeled, or not yet anticipated. Such impacts are already being observed (IPCC, 2022a, Ch2.4 and Ch2.5; see insight 4). This uncertainty is represented by the red shaded area in Figure 1.

Figure 1. Overshoot and non-overshoot scenarios. Stylized representation of a temperature overshoot scenario (red line) and its risks after reaching net-zero CO2 emissions in comparison to a non-overshoot scenario (yellow line) stabilizing at the target temperature of 1.5°C by 2100. The temperature of the overshoot pathway may not return to 1.5°C on reaching the same cumulative emissions as the non-overshoot scenario due to feedbacks and response lags in the Earth system components. The associated uncertainty of global mean temperature reversibility after the overshoot, together with simultaneous regional climate irreversibility, mean that overall only ‘partial reversibility’ might be possible. Note that the tipping elements at risk of instability in the upper panel only relate to the global warming levels axis, not to the time axis. Data for the tipping element risk assessment are taken from Armstrong McKay et al. (Reference Armstrong McKay, Staal, Abrams, Winkelmann, Sakschewski, Loriani, Fetzer, Cornell, Rockström and Lenton2022).
Breaching the 1.5°C target over decades would leave a long-lasting legacy on the Earth system, since some aspects of the climate and wider environment will not recover, within human-relevant timescales of decades to a century, to the same state as in a reference scenario without overshoot. This is mainly due to the slow response time of key Earth system components. Surface air temperature and precipitation changes appear largely reversible at the global scale following a decline in atmospheric CO2, but they exhibit irreversibility at regional scale on a timescale of centuries, posing a greater risk to human and natural systems in regions of irreversibility (Kim et al., Reference Kim, Shin, An, Kim, Im, Xie, Kug and Yeh2022; Oh et al., Reference Oh, An, Shin and Kug2022). In the long term, irreversible changes include sea-level rise from ocean thermal expansion and melting of ice sheets and glaciers, sea-ice loss, changes in the deep sea environment (e.g. oxygen, acidity), and changes in structure and composition of terrestrial ecosystems that affect carbon uptake and losses, including permafrost carbon loss (Bauer et al., Reference Bauer, Keller, Garbe, Karstens, Piontek, von Bloh, Thiery, Zeitz, Mengel, Strefler, Thonicke and Winkelmann2023; IPCC, 2021, Ch4.6.2.1; IPCC, 2022a, Ch2.5.2.10; Schwinger et al., Reference Schwinger, Asaadi, Steinert and Lee2022).
Spatially heterogeneous and potentially irreversible impacts, such as more frequent heatwave exposure with subsequent economic damages as well as mass mortality of species, worsen with higher peak and duration of overshoot (Bauer et al., Reference Bauer, Keller, Garbe, Karstens, Piontek, von Bloh, Thiery, Zeitz, Mengel, Strefler, Thonicke and Winkelmann2023; Meyer et al., Reference Meyer, Bentley, Odoulami, Pigot and Trisos2022). Irreversible impacts can be most clearly identified for marine biodiversity, with species facing the added pressure of prolonged ocean acidification after peak overshoot (Meyer et al., Reference Meyer, Bentley, Odoulami, Pigot and Trisos2022).
Lastly, there is considerable risk that a long overshoot period above 2°C could trigger self-perpetuating feedbacks associated with climate tipping elements, such as instabilities of the Greenland or West Antarctic ice sheets or loss of mountain glaciers (Armstrong McKay et al., Reference Armstrong McKay, Staal, Abrams, Winkelmann, Sakschewski, Loriani, Fetzer, Cornell, Rockström and Lenton2022; Wunderling et al., Reference Wunderling, Winkelmann, Rockström, Loriani, Armstrong McKay, Ritchie, Sakschewski and Donges2023), which would be largely irreversible on timescales of centuries to millennia. Impacts of triggering these tipping elements include several meters of sea-level rise in the long term, causing loss of land, livelihoods, and cultural heritage in coastal communities and small island states, and irreversible degradation of mid-latitude coral reef species (Bauer et al., Reference Bauer, Keller, Garbe, Karstens, Piontek, von Bloh, Thiery, Zeitz, Mengel, Strefler, Thonicke and Winkelmann2023; Meyer et al., Reference Meyer, Bentley, Odoulami, Pigot and Trisos2022). All these risks increase severely with the extent of overshoot above 1.5°C. If the 1.5°C goal is missed, decision-makers should continue to strive to limit warming to as close to 1.5°C as possible and minimize the duration of overshoot. Further studies are urgently needed to investigate the direct and indirect impacts of overshoot to inform policy and action.
3.2 Insight 2. Fast-shrinking carbon budget calls for a managed and equitable fossil fuel phase-out
Fossil fuels are the largest cause of climate change, accounting for close to 90% of global CO2 emissions (Friedlingstein et al., Reference Friedlingstein, O'Sullivan, Jones, Andrew, Gregor, Hauck, Le Quéré, Luijkx, Olsen, Peters, Peters, Pongratz, Schwingshackl, Sitch, Canadell, Ciais, Jackson, Alin, Alkama and Zheng2022). The ‘committed emissions’ that would occur over the lifetime of already-existing infrastructure used in extraction and consumption of fossil fuels are estimated to exceed the remaining carbon budget (RCB) for a 50% chance of limiting warming to 1.5°C (Tong et al., Reference Tong, Zhang, Zheng, Caldeira, Shearer, Hong, Qin and Davis2019; Trout et al., Reference Trout, Muttitt, Lafleur, Van de Graaf, Mendelevitch, Mei and Meinshausen2022; see Figure 2). Investments in new fields, mines, power plants, heating systems, and other long-lived fossil fuel infrastructure are thus inconsistent with pathways to keep the 1.5°C goal within reach (IEA, 2023c; IISD, 2022) and risk creating trillions of dollars of stranded assets (see Box 2). Furthermore, they create ‘carbon lock-in’: it is harder to stop fossil fuel projects from operating once they are built, since companies tend to pursue full-lifetime use of assets to recover sunk investment. Yet, governments and companies still plan on extracting vastly more fossil fuels than is consistent with the 1.5°C warming limit target, thereby creating a so-called ‘production gap’ (Rekker et al., Reference Rekker, Chen and Heede2023; SEI et al., 2023). Government subsidies for fossil fuel production and use also reached an all-time high of 1 trillion USD in 2022 (IEA, 2023b).
Box 2. Early retirement, asset stranding, and lock-in
A key problem for a fast fossil fuel phase-out is the long operational lifetimes of many fossil fuel assets, ranging from less than 20 years for vehicles to 60 years for infrastructure. Limiting global warming to 1.5°C will require a substantial amount of existing fossil fuel infrastructure to be retired early, shortening these lifetimes, and/or running assets below their normal capacity (Figure 2). Asset stranding occurs when such early retirement, or devaluation of the asset's product (e.g. a structurally lower oil price), is unanticipated at the time a firm invests building the asset, thus missing the firm's expected rate of return, and leading to income losses. Financial assets that derive their value from physical fossil fuel assets, such as shares in a fossil fuel company, can also strand (Semieniuk et al., Reference Semieniuk, Holden, Mercure, Salas, Pollitt, Jobson, Vercoulen, Chewpreecha, Edwards and Viñuales2022). This can happen earlier than physical asset stranding and might happen abruptly if enough investors adjust their expectations of future returns downward at the same time. Such a ‘green swan’ event substantially devalues stocks, which in turn could affect macro-financial stability (Campiglio & van der Ploeg, Reference Campiglio and van der Ploeg2022). Carbon lock-in is when an incumbent set of infrastructure, institutions, and behaviors creates inertia that make it harder for clean energy to compete and replace fossil fuels (Kemfert et al., Reference Kemfert, Präger, Braunger, Hoffart and Brauers2022). For example, income and wealth losses of fossil fuel companies and financial beneficiaries due to asset stranding may lead to their opposition to mitigation policies.

Figure 2. Committed CO2 emissions from fossil fuel infrastructure compared to carbon budgets reflecting the Paris Agreement goals. Bars show future emissions arising from full-lifetime operation of fossil fuel consuming infrastructure (Tong et al., Reference Tong, Zhang, Zheng, Caldeira, Shearer, Hong, Qin and Davis2019) and of fossil fuel extracting infrastructure (Trout et al, Reference Trout, Muttitt, Lafleur, Van de Graaf, Mendelevitch, Mei and Meinshausen2022), also showing proposed ‘carbon bombs’, defined as fossil fuel extraction projects whose lifetime emissions exceed 1 Gt CO2 (Kühne et al, Reference Kühne, Bartsch, Tate, Higson and Habet2022). These are compared with the remaining carbon budget (Friedlingstein et al., Reference Friedlingstein, O'Sullivan, Jones, Andrew, Gregor, Hauck, Le Quéré, Luijkx, Olsen, Peters, Peters, Pongratz, Schwingshackl, Sitch, Canadell, Ciais, Jackson, Alin, Alkama and Zheng2022) updated for early 2023 (Forster et al., Reference Forster, Smith, Walsh, Lamb, Lamboll, Hauser, Ribes, Rosen, Gillett, Palmer, Rogelj, von Schuckmann, Seneviratne, Trewin, Zhang, Allen, Andrew, Birt, Borger and Zhai2023). While the estimates for existing infrastructure are comprehensive, those for proposed infrastructure are partial due to lack of available data on consuming infrastructure in industry, transport, buildings, or other, or on smaller future extraction projects. ‘Existing’ generally means that capital has been invested or committed, as at the start of 2018 (see original papers for further details on methods). New infrastructure built since then will likely exceed retirements and reductions in remaining life, hence an updated estimate would likely be larger. Since the infrastructure estimates for extraction and consumption relate to different ends of the supply chain, they are non-additive: each carbon atom passes through both extraction and combustion stages. While amounts of extraction and consumption are equal in any given year (apart from minor changes in storage), the committed emissions differ due to different amounts and lifetimes of capital stock of the types of infrastructure.
Fossil fuel expansion is also perpetuated by financial actors through their investments and company ownership. Potential economic losses from stranded assets in upstream oil and gas are estimated upward of 1 trillion USD, held predominantly in the Global North through cross-border financial stakes in oil and gas fields elsewhere (Semieniuk et al., Reference Semieniuk, Holden, Mercure, Salas, Pollitt, Jobson, Vercoulen, Chewpreecha, Edwards and Viñuales2022). Stranded assets can have macroeconomic consequences by affecting the valuation of other assets, that is, contagion and triggering spillovers from the financial to the real economy (Campiglio & van der Ploeg, Reference Campiglio and van der Ploeg2022). Governments are directly exposed through state-owned companies and reduced taxes and royalties, and indirectly through commitments to bailing out private fossil fuel investors or stabilizing the financial system. New government policies and explicit planning are thus needed to facilitate a rapid and managed phase-out of fossil fuel production and use in line with achieving net-zero CO2 emissions by 2050 (Grubert & Hastings-Simon, Reference Grubert and Hastings-Simon2022; IEA, 2023c).
A managed approach is needed, which entails preventing new facilities being built (IEA, 2023c), establishing timelines for phasing-out existing facilities (Trencher et al., Reference Trencher, Rinscheid, Rosenbloom and Truong2022), creating financial mechanisms for an orderly wind-down (GFANZ, 2022), coordinating actions on fossil fuel supply and demand to avoid price volatility (Diluiso et al., Reference Diluiso, Walk, Manych, Cerutti, Chipiga, Workman, Ayas, Cui, Cui, Song, Banisch, Moretti, Callaghan, Clarke, Creutzig, Hilaire, Jotzo, Kalkuhl, Lamb and Minx2021), ensuring a just transition for workers and communities, and fairly allocating efforts between countries (Muttitt & Kartha, Reference Muttitt and Kartha2020).
A global phase-out of fossil fuels faces several barriers. First, fossil fuels are intertwined with geopolitics and concerns over energy security (Espagne et al., Reference Espagne, Oman, Mercure, Svartzman, Volz, Pollitt, Semieniuk and Campiglio2023). Europe's dramatic reduction of Russian gas imports following the invasion of Ukraine sparked a new dash for gas supplies and, in some cases, heightened reliance on coal power. Second, pressing energy and economic needs in developing countries are driving increased fossil fuel consumption and production (Saha & Carter, Reference Saha and Carter2022). The need to leapfrog to clean energy systems is hampered by insufficient international finance (Pachauri et al., Reference Pachauri, Pelz, Bertram, Kreibiehl, Rao, Sokona and Riahi2022). Additionally, exporter countries continue to depend on fossil fuel revenues, making it hard to transform their economies (Muttitt & Kartha, Reference Muttitt and Kartha2020). Third, long-standing political opposition from fossil fuel interests and incumbents continues to undermine and delay mitigation efforts across all sectors (Steckel & Jakob, Reference Steckel and Jakob2022; Stoddard et al., Reference Stoddard, Anderson, Capstick, Carton, Depledge, Facer, Gough, Hache, Hoolohan, Hultman, Hällström, Kartha, Klinsky, Kuchler, Lövbrand, Nasiritousi, Newell, Peters, Sokona and Williams2021).
Fourth, investors in fossil fuel assets covered by international investment treaties are increasingly using investor–state dispute settlement to protect expected profits, with governments potentially exposed to up to 340 billion USD in liabilities from potential legal claims in the oil and gas sector alone (Tienhaara et al., Reference Tienhaara, Thrasher, Simmons and Gallagher2022). Finally, while diverse policy options exist for implementing a managed and equitable wind-down of fossil fuels (Diluiso et al., Reference Diluiso, Walk, Manych, Cerutti, Chipiga, Workman, Ayas, Cui, Cui, Song, Banisch, Moretti, Callaghan, Clarke, Creutzig, Hilaire, Jotzo, Kalkuhl, Lamb and Minx2021), sociopolitical contexts can affect their feasibility and effectiveness (Steckel & Jakob, Reference Steckel and Jakob2022).
Nevertheless, in recent years, climate policies and actions are increasingly targeting fossil fuel phase-out. Notable inter-governmental efforts include the ‘Powering Past Coal Alliance’, aimed at phasing-out coal-fired power by 2030–2040, and the ‘Beyond Oil and Gas Alliance’, focused on phasing-out oil and gas production. Many governments are also exploring legal and diplomatic means of limiting exposure to potential investor–state disputes of fossil fuel infrastructure, such as by leaving the Energy Charter Treaty. Many national-level efforts are addressing gasoline vehicles and fossil-gas heating systems in buildings (Kerr & Winskel, Reference Kerr and Winskel2022). In sectors lacking viable alternatives (steel, cement, aviation, shipping), there is less phase-out research and policy (Trencher et al., Reference Trencher, Rinscheid, Rosenbloom and Truong2022). Efforts to reduce fossil fuel consumption in military operations, which are a major source of publicly financed emissions globally, also lag (Stoddard et al., Reference Stoddard, Anderson, Capstick, Carton, Depledge, Facer, Gough, Hache, Hoolohan, Hultman, Hällström, Kartha, Klinsky, Kuchler, Lövbrand, Nasiritousi, Newell, Peters, Sokona and Williams2021).
Researchers are increasingly focused in understanding how fast phase-outs can and should be achieved in different countries, taking into account differentiated capacities and circumstances (Calverley & Anderson, Reference Calverley and Anderson2022; Fyson et al., Reference Fyson, Ganti, Grant and Hare2022). The relative phase-out pace of the three fossil fuels matters too: while cost-optimized models vary in their pace of phase-out (Achakulwisut et al., Reference Achakulwisut, Erickson, Guivarch, Schaeffer, Brutschin and Pye2023) a common feature is that they generally phase-out coal much more rapidly. However, this can put an unrealistic burden on coal-dependent developing countries, suggesting more attention is needed on oil and gas phase-out (Muttitt et al., Reference Muttitt, Price, Pye and Welsby2023). There is also a growing literature on what a multilateral response to phasing out fossil fuels globally could look like, including models for international cooperation and the role of climate litigation and social movements (van Asselt & Newell, Reference van Asselt and Newell2022). On the financial side, studies have mapped out the influence of specific actors in perpetuating fossil fuel lock-in, including asset managers and governments (Baines & Hager, Reference Baines and Hager2023; Dordi et al., Reference Dordi, Gehricke, Naef and Weber2022), top wealth owners (Semieniuk et al., Reference Semieniuk, Chancel, Saïsset, Holden, Mercure and Edwards2023), and banks (Rainforest Action Network et al., 2023).
In sum, building new fossil fuel infrastructure carries significant economic, financial, legal, and climate risks. Delaying action would not only necessitate a faster and costlier decarbonization later on, but also heighten socioeconomic disruptions (Skjølsvold & Coenen, Reference Skjølsvold and Coenen2021). Strategies to phase-out fossil fuels should also be paired with actions to accelerate the uptake of clean alternatives to avoid energy shortages, price spikes, and inflation, and to create employment opportunities for workers transitioning from fossil fuel industries (Grubert & Hastings-Simon, Reference Grubert and Hastings-Simon2022; Heffron & McCauley, Reference Heffron and McCauley2022). Phase-out and phase-in are synergistic: evidence from past experiences shows that phase-out policies can drive innovation and the scale-up of alternatives (Diluiso et al., Reference Diluiso, Walk, Manych, Cerutti, Chipiga, Workman, Ayas, Cui, Cui, Song, Banisch, Moretti, Callaghan, Clarke, Creutzig, Hilaire, Jotzo, Kalkuhl, Lamb and Minx2021; Trencher et al., Reference Trencher, Rinscheid, Florentine, Truong and Temocin2023). Governments and financial institutions should plan a managed and internationally coordinated, and equitable phase-out, beginning now.
3.3 Insight 3. Carbon dioxide removal is necessary but faces challenges in up-scaling, accounting, and governance
Meeting the Paris Agreement will require rapidly reducing emissions while also scaling up carbon dioxide removal (CDR) (IPCC, 2022b, Ch12.3). CDR involves capturing atmospheric CO2 and durably storing it. Scenarios that keep warming well below 2°C include removing hundreds of billions of tons of CO2 from the atmosphere over the course of the century to compensate for both residual emissions and potential overshoot (IPCC, 2022b, Ch12.3). Currently, nearly all CDR consists of afforestation/reforestation (A/R) and forestry. By contrast, only 0.1% of current removals come from more ‘novel CDR’ methods which partly go beyond the land use, land-use change, and forestry (LULUCF) sector. These more novel methods comprise in particular bioenergy with carbon capture and storage (BECCS) and biochar, with an even smaller contribution from other novel methods (e.g. direct air carbon capture and storage [DACCS], enhanced weathering, and ocean CDR approaches such as alkalinity enhancement or macroalgae sinking) (Powis et al., Reference Powis, Smith, Minx and Gasser2023). However, novel CDR methods have large technical removal potential (Smith et al., Reference Smith, Geden, Nemet, Gidden, Lamb, Powis, Bellamy, Callaghan, Cowie, Cox, Fuss, Gasser, Grassi, Greene, Lück, Mohan, Müller-Hansen, Peters, Pratama and Minx2023) and are scaled up in virtually all scenarios that limit warming to 1.5°C or 2°C (Fuss et al., Reference Fuss, Lamb, Callaghan, Hilaire, Creutzig, Amann, Beringer, de Garcia, Hartmann, Khanna, Luderer, Nemet, Rogelj, Smith, Vicente, Wilcox, del Dominguez and Minx2018; IPCC, 2022b, Ch12.3).
A ‘CDR gap’ exists between the extent of CDR deployment in countries' plans and what mitigation scenarios indicate would be needed to meet the Paris Agreement temperature limit. The median yearly gap for 2050 across scenarios is >1 Gt CO2 at a minimum and >7 Gt CO2 with less ambitious emission reductions (for comparison, the total current CDR capacity is 2 Gt/year) (Smith et al., Reference Smith, Geden, Nemet, Gidden, Lamb, Powis, Bellamy, Callaghan, Cowie, Cox, Fuss, Gasser, Grassi, Greene, Lück, Mohan, Müller-Hansen, Peters, Pratama and Minx2023). Closing the CDR gap requires lengthy times (one–three decades) for developing technology, designing effective monitoring, reporting, and verification (MRV), ensuring ecosystem safety, building supporting infrastructure, and scaling up deployment. Therefore, the extent of early deployment of novel CDR over the next decade is likely to be consequential in determining whether CDR will be available at scale and in time to reach net-zero CO2 emissions by the early 2050s, as well as whether it will be available for net-negative CO2 emissions afterward.
CDR options include a wide variety of approaches, levels of technological readiness, and durability of sequestration (Figure 3) (Fuhrman et al., Reference Fuhrman, Bergero, Weber, Monteith, Wang, Clarens, Doney, Shobe and McJeon2023). All CDR options have remaining scientific uncertainties around MRV and life-cycle assessment boundaries that need to be addressed (Mercer & Burke, Reference Mercer and Burke2023) (see Box 3). For example, while measuring the capture and sequestration from DACCS is straightforward, its high-energy intensity risks diverting clean energy that could otherwise be used for grid decarbonization (Sovacool et al., Reference Sovacool, Baum and Low2023). Estimates of CO2 fluxes in the LULUCF sector suffer from high levels of uncertainty in general and are hampered by confounding effects from environmental changes and inconsistent definitions (Pongratz et al., Reference Pongratz, Schwingshackl, Bultan, Obermeier, Havermann and Guo2021).
Box 3. Mix of CDR options deployed and included in scenarios will evolve
Currently, the vast majority of CDR happens through methods that fall in the LULUCF sector (such as afforestation/reforestation (A/R); improved forest management, and long-lived product usage; agroforestry; soil carbon in croplands and grasslands). All methods imply trade-offs but may offer co-benefits, for example, local climate or biodiversity, if implemented carefully. But the impermanence of the carbon storage in LULUCF options is of particular concern, as forests in many regions are increasingly threatened by climate driven disturbances (droughts, heatwaves, fires, storms, pests). ‘Novel’ CDR options, like bioenergy with carbon capture and storage (BECCS), direct air carbon capture and storage (DACCS), biochar and ocean alkalinization currently play only a minor role, and come with their own challenges, for example, cost, energy demand, and unintended ecological side-effects. Scenarios evaluated by the IPCC that stay below a 2°C warming (2022b, Ch3) typically assign a large relevance to LULUCF CDR and to BECCS. Many of these scenarios assume BECCS scales up substantially by the end of the century, some also assume a large scale-up of DACCS in the second half of the century. The central role of these three CDR options reflects the current capabilities of the underlying socioeconomic models, rather than a judgment of the feasibility of CDR options. But many research and demonstration programs, as well as policy strategies, consider a broader range of CDR options. And given the limited potential of each method and associated risks at scale, it may be preferable to complement emission reduction efforts with a portfolio of CDR options, which adjusts over time to account for technological progress and changing environmental, societal, economic, and political requirements.

Figure 3. Taxonomy of CDR options. The ‘CDR method’ (first row of the figure), featuring the most widely discussed in recent literature, ‘Time scale of carbon storage’ (second row) refers to the expected durability of the carbon storage, ‘Current readiness to scale’ (third row) refers to the maturity level for deployment at scale, and ‘Biophysical or technical sequestration potential’ (fourth row) reflects current understanding (based largely on IPCC, 2022b, Ch12.3), additional references are given in the Supplementary material, SM7. Modified from IPCC (2022b, Ch12.3).
Similarly, there are large uncertainties (as well as practical limitations to measurement) in the weathering rates of silicate rocks when applied to fields (Buckingham et al., Reference Buckingham, Henderson, Holdship and Renforth2022) and air–sea gas exchange dynamics for direct ocean removal or ocean alkalinity enhancement (Bach et al., Reference Bach, Ho, Boyd and Tyka2023). There are also unclear risks of runaway secondary precipitation associated with ocean alkalinity enhancement (Hartmann et al., Reference Hartmann, Suitner, Lim, Schneider, Marín-Samper, Arístegui, Renforth, Taucher and Riebesell2023), and counterfactual carbon storage uncertainties associated with biomass-based CDR (Hausfather et al., Reference Hausfather, Klitzke and Chay2022). When CDR is used to claim that a ton of fossil CO2 emissions is effectively undone, a mismatch in timeframes may still result in long-term climate effects (Allen et al., Reference Allen, Friedlingstein, Girardin, Jenkins, Malhi, Mitchell-Larson, Peters and Rajamani2022). All these can undermine our ability to meet the Paris Agreement temperature goal, which is a function of cumulative CO2 emissions and can only be achieved if CO2 emissions reach net-zero.
The durability of using A/R or soil carbon is at risk in a warming world because of the increased prevalence of wildfires, droughts, and pests (Anderegg et al., Reference Anderegg, Wu, Acil, Carvalhais, Pugh, Sadler and Seidl2022); analogous to effects in natural carbon sinks (see insight 4). Vegetation regrowth in the absence of anthropogenic A/R interventions is also largely unaccounted for (Jayakrishnan et al., Reference Jayakrishnan, Bala, Cao and Caldeira2022). For this reason both the scientific community (Allen et al., Reference Allen, Friedlingstein, Girardin, Jenkins, Malhi, Mitchell-Larson, Peters and Rajamani2022) and standard-setting bodies (e.g. SBTi, 2020) are increasingly emphasizing a ‘like-for-like’ approach to CDR neutralization claims, where fossil CO2 emissions should be neutralized through CDR that durably sequesters CO2, while LULUCF CDR can only be used to neutralize land-use-related CO2 emissions (Allen et al., Reference Allen, Friedlingstein, Girardin, Jenkins, Malhi, Mitchell-Larson, Peters and Rajamani2022). Propositions for how to account for non-permanence have emerged in frameworks for quantifying the climate benefit (Prado & Mac Dowell, Reference Prado and Mac Dowell2023) and the design of policy architectures (Edenhofer et al., Reference Edenhofer, Franks, Kalkuhl and Runge-Metzger2023). One practical example is a European Commission proposal for temporary CDR credits for less permanent options (European Commission, 2022c).
With the widespread adoption of net-zero emission targets, countries have begun to integrate CDR into modeled national mitigation pathways, increase research, development, and demonstration (RD&D) efforts on CDR methods, and consider CDR-specific incentives and policies (IPCC 2022b, Ch12.3; Smith et al., Reference Smith, Geden, Nemet, Gidden, Lamb, Powis, Bellamy, Callaghan, Cowie, Cox, Fuss, Gasser, Grassi, Greene, Lück, Mohan, Müller-Hansen, Peters, Pratama and Minx2023). CDR policymaking is faced with the need to consider the method-specific timescales of CO2 storage, and challenges in MRV and accounting described above, as well as potential co-benefits, adverse side effects, interactions with adaptation and trade-offs with the sustainable development goals (SDGs) (IPCC, 2022b, Ch3.7, Ch12.3). Therefore, CDR governance and policymaking are expected to focus on responsibly incentivizing RD&D and targeted deployment, building on the technical and governance experience gained from already widely practiced CDR methods like A/R, as well as learning from two decades of the slow-moving carbon capture and storage (CCS) deployment. For novel CDR, such as ocean alkalinization or enhanced weathering, investment in RD&D would help in understanding the risks, rewards, and uncertainties of deployment (Smith et al., Reference Smith, Geden, Nemet, Gidden, Lamb, Powis, Bellamy, Callaghan, Cowie, Cox, Fuss, Gasser, Grassi, Greene, Lück, Mohan, Müller-Hansen, Peters, Pratama and Minx2023).
Some aspects of CDR governance and policy instruments will be similar to those around emission reduction measures, while others will require governance innovation. Effectively integrating CDR into governments' mitigation portfolios, to close the ‘CDR gap’, can build on already existing rules, procedures, and instruments for emission abatement (Edenhofer et al., Reference Edenhofer, Franks, Kalkuhl and Runge-Metzger2023; Michaelowa et al., Reference Michaelowa, Honegger, Poralla, Winkler, Dalfiume and Nayak2023). A political commitment to formal integration into existing climate policy frameworks is required (Sovacool et al., Reference Sovacool, Baum and Low2023), such as robust MRV systems (Mercer & Burke, Reference Mercer and Burke2023). To avoid CDR being misperceived as a substitute for deep emission reductions (Buck et al., Reference Buck, Carton, Lund and Markusson2023), the prioritization of emission cuts can be signaled and achieved by explicitly setting separate targets for reductions and removals (Carton et al., Reference Carton, Hougaard, Markusson and Lund2023), for example. Similarly, sub-targets are conceivable for different types of CDR, to prioritize preferred methods according to characteristics such as removal processes or timescales of storage (Allen et al., Reference Allen, Friedlingstein, Girardin, Jenkins, Malhi, Mitchell-Larson, Peters and Rajamani2022).
3.4 Insight 4. New reasons for concern: the uncertain future contribution of land and ocean carbon sinks
The remaining carbon budget (RCB), for staying within a given temperature limit, shrinks with every ton of emitted CO2, and the RCB for staying below 1.5°C of global warming with a chance of 50% will soon be exhausted (see insight 1 on overshoot). The magnitude of the RCB substantially depends on assumptions about the future contribution of the natural carbon sinks on land and in the ocean. In IPCC's carbon budgets for the 21st century (2021, Ch5.5), the sinks are assumed to respond in a relatively linear manner to changes in temperature, CO2, and other forcings, yet recent scientific insights cast doubts on our understanding and knowledge of their future trajectory (Figure 4).

Figure 4. Future carbon sinks and the remaining carbon budget (RCB): for any given temperature limit, the RCB (cumulative net global anthropogenic for a given global warming limit. CO2 emissions, expressed from a recent specified date) is constructed to balance expectations on the future capacities of natural carbon sinks (among other variables). If sinks are smaller than expected (and skewed uncertainties are currently pointing in that direction, especially for land), there will be even more warming than expected (unless the RCB is adjusted and action taken accordingly to stay within the adjusted budget).
In spite of rising emissions, a relatively constant fraction of only about 44% has remained in the atmosphere over the past 50 years (IPCC, 2021, Ch5), because the sinks on land and in the ocean have become stronger in line with increases in atmospheric CO2. However, recent data suggest that the rate of increase in the strength of the land sink may have slowed down (Chandra et al., Reference Chandra, Patra, Niwa, Ito, Iida, Goto, Morimoto, Kondo, Takigawa, Hajima and Watanabe2022; Friedlingstein et al., Reference Friedlingstein, O'Sullivan, Jones, Andrew, Gregor, Hauck, Le Quéré, Luijkx, Olsen, Peters, Peters, Pongratz, Schwingshackl, Sitch, Canadell, Ciais, Jackson, Alin, Alkama and Zheng2022). This could be the result of natural variability of the land sink, or potentially an indication of reduced capacity of terrestrial ecosystems to take up and store CO2. The latter would be related to negative effects of climate change including the associated increase in temperature (Fernández-Martínez et al., Reference Fernández-Martínez, Peñuelas, Chevallier, Ciais, Obersteiner, Rödenbeck, Sardans, Vicca, Yang, Sitch, Friedlingstein, Arora, Goll, Jain, Lombardozzi, McGuire and Janssens2023), changes in rainfall patterns and weather extremes like concurrent hot–dry conditions (Tschumi et al., Reference Tschumi, Lienert, Bastos, Ciais, Gregor, Joos, Knauer, Papastefanou, Rammig, van der Wiel, Williams, Xu, Zaehle and Zscheischler2023), and a general risk of destabilization of the sinks owing to multiple human disturbances (Fernández-Martínez et al., Reference Fernández-Martínez, Peñuelas, Chevallier, Ciais, Obersteiner, Rödenbeck, Sardans, Vicca, Yang, Sitch, Friedlingstein, Arora, Goll, Jain, Lombardozzi, McGuire and Janssens2023). Even though additional human factors like land-use change and landscape fragmentation, as well as model limitations, make these interpretations highly uncertain (Rosan et al., Reference Rosan, Sitch, Mercado, Heinrich, Friedlingstein and Aragão2022), there is sparse but strong evidence that the land sinks are changing more rapidly than expected: for the tropics, concerns have been raised already in 2020 that observed carbon uptake may have peaked (Hubau et al., Reference Hubau, Lewis, Phillips, Affum-Baffoe, Beeckman, Cuní-Sanchez, Daniels, Ewango, Fauset, Mukinzi, Sheil, Sonké, Sullivan, Sunderland, Taedoumg, Thomas, White, Abernethy, Adu-Bredu and Zemagho2020) or even shifted to a carbon source (Gatti et al., Reference Gatti, Basso, Miller, Gloor, Gatti Domingues, Cassol, Tejada, Aragão, Nobre, Peters, Marani, Arai, Sanches, Corrêa, Anderson, Von Randow, Correia, Crispim and Neves2021), and most models do not reproduce the observed strengthening in the coupling between water availability and the terrestrial carbon cycle (Liu et al., Reference Liu, Ciais, Wu, Padrón, Friedlingstein, Schwaab, Gudmundsson and Seneviratne2023a). Additionally, forest degradation triggered mainly by drought and increased vapor pressure deficit, is usually not well represented in ecosystem models, but may account for as much carbon emissions as deforestation (Lapola et al., Reference Lapola, Pinho, Barlow, Aragão, Berenguer, Carmenta, Liddy, Seixas, Silva, Silva-Junior, Alencar, Anderson, Armenteras, Brovkin, Calders, Chambers, Chini, Costa, Faria and Walker2023). Boreal forests are also under stress: Liu et al. (Reference Liu, Peng, Schneider, Cyr, McDowell and Kneeshaw2023b) report a drought-induced increase in tree mortality and a corresponding decrease in the carbon sink capacity of Canadian boreal forests (which represent a third of all boreal forests worldwide) over the past 50 years. Unexpected events of elevated tree mortality are observed across the world, but due to a lack of data and understanding it is not yet clear whether this represents a global trend toward increasing tree mortality (Hartmann et al., Reference Hartmann, Bastos, Das, Esquivel-Muelbert, Hammond, Martínez-Vilalta, McDowell, Powers, Pugh, Ruthrof and Allen2022).
The ocean sink strength stalled in the 1990s, primarily driven by wind changes in the Southern Ocean, and has recovered since (DeVries et al., Reference DeVries, Yamamoto, Wanninkhof, Gruber, Hauck, Müller, Bopp, Carroll, Carter, Chau, Doney, Gehlen, Gloege, Gregor, Henson, Kim, Iida, Ilyina, Landschützer and Zeng2023; Gruber et al., Reference Gruber, Bakker, DeVries, Gregor, Hauck, Landschützer, McKinley and Müller2023). While the main contribution for this increase in the uptake due to rising atmospheric CO2 concentrations (Müller et al., Reference Müller, Gruber, Carter, Feely, Ishii, Lange, Lauvset, Murata, Olsen, Pérez, Sabine, Tanhua, Wanninkhof and Zhu2023), but climate change acting on the large natural carbon reservoir in the ocean seems to be having effects as well (Crisp et al., Reference Crisp, Dolman, Tanhua, McKinley, Hauck, Bastos, Sitch, Eggleston and Aich2022; Gruber et al., Reference Gruber, Bakker, DeVries, Gregor, Hauck, Landschützer, McKinley and Müller2023). For example, ocean warming may reduce CO2 uptake (Mignot et al., Reference Mignot, von Schuckmann, Landschützer, Gasparin, van Gennip, Perruche, Lamouroux and Amm2022) and lead to a substantial shift of natural carbon flux from the surface ocean to deeper ocean layers, via a feedback between biology and circulation (Keppler et al., Reference Keppler, Landschützer, Lauvset and Gruber2023). The imprints of climate change are strongest in the polar regions. In the Southern Ocean, wind changes continue to expose more carbon-rich deep waters to the air–sea interface and thus to a loss of natural carbon (Hauck et al., Reference Hauck, Gregor, Nissen, Patara, Hague, Mongwe, Bushinsky, Doney, Gruber, Quéré, Manizza, Mazloff, Monteiro and Terhaar2023). The Arctic Ocean stands out as the only region where climate change increases the carbon sink strength with sea-ice retreat leading to larger ocean surface areas where CO2 uptake happens (Yasunaka et al., Reference Yasunaka, Manizza, Terhaar, Olsen, Yamaguchi, Landschützer, Watanabe, Carroll, Adiwara, Müller and Hauck2023).
Still, large uncertainties remain about the trends in the ocean carbon sink and on the confounding effects of changes in land-use and management, and the impact of extreme events and disturbances. While observational records span only a few decades, trends are influenced by slow modes of natural climate variability (Li et al., Reference Li, Sippel, Winkler, Mahecha, Reichstein and Bastos2022b), superimposed on longer-term effects such as climate change, elevated CO2, and others. Additionally, the effects of climate change on internal ecological processes, which are currently poorly constrained and represented in models, may play a much bigger role in the future. This is expected to become especially important after peak emissions and under CO2 removal scenarios, when sinks will no longer be influenced by the current near-exponential increase in atmospheric CO2 and rather start outgassing some of the accumulated carbon (Keller et al., Reference Keller, Lenton, Littleton, Oschlies, Scott and Vaughan2018; Zickfield et al., Reference Zickfield, Azevedo, Mathesius and Matthews2021). Therefore, disentangling the impacts of anthropogenic activity from natural climate variability on the carbon cycle is crucial (Bastos et al., Reference Bastos, Ciais, Sitch, Aragão, Chevallier, Fawcett, Rosan, Saunois, Günther, Perugini, Robert, Deng, Pongratz, Ganzenmüller, Fuchs, Winkler, Zaehle and Albergel2022; Friedlingstein et al., Reference Friedlingstein, O'Sullivan, Jones, Andrew, Gregor, Hauck, Le Quéré, Luijkx, Olsen, Peters, Peters, Pongratz, Schwingshackl, Sitch, Canadell, Ciais, Jackson, Alin, Alkama and Zheng2022; Gruber et al., Reference Gruber, Bakker, DeVries, Gregor, Hauck, Landschützer, McKinley and Müller2023).
Overall, these remaining uncertainties, including some recently discovered ones, give rise to new reasons for concern about the future of the global natural carbon sink, with implications for the reliability of nature-based solutions (NbS) (Box 4) and nature-based CDR (see insight 3).
Box 4. ‘Reality check’ on nature-based solutions
The future of the natural sinks is decisive for a realistic understanding of the potential of nature-based solutions (NbS) in the coming decades, as natural sinks are the basis for most anthropogenic activities directed at carbon dioxide removal (see insight 3 on CDR). Therefore, by building on NbS, scenarios for climate mitigation policies rely heavily on current expectations for natural carbon sinks. In this box, we highlight some examples:
An increase in extreme events would change established disturbance regimes both on land and in the ocean (see insight 6 on compound events), with the risk of reducing the carbon storage potential. On land, a good example is fire, as it is a key driver of change among all types of disturbances that will increase in the future (Canadell et al., Reference Canadell, Meyer, Cook, Dowdy, Briggs, Knauer, Pepler and Haverd2021; Jones et al., Reference Jones, Abatzoglou, Veraverbeke, Andela, Lasslop, Forkel, Smith, Burton, Betts, van der Werf, Sitch, Canadell, Santín, Kolden, Doerr and Le Quéré2022; Zheng et al., Reference Zheng, Ciais, Chevallier, Yang, Canadell, Chen, van der Velde, Aben, Chuvieco, Davis, Deeter, Hong, Kong, Li, Li, Lin, He and Zhang2023). In the ocean, there are large uncertainties in carbon accounting due to the flows between coastal ecosystems, the shelves, and beyond. Additionally, the rapid increase of recent past and future marine heatwaves and extremes in ocean oxygen loss and acidification makes these ecosystems particularly vulnerable to climate change (Gattuso et al., Reference Gattuso, Williamson, Duarte and Magnan2021; Williamson & Gattuso, Reference Williamson and Gattuso2022) and increases uncertainties even further. This casts doubt on the potential of coastal ecosystems restoration (blue carbon) and other marine-based CDR methodologies.
Forests play a key role in the nationally determined contributions (NDCs) by many countries (European Commission, 2022a, 2022b). In total, 54% of parties refer to forest conservation, reforestation, or afforestation as a domestic opportunity (UNFCCC, 2022a). Even though there is certainly a role for this type of mitigation, limits to the natural basis exist that have not always been sufficiently accounted for in earlier estimates. For example, a recent study by Roebroek et al. (Reference Roebroek, Duveiller, Seneviratne, Davin and Cescatti2023) has shown that when accounting for natural disturbances at present-day levels, all types of forest management would result in only low mitigation. Rohatyn et al. (Reference Rohatyn, Yakir, Rotenberg and Carmel2022) find that carbon benefits of forestation of the vast global drylands would be largely counteracted by albedo effects.
In sum, given these concerns, scientists and policymakers need to be alert on a potential problem: assessments of mitigation requirements for the Paris Agreement rely on current model projections of the sink capacity (IPCC, 2021, Ch5.5). If these projections were to overestimate the potential future sink size the RCB would be smaller than it has been estimated (see Figure 4; such overestimation is likely, because model evaluation over the historical period has not recognised the need to adjust assumptions to new climatic conditions, as well as include other relevant processes). This has significant implications for policymaking toward net-zero emission goals. In order to reduce the uncertainties and avoid possible over- reliance on the benefits of natural carbon sinks, reliable quantifications of the sinks with reduced, but known, uncertainty are needed, particularly in forecasting. Thus, a fit-for-purpose and sustainably funded and managed ocean and land carbon observation system is crucially needed (Crisp et al., Reference Crisp, Dolman, Tanhua, McKinley, Hauck, Bastos, Sitch, Eggleston and Aich2022; Hartmann et al., Reference Hartmann, Bastos, Das, Esquivel-Muelbert, Hammond, Martínez-Vilalta, McDowell, Powers, Pugh, Ruthrof and Allen2022).
We acknowledge the importance of NbS as negative emission strategies, but a clear vulnerability assessment is required to make them effective and a permanent solution. It is essential that plans of carbon sequestration with NbS (of shorter storage durability, see insight 3) are not used to justify further delays on urgently needed emission reductions.
3.5 Insight 5. The climate and biodiversity emergencies and their solutions are intimately linked
There is now ample scientific evidence that the climate and biodiversity crises are closely intertwined, and yet continuet to be addressed by separate political, economic, social and legal institutions, and different actors. In 2019–2021, for the first time, the Intergovernmental Science-Policy Platform on Biodiversity and Ecosystem Services (IPBES) and the Intergovernmental Panel on Climate Change (IPCC) together gathered international experts in a joint report on the co-benefits and trade-offs of climate and biodiversity actions (Pörtner et al., Reference Pörtner, Scholes, Agard, Archer, Arneth, Bai, Barnes, Burrows, Chan, Cheung, Diamond, Donatti, Duarte, Eisenhauer, Foden, Gasalla, Hanada, Hickler, Hoegh-Guldberg and Ngo2021). ‘Nexus interactions’ are increasingly being studied (Estoque, Reference Estoque2023), and reflected under the policy umbrella of the SDGs (Martín et al., Reference Martín, Giordano, Pagano, Van Der Keur and Costa2020), which are key instruments to support decision-making and trigger synergistic and effective actions.
The major global crises of both biodiversity loss and climate change result from the dominant economic development and sociopolitical systems in modern societies (Dasgupta, Reference Dasgupta2021). These drivers manifest in a range of proximate pressures, some of which impact both climate and biodiversity, such as deforestation and intensive agriculture. Their expression locally varies with myriad contextual factors, and interact with other pressures acting at varied scales. Both drivers and pressures are governed and entrenched through institutional factors such as economic regulations, legislation, and financial and tax systems, that have promoted and incentivized environmentally damaging production and consumption models (Pörtner et al., Reference Pörtner, Scholes, Agard, Archer, Arneth, Bai, Barnes, Burrows, Chan, Cheung, Diamond, Donatti, Duarte, Eisenhauer, Foden, Gasalla, Hanada, Hickler, Hoegh-Guldberg and Ngo2021). Reform is needed to alleviate both crises.
Climate change has far-reaching impacts, affecting biological processes from the smallest intracellular level to entire ecosystems. These effects across multiple levels may amplify predicted impacts at a single level, triggering a rapid and unexpected shift of ecosystem and species across equatorial to polar, and the terrestrial and aquatic realms (Arneth et al., Reference Arneth, Shin, Leadley, Rondinini, Bukvareva, Kolb, Midgley, Oberdorff, Palomo and Saito2020). When modeling climate impacts, large-scale analyses tend to show smoothed aggregate responses, while models developed for individual species can exhibit abrupt responses to changing climate conditions, often experiencing half of their impact within just a decade (Pigot et al., Reference Pigot, Merow, Wilson and Trisos2023). Addressing multiple processes and interactions is as important as addressing multiple scales. To obtain a more accurate understanding of the biodiversity losses resulting from climate change, incorporating species interactions and potential co-extinction cascades into climate–biodiversity models is also needed (Moullec et al., Reference Moullec, Barrier, Drira, Guilhaumon, Hattab, Peck and Shin2022). Furthermore, feedbacks caused by climate-induced changes in species' physiology and shifts in functional diversity can trigger changes in marine and terrestrial carbon uptake and losses, potentially amplifying the initial CO2 forcing in many climate change scenarios (Arneth et al., Reference Arneth, Shin, Leadley, Rondinini, Bukvareva, Kolb, Midgley, Oberdorff, Palomo and Saito2020). In coastal marine ecosystems, climate migration and complex ecosystem transitions compounded by local pressures (overfishing, increasing turbidity of coastal waters, increasing toxicity of metal pollution) are anticipated to strongly impact coastal marine ecosystems, particularly in tropical regions (Herbert-Read et al., Reference Herbert-Read, Thornton, Amon, Birchenough, Côté, Dias, Godley, Keith, McKinley, Peck, Calado, Defeo, Degraer, Johnston, Kaartokallio, Macreadie, Metaxas, Muthumbi, Obura and Sutherland2022) where social and political vulnerability is already high.
Climate impacts on society mediated by biodiversity occur through shifts in nature's contributions to people (NCP). For example, pollinator diversity is strongly affected by fluctuations in winter weather, changes in the length of the vegetational season, and increased frequency of extreme weather events (Vasiliev & Greenwood, Reference Vasiliev and Greenwood2021), and con influence food production and hence human health (Smith et al., Reference Smith, Mueller, Springmann, Sulser, Garibaldi, Gerber, Wiebe and Myers2022). Complex climate–biodiversity–NCP feedback loops are increasingly being shown (Pörtner et al., Reference Pörtner, Scholes, Agard, Archer, Arneth, Bai, Barnes, Burrows, Chan, Cheung, Diamond, Donatti, Duarte, Eisenhauer, Foden, Gasalla, Hanada, Hickler, Hoegh-Guldberg and Ngo2021). For example, coastal ecosystems such as marshes and mangroves mitigate climate change by sequestering carbon while also reducing the impact of coastal storms on people, but they are vulnerable to sea-level rise, flooding from inland rainfall, and warming, thus compromising the benefits they provide (Temmerman et al., Reference Temmerman, Horstman, Krauss, Mullarney, Pelckmans and Schoutens2023).
NbS and ‘multifunctional-scape’ approaches can provide not only precautionary but also regenerative options for protecting biodiversity, mitigating climate change, and reinforcing the adaptation of nature and society to a wide spectrum of impacts if they are implemented appropriately (Pörtner et al., Reference Pörtner, Scholes, Agard, Archer, Arneth, Bai, Barnes, Burrows, Chan, Cheung, Diamond, Donatti, Duarte, Eisenhauer, Foden, Gasalla, Hanada, Hickler, Hoegh-Guldberg and Ngo2021). However, caution is needed, as demonstrated by the hasty implementation of large-scale tree planting to maximize carbon sequestration, resulting in missed synergistic opportunities and harm to other aspects of nature, the provisioning of benefits to people as well as to broader human rights (Seddon et al., Reference Seddon, Smith, Smith, Key, Chausson, Girardin, House, Srivastava and Turner2021). Safeguards and guidance for well-designed NbS that deliver multiple benefits for people and nature are required (Seddon et al., Reference Seddon, Smith, Smith, Key, Chausson, Girardin, House, Srivastava and Turner2021; Shin et al., Reference Shin, Midgley, Archer, Arneth, Barnes, Chan, Hashimoto, Hoegh-Guldberg, Insarov, Leadley, Levin, Ngo, Pandit, Pires, Pörtner, Rogers, Scholes, Settele and Smith2022), using ‘ecosystem-based approaches’. For example, synergies and trade-offs between biodiversity protection, climate mitigation, and food production show that moderate ambition across all targets may achieve balance, but high ambition for just one results in lower achievement of others (Arneth et al., Reference Arneth, Leadley, Claudet, Coll, Rondinini, Rounsevell, Shin, Alexander and Fuchs2023).
Understanding and managing the interlinked impacts of climate and biodiversity change on society remains extremely challenging. Available data and dominant approaches for biodiversity conservation are still strongly biased to the Global North (Isbell et al., Reference Isbell, Balvanera, Mori, He, Bullock, Regmi, Seabloom, Ferrier, Sala, Guerrero-Ramírez, Tavella, Larkin, Schmid, Outhwaite, Pramual, Borer, Loreau, Omotoriogun, Obura and Palmer2023), as are capacities for producing and using climate information. While there is significant progress in scientific understanding of Global South ecosystems (e.g. grassland ecosystems, Stevens et al., Reference Stevens, Bond, Feurdean and Lehmann2022), much evidence coming from researchers of Global South still does not find its way to global decision-making (e.g. Armani et al., Reference Armani, Asefa, Zakari, Agyekum, Archibald, Pereira and Coetzer2022). Even so, our ability to model and anticipate risks and shifts induced by biodiversity–climate changes is insufficient to incorporate them into policy responses (Marske et al., Reference Marske, Lanier, Siler, Rowe and Stein2023) and their complexity challenges implementation. The disproportionate impacts of climate–biodiversity interactions in tropical regions in both terrestrial and coastal marine zones (Arneth et al., Reference Arneth, Shin, Leadley, Rondinini, Bukvareva, Kolb, Midgley, Oberdorff, Palomo and Saito2020; Herbert-Read et al., Reference Herbert-Read, Thornton, Amon, Birchenough, Côté, Dias, Godley, Keith, McKinley, Peck, Calado, Defeo, Degraer, Johnston, Kaartokallio, Macreadie, Metaxas, Muthumbi, Obura and Sutherland2022) carry strong climate equity implications (see insight 9 on adaptation justice). Countries in these regions have contributed least to climate forcing yet face high potential for cascades and tipping dynamics, making a strong case for precautionary and transformative policies.
The intimate interlinkages between climate change mitigation and adaptation, biodiversity conservation actions, and broader societal needs will require transformative change in the governance of social–ecological systems at all scales (Pörtner et al., Reference Pörtner, Scholes, Agard, Archer, Arneth, Bai, Barnes, Burrows, Chan, Cheung, Diamond, Donatti, Duarte, Eisenhauer, Foden, Gasalla, Hanada, Hickler, Hoegh-Guldberg and Ngo2021), aiming at policy alignment:
(1) Policy must address the interactions between biodiversity loss and climate change mitigation and adaptation to trigger synergies, minimize trade-offs with other benefits (e.g. food production or soil regulation), and plan for performance under potential emission scenarios. NbS proposed for carbon sequestration, and their financing, must meet ecosystem-based and social justice criteria. Planning of conservation and climate actions must also consider the influence of harmful financial incentives, risks posed by complex interactions, and positive economic and social effects (Kedward et al., Reference Kedward, Ryan-Collins and Chenet2022).
(2) This alignment of actions may only be feasible through integrating work programs and decisions of the climate and biodiversity conventions, and their instruments (two-thirds of the post-2020 biodiversity actions of the CDR directly support climate goals) (Shin et al., Reference Shin, Midgley, Archer, Arneth, Barnes, Chan, Hashimoto, Hoegh-Guldberg, Insarov, Leadley, Levin, Ngo, Pandit, Pires, Pörtner, Rogers, Scholes, Settele and Smith2022) and other conventions under the framework of the SDGs (three-quarter of SDG targets are positively related to at least one NbS in a given ecosystem) (Mariani et al., Reference Mariani, Moullec, Atwood, Clarkson, Conant, Cullen-Unsworth, Griscom, Gutt, Howard, Krause-Jensen, Leavitt, Lee, Livesley, Macreadie, St-John, Zganjar, Cheung, Duarte, Shin and Mouillotin revision). This is needed to inform consultations on the post-2030 agenda.
(3) Reform-dominant economic and sociopolitical systems that drive climate change and biodiversity loss (Dasgupta, Reference Dasgupta2021); for example, through re-appraising indicators of economic and social development (European Parliament, 2023) and better addressing systemic biodiversity and climate-related risks (Kedward et al., Reference Kedward, Ryan-Collins and Chenet2022) (Box 5).
Box 5. Example: Coral reefs threatened by climate change and biodiversity loss
Coral reefs are among the first ecosystems being driven to collapse globally, by multiple interacting drivers. Of the 11 Western Indian Ocean ecoregions, four are ‘critically endangered’, three are ‘endangered’ and four are ‘vulnerable’ to collapse over a 50 year period (Figure 5; Obura et al., Reference Obura, Gudka, Samoilys, Osuka, Mbugua, Keith, Porter, Roche, Van Hooidonk, Ahamada, Araman, Karisa, Komakoma, Madi, Ravinia, Razafindrainibe, Yahya and Zivane2021). Biodiversity–Climate interactions underpin their risk of future collapse. Broadly, island reefs are at higher risk from increasing temperatures in the next three–four decades, while continental reefs have better climate futures, but higher impact from fishing and other local threats. The differential vulnerability of the ecoregions highlights the narrow gradient in vulnerability among reefs and that very small increments in global temperatures may make a difference between just some or all reef ecoregions crossing their point of collapse. The importance of coral reefs to coastal economies and livelihoods is illustrated in the differential vulnerability of ecoregions to fishing and temperature, and the importance of maximizing synergies among management actions to minimize both.

Figure 5. Ecoregions of the Western Indian Ocean showing their risk of collapse in the IUCN Red List of Ecosystems. Colors in ecoregions and circles show: least concern – dark green; near threatened – light green; vulnerable – yellow; endangered – orange; critically endangered – red; data deficient – gray. Risk levels for climate (thermometer icon) and biotic (coral and fish icons) ecosystem components are shown and their individual levels of risk. The combined biotic risk level is shown as well (coral/fish icon), and for each ecoregion by background shading and the map. The text highlights biodiversity–Climate interactions and prospects for management and benefits for people.
3.6 Insight 6. Compound events heighten climate risks in unexpected ways
Compound events are defined as events that occur when a combination of drivers and/or hazards contribute to environmental or societal risks (Zscheischler et al., Reference Zscheischler, Westra, van den Hurk, Seneviratne, Ward, Pitman, AghaKouchak, Bresch, Leonard, Wahl and Zhang2018) (Figure 6). These phenomena span a wide range of spatiotemporal scales and interaction types, including preconditioning, multiple variables, temporal compounding, and spatial compounding (Zscheischler et al., Reference Zscheischler, Martius, Westra, Bevacqua, Raymond, Horton, van den Hurk, AghaKouchak, Jézéquel, Mahecha, Maraun, Ramos, Ridder, Thiery and Vignotto2020). In the physical science domain, research on compound events was initially largely focused on the atmosphere and on bivariate events, such as drought–heat wave interactions.

Figure 6. Conceptual illustration of a compound event. The illustration shows how a cyclone followed on by a fire (a temporally compounded event) creates a much larger impact than either one on its own. On the bottom right is an idealized illustration of a two-dimensional distribution of the same two hazards and a potential impact that gets worse toward the upper right. Based on Ibanez et al. (Reference Ibanez, Platt, Bellingham, Vieilledent, Franklin, Martin, Menkes, Pérez-Salicrup, Russell-Smith and Keppel2022) and Zscheischler et al. (Reference Zscheischler, Martius, Westra, Bevacqua, Raymond, Horton, van den Hurk, AghaKouchak, Jézéquel, Mahecha, Maraun, Ramos, Ridder, Thiery and Vignotto2020).
Recently, substantial progress has been made in adapting the ‘compound event’ concept to a wider range of domains, including terrestrial ecosystems (Coughlan de Perez et al., Reference Coughlan de Perez, Ganapathi, Masukwedza, Griffin and Kelder2023; Lesk et al., Reference Lesk, Anderson, Rigden, Coast, Jägermeyr, McDermid, Davis and Konar2022; Vautard et al., Reference Vautard, van Oldenborgh, Bonnet, Li, Robin, Kew, Philip, Soubeyroux, Dubuisson, Viovy, Reichstein, Otto and Garcia de Cortazar-Atauri2023), the ocean (Burger et al., Reference Burger, Terhaar and Frölicher2022; Gruber et al., Reference Gruber, Boyd, Frölicher and Vogt2021; Le Grix et al., Reference Le Grix, Zscheischler, Rodgers, Yamaguchi and Frölicher2022), and inter-domain linkages (Pathmeswaran et al., Reference Pathmeswaran, Sen Gupta, Perkins-Kirkpatrick and Hart2022). New methodological approaches, such as the use of large ensembles (Burger et al., Reference Burger, Terhaar and Frölicher2022; Le Grix et al., Reference Le Grix, Zscheischler, Rodgers, Yamaguchi and Frölicher2022; Raymond et al., Reference Raymond, Suarez-Gutierrez, Kornhuber, Pascolini-Campbell, Sillmann and Waliser2022) and extreme event attribution (Zscheischler & Lehner, Reference Zscheischler and Lehner2022), have been developed and applied, demonstrating the relevance of compound events for a range of impacted domains. Recent literature shows how compound events pose critical risks for food security and ecosystem services over both land and ocean (Gruber et al., Reference Gruber, Boyd, Frölicher and Vogt2021; Yin et al., Reference Yin, Gentine, Slater, Gu, Pokhrel, Hanasaki, Guo, Xiong and Schlenker2023), make disaster risk management more challenging (Schlumberger et al., Reference Schlumberger, Haasnoot, Aerts and de Ruiter2022; van den Hurk et al., Reference van den Hurk, White, Ramos, Ward, Martius, Olbert, Roscoe, Goulart and Zscheischler2023), interfere with adaptation strategies (Simpson et al., Reference Simpson, Williams, Mach, Berrang-Ford, Biesbroek, Haasnoot, Segnon, Campbell, Musah-Surugu and Joe2023), and affect human migration patterns (Thalheimer et al., Reference Thalheimer, Choquette-Levy and Garip2022). Parallel multi-hazard work has been making strides in developing analysis and adaptation tools to better prepare societies for these systemic complexities (De Angeli et al., Reference De Angeli, Malamud, Rossi, Taylor, Trasforini and Rudari2022).
For agriculture and terrestrial ecosystems, compound events may be viewed as causing physiological stress directly, or as a combination of stressors that leads to an impact. Crops are particularly sensitive to the co-occurrence of extremely hot and dry conditions (Lesk et al., Reference Lesk, Anderson, Rigden, Coast, Jägermeyr, McDermid, Davis and Konar2022; Yin et al., Reference Yin, Gentine, Slater, Gu, Pokhrel, Hanasaki, Guo, Xiong and Schlenker2023). In a warming climate, impacts are expected to intensify in many regions of the world. Some compound impacts on crops are more closely linked to variability, such as an early spring followed by a late frost, an event type anticipated to increase in frequency (Vautard et al., Reference Vautard, van Oldenborgh, Bonnet, Li, Robin, Kew, Philip, Soubeyroux, Dubuisson, Viovy, Reichstein, Otto and Garcia de Cortazar-Atauri2023). Given that a large proportion of crops are grown in just a few breadbasket regions, if yields are impacted within the same harvest year in more than one region (i.e. spatially compounding events) there could be repercussions for global food security (Coughlan de Perez et al., Reference Coughlan de Perez, Ganapathi, Masukwedza, Griffin and Kelder2023; Gaupp et al., Reference Gaupp, Hall, Hochrainer-Stigler and Dadson2020; Raymond et al., Reference Raymond, Suarez-Gutierrez, Kornhuber, Pascolini-Campbell, Sillmann and Waliser2022). More generally, terrestrial ecosystems can be highly sensitive to compounding impact drivers. After severe compound hot–dry events, plant recovery usually lags due to reduced growth, irreversible losses in hydraulic conductance, or depletion of carbon reserves. Lagged growth may in turn increase vulnerability to another compound event if it occurs before complete recovery, potentially limiting vegetation's capacity to act as a carbon sink (Yin et al., Reference Yin, Gentine, Slater, Gu, Pokhrel, Hanasaki, Guo, Xiong and Schlenker2023; see insight 3). Nonlinear effects of separate events, such as cyclones and fires, can lead to a permanently altered equilibrium ecological state (Ibanez et al., Reference Ibanez, Platt, Bellingham, Vieilledent, Franklin, Martin, Menkes, Pérez-Salicrup, Russell-Smith and Keppel2022).
Compound ocean events, such as marine heatwaves alongside changes in oxygen availability, ocean acidity, and/or net primary production, can impact marine ecosystems at the individual, population, and community levels (Burger et al., Reference Burger, Terhaar and Frölicher2022; Gruber et al., Reference Gruber, Boyd, Frölicher and Vogt2021; Le Grix et al., Reference Le Grix, Zscheischler, Rodgers, Yamaguchi and Frölicher2022). For example, some of the devastating impacts of the Northeast Pacific 2013–2015 marine heatwave, including extreme mortality and reproductive failure of sea birds, mass stranding of whales and sea lions, and shifts in species composition toward warm-water species, were amplified by co-occurring extreme ocean acidity, low oxygen, and low net primary production conditions (Gruber et al., Reference Gruber, Boyd, Frölicher and Vogt2021). Compound ocean events, such as concurrent marine heatwaves and low oxygen events, can impact food security and cause considerable societal impacts. Increasingly, these events are co-occurring with land events, multiplying the impact (Pathmeswaran et al., Reference Pathmeswaran, Sen Gupta, Perkins-Kirkpatrick and Hart2022).
Studies oncompounding drivers are in the early stages of development but, following on the multi-hazard model, these can improve disaster risk assessment. More specifically, ‘compound event thinking’ improves early warning, emergency response, infrastructure management, long-term planning, and capacity building (van den Hurk et al., Reference van den Hurk, White, Ramos, Ward, Martius, Olbert, Roscoe, Goulart and Zscheischler2023). Adaptation pathways, typically designed for univariate hazards, could also be extended to compounding hazards in many cases (Schlumberger et al., Reference Schlumberger, Haasnoot, Aerts and de Ruiter2022). Niggli et al. (Reference Niggli, Huggel, Muccione, Neukom and Salzmann2022) assessed cascading impacts of hot–dry compound events, showing interlinking effects throughout socioeconomic systems – health, energy, and agricultural impacts cascading on to public services, society, and culture. So far, however, there is limited evidence that adaptation efforts take into account compound events, and maladaptive characteristics are particularly prominent in this context (Simpson et al., Reference Simpson, Williams, Mach, Berrang-Ford, Biesbroek, Haasnoot, Segnon, Campbell, Musah-Surugu and Joe2023). Such shortcomings are partly due to a lack of knowledge about the physical system, and partly to the difficulty in translating knowledge into action.
The last few years have seen the occurrence of exceptional events far outside the previous local historical range, with severe socioecological impacts. Events are being connected to combinations of antecedent and/or simultaneous drivers that only together were able to achieve the observed conditions. Most prominently, this includes heatwaves, for example the events in western North America in June 2021, as the integrated outcome of processes acting across scales, including atmospheric ridging, low soil moisture, and latent heating from upwind precipitation (Bartusek et al., Reference Bartusek, Kornhuber and Ting2022). Even when occurring in a single region, these exceptional events can be compound by heightening multiple types of impacts simultaneously, such as simultaneous heat stress, wildfire risk, and air pollution (Rosenthal et al., Reference Rosenthal, Benmarhnia, Ahmadov, James and Marlier2022), or heat–drought and heat–flood linkages (Gu et al., Reference Gu, Chen, Yin, Slater, Wang, Guo, Feng, Qin and Zhao2022).
Efforts to quantify how extreme weather on land and oceans will respond to climate change benefit from consideration of how discrete climate hazards can interact with and intensify each other. Using improved modeling tools and new statistical methods, an emerging body of evidence is also revealing that, relative to singular hazards, the impacts from compound events are more likely to exacerbate each other, in part because of longer recovery timescales (de Ruiter et al., Reference de Ruiter, Couasnon, van den Homberg, Daniell, Gill and Ward2020). This interconnectedness emphasizes the need for cooperation at the scales over which compound event impacts are shared, which vary by event and sector but are typically larger and longer than many existing decision-making frameworks account for. There is a new level of recognition that the impacts of compound events are substantially shaped by local preconditions, whether societal or environmental, making those contextual features of crucial importance to assess and incorporate.
3.7 Insight 7. Accelerated mountain glacier loss
Mountain glaciers are highly sensitive indicators of climate change. Recent advances related to satellite observations and modeling have enhanced our ability to measure glaciers' response to climate change and project their evolution over the next century. In comparison to the vast ice sheets in Greenland and Antarctica, mountain glaciers occupy much smaller areas and account for a sea-level rise potential of only about 30 cm (Millan et al., Reference Millan, Mouginot, Rabatel and Morlighem2022). However, mountain glaciers respond to changes in atmospheric forcing over shorter temporal scales, compared to ice sheets, such that their mass loss explains almost one-quarter of currently observed rates of sea-level rise (Hugonnet et al., Reference Hugonnet, McNabb, Berthier, Menounos, Nuth, Girod, Farinotti, Huss, Dussaillant, Brun and Kääb2021). Natural hazards such as glacier outburst floods and collapses are also key threats resulting from accelerated mountain glacier loss (Emmer et al., Reference Emmer, Allen, Carey, Frey, Huggel, Korup, Mergili, Sattar, Veh, Chen, Cook, Correas-Gonzalez, Das, Diaz Moreno, Drenkhan, Fischer, Immerzeel, Izagirre, Joshi and Yde2022; Taylor et al., Reference Taylor, Robinson, Dunning, Carr and Westoby2023). Furthermore, glaciers have considerable touristic, spiritual, and ideological value, and contribute to healthy mountain environments. During the summer, especially in times of drought, glacier meltwater is vital for maintaining river flow (Immerzeel et al., Reference Immerzeel, Lutz, Andrade, Bahl, Biemans, Bolch, Hyde, Brumby, Davies, Elmore, Emmer, Feng, Fernández, Haritashya, Kargel, Koppes, Kraaijenbrink, Kulkarni, Mayewski and Baillie2020) thereby providing freshwater that supports mountain and downstream regions, the quantity and quality of drinking water, irrigation, aquifer replenishment, ecosystems functions, biodiversity, and fluvial transport.
Present-day observations of glacier change reveal a loss of 267 ± 16 Gt/year with a clear acceleration over the last two decades (Hugonnet et al., Reference Hugonnet, McNabb, Berthier, Menounos, Nuth, Girod, Farinotti, Huss, Dussaillant, Brun and Kääb2021). As these glaciers retreat, biodiversity in high-alpine catchments may strongly decrease, compromising ecosystem function, but also opportunities for species to occupy new territory (Bosson et al., Reference Bosson, Huss, Cauvy-Fraunié, Clément, Costes, Fischer, Poulenard and Arthaud2023; Cauvy-Fraunié & Dangles, Reference Cauvy-Fraunié and Dangles2019; Wilkes et al., Reference Wilkes, Carrivick, Castella, Ilg, Cauvy-Fraunié, Fell, Füreder, Huss, James, Lencioni, Robinson and Brown2023). Glacier retreat is further accelerated by the growth of moraine-dammed glacial lakes. Ice melt below the water surface, unaccounted for by available estimates, indicates that glacier mass loss is, for example, 7 ± 2% greater than previously reported in the Greater Himalayas (Zhang et al., Reference Zhang, Bolch, Yao, Rounce, Chen, Veh, King, Allen, Wang and Wang2023; that study also estimates the global loss to be 12% greater, though the uncertainties in the underlying data are substantial). Downstream populations are also growing rapidly, such that roughly 15 million people worldwide are potentially exposed to glacial lake outburst floods with the greatest impacts found in High Mountain Asia and the Andes (Taylor et al., Reference Taylor, Robinson, Dunning, Carr and Westoby2023). In High Mountain Asia, the development and expansion of glacial lakes is expected to triple the risk of moraine-dammed glacial lake outburst floods over the next century (Zheng et al., Reference Zheng, Allen, Bao, Ballesteros-Cánovas, Huss, Zhang, Li, Yuan, Jiang, Yu, Chen and Stoffel2021). However, outburst floods from ice-dammed lakes were found to become less intense, and are expected to decrease in frequency over the next century (Veh et al., Reference Veh, Lützow, Tamm, Luna, Hugonnet, Vogel, Geertsema, Clague and Korup2023).
New global glacier projections estimate that glaciers will lose 26% (+1.5°C) to 41% (+4°C) of their current volume by 2100 depending on the global temperature change scenario (Rounce et al., Reference Rounce, Hock, Maussion, Hugonnet, Kochtitzky, Huss, Berthier, Brinkerhoff, Compagno, Copland, Farinotti, Menounos and Mcnabb2023) (Figure 7). Mountain glaciers will thus continue to be one of the primary contributors to sea-level rise throughout the 21st century. Relative mass loss varies greatly at regional scales, with mid-latitude regions (e.g. Western Canada, Central Europe, Caucasus) being expected to experience widespread deglaciation for scenarios with global average warming beyond 3°C. Limiting the temperature increase by reducing GHG emissions is thus critical for limiting glacier contribution to sea-level rise and preserving these glacierized regions.

Figure 7. Regional glacier mass change and contributions to sea-level rise from 2015 to 2100. Disks show global and regional projections of glacier mass remaining by 2100, relative to 2015, for global mean temperature change scenarios. Disks are scaled based on each region's contribution to global mean sea-level rise from 2015 to 2100 for the +2°C scenario by 2100 relative to preindustrial levels. Nested rings are colored by temperature change scenarios showing normalized mass remaining in 2100. Regional sea-level rise contributions larger than 1 mm sea-level equivalent (SLE) for the +2°C scenario are printed in the center of the ring charts. The color of the rings for each region indicates the risk to livelihoods and the economy from changing mountain water resources between 1.5 and 2°C global warming (IPCC, 2022a, CCP5.3). The gridded population density (people per km2) is also shown (grey scale). Glaciers are shown in blue. Modified from Rounce et al. (Reference Rounce, Hock, Maussion, Hugonnet, Kochtitzky, Huss, Berthier, Brinkerhoff, Compagno, Copland, Farinotti, Menounos and Mcnabb2023).
The biggest challenges for quantifying present and future mountain glacier loss are related to observations and modeling. While measuring decadal-scale glacier mass changes from space is now possible for every glacier on Earth (Hugonnet et al., Reference Hugonnet, McNabb, Berthier, Menounos, Nuth, Girod, Farinotti, Huss, Dussaillant, Brun and Kääb2021), observations of year-to-year variability at coarser scales need to be integrated, and disentangling mass loss due to changes in snow accumulation and melt is still hampered by limited in situ information from data-scarce regions. Despite important advances, global models still rely on estimates of bedrock topography that include large uncertainties (Millan et al., Reference Millan, Mouginot, Rabatel and Morlighem2022) and are also hampered by a lack of direct ice thickness measurements. Similarly, remote-sensing data will continue to provide unique opportunities to improve the representation of important processes in models, such as frontal ablation for marine- and lake-terminating glaciers (Zhang et al., Reference Zhang, Bolch, Yao, Rounce, Chen, Veh, King, Allen, Wang and Wang2023) or the impact of debris cover (Rounce et al., Reference Rounce, Hock, Maussion, Hugonnet, Kochtitzky, Huss, Berthier, Brinkerhoff, Compagno, Copland, Farinotti, Menounos and Mcnabb2023). In all cases, additional in situ observations to better constrain remotely sensed data and improve representation of processes in models are key areas of future work that will help reduce uncertainties in glacier projections. Transforming these projections into products that support adaptation and mitigation efforts will benefit from directly coupling atmospheric, cryospheric, and hydrological models (Yao et al., Reference Yao, Bolch, Chen, Gao, Immerzeel, Piao, Su, Thompson, Wada, Wang, Wang, Wu, Xu, Yang, Zhang and Zhao2022), incorporating high-resolution models to ensure projections are provided at the scale required to inform disaster risk management strategies, and implementing effective adaptation programs that build trust between governments and local populations (see insight 9).
The impact of climate change on mountain environments is highly diverse. Beyond glacier mass loss, it results in permafrost thawing and various cascading hazards, including avalanches, landslides, debris flows, and flooding. Water resource systems are directly affected, including the drying of springs, changes in mountain snow cover, and expansion of glacial lakes (Prakash, Reference Prakash2020). Consequently, socioeconomic development and ecosystems are impacted (Aggarwal et al., Reference Aggarwal, Frey, McDowell, Drenkhan, Nüsser, Racoviteanu and Hoelzle2022). The SDGs for ensuring well-being and building resilience face challenges in the face of these changing mountain ecosystems. Adaptation strategies vary across sectors and regions, highlighting a need for more stakeholder cooperation to ensure effective implementation and management (Aggarwal et al., Reference Aggarwal, Frey, McDowell, Drenkhan, Nüsser, Racoviteanu and Hoelzle2022; Pandey et al., Reference Pandey, Prakash and Werners2021). The establishment of a loss and damage fund at COP27 highlights the need for substantial adaptation implementation in the disaster risk reduction sector while also addressing climate justice issues by supporting the most vulnerable (Wentz et al., Reference Wentz, Merner, Franta, Lehmen and Frumhoff2023) (see insight 9 on adaptation justice). Still, too few risk control measures have been implemented to date to address the impacts of global climate change in mountain regions (Alcántara-Ayala et al., Reference Alcántara-Ayala, Pasuto and Cui2022).
The number of people affected by mountain glacier loss has risen substantially. Regions with significant mountainous areas and high population density, such as the Himalayas, are particularly vulnerable (Figure 7). Since the 1960s the number of people who are largely or fully dependent on water from mountains has increased from approximately 0.6 to 2 billion worldwide (IPCC, 2022a, CCP5). The mountains of the Hindu Kush Himalaya are an essential source of fresh water for 240 million people living in this region and 1.65 billion downstream (Sharma et al., Reference Sharma, Molden, Rahman, Khatiwada, Zhang, Singh, Yao, Wester, Wester, Mishra, Mukherji and Shrestha2019; Singh et al., Reference Singh, Tanvir Hassan, Hassan and Bharti2020). Substantial atmospheric warming and pronounced dry seasons will continue to drive glacier mass loss and thus amplify water stress. Regions such as Central Asia, South Asia, and tropical and subtropical western South America, are expected to experience the most significant impacts from changing water availability throughout the 21st century (Lutz & Biemans, Reference Lutz and Biemans2022). The changes to the water cycle, including variable timing of glacier and snow melt, have diverse impacts on water availability and may lead to tensions or conflicts over resources, especially in seasonally dry regions (IPCC, 2022a, CCP5). Further commitments to reducing GHG emissions will help offset the worst of these impacts. Effective, community-driven adaptation strategies will be key in supporting resource and disaster risk management, especially for vulnerable communities.
3.8 Insight 8. Immobility in the face of climate risks: between constraints and agency
People who are unable or unwilling to relocate from high-risk areas may face even greater challenges than those who are displaced by climate-related events. Some climate-impacted communities are limited in their mobility options by economic, political, socio-cultural, and physical constraints, and are unable to move (Schewel, Reference Schewel2019). Demographic factors, access to information on safe accommodation, safe migration opportunities, and labor markets at destination can also influence (im)mobility outcomes (Siddiqui et al., Reference Siddiqui, Bhuiyan, Das, Chakraborty and Hasan2018). Individual differences in ability to move can create gendered and other forms of inequities at the household level (Ayeb-Karlsson, Reference Ayeb-Karlsson2020). However, not all immobility is involuntary. While involuntary immobility has been widely recognized at least since the UK Foresight report (Foresight UK, 2011), increasing evidence shows that individuals and communities facing high displacement risk are articulating a desire to stay, sometimes in opposition to planned relocation (van der Geest et al., Reference van der Geest, de Sherbinin, Gemenne and Warner2023; Wiegel et al., Reference Wiegel, Warner, Boas and Lamers2021; Yee et al., Reference Yee, Piggott-McKellar, McMichael and McNamara2022b), invoking questions of social justice (Boas et al., Reference Boas, Wiegel, Farbotko, Warner and Sheller2022). More on adaptation justice is provided in insight 9.
Recent studies show an increase in involuntary immobility particularly among the poorest populations, due to the negative impacts of climate change on economies and resources. Climate change might decrease emigration rates by over 10% among the lowest-income groups by 2100, under medium development and climate scenarios, compared to no climate change, and up to 35% in more pessimistic scenarios (Benveniste et al., Reference Benveniste, Oppenheimer and Fleurbaey2022). Rikani et al. (Reference Rikani, Otto, Levermann and Schewe2023) find that climate change decreases immigration and emigration predominantly in countries located in sub-Saharan Africa and South Asia. In terms of region-level bilateral flows, there is a decrease in migration within Africa, South Asia, and West Asia, while migration within Europe and the former Soviet Union has increased, suggesting that mobility is facilitated in wealthier regions and inhibited in the poorest (Rikani et al., Reference Rikani, Otto, Levermann and Schewe2023).
Recent studies in coastal Bangladesh illustrate how (im)mobility outcomes in climate hazard contexts can result from a rational decision-making process shaped by intersecting community- and individual-level factors (Khatun et al., Reference Khatun, Ahsan, Afrin, Warner, Ahsan, Mallick and Kumar2022; Mallick et al., Reference Mallick, Best, Carrico, Ghosh, Priodarshini, Sultana and Samanta2023a; Paul et al., Reference Paul, Rahman, Lu and Crawford2022). Community-level factors, including social/community cohesion, economic, and political conditions, contribute to overall livelihood conditions and shape place attachment (Figure 8). Climate impacts and risks affect both individual and community levels. At the individual level, personal/household characteristics, risk perception and tolerance, influence self-efficacy. Self-efficacy refers to the perceived coping capacity to withstand or respond to climate impacts and risks, which, in turn, affects an individual's capability and aspiration to migrate (Mallick et al., Reference Mallick, Best, Carrico, Ghosh, Priodarshini, Sultana and Samanta2023a).

Figure 8. Intersecting community and individual level factors influencing individual decision-making processes regarding immobility in climate-risk contexts. Adapted from Mallick et al. (Reference Mallick, Best, Carrico, Ghosh, Priodarshini, Sultana and Samanta2023a).
Other recent case studies show that populations at risk of displacement express a strong desire to stay in their current location in response to proposed relocation programs (Farbotko et al., Reference Farbotko, Dun, Thornton, McNamara and McMichael2020; Wiegel et al., Reference Wiegel, Warner, Boas and Lamers2021). Within these communities, individuals possess valuable local knowledge of habitability, exhibit profound place attachment, and prioritize safeguarding cultural identity and political agency, despite climate-related risks. In essence, the perceived risks associated with relocation, including threats to livelihood, social connection, personal safety, and access to services, outweigh the perceived risks posed by climate change (Farbotko & Campbell, Reference Farbotko and Campbell2022; Santos & Mourato, Reference Santos and Mourato2022; Yee et al., Reference Yee, McNamara, Piggott-McKellar and McMichael2022a, Reference Yee, Piggott-McKellar, McMichael and McNamara2022b). While relocation programs can contribute to adaptation (Khatun et al., Reference Khatun, Ahsan, Afrin, Warner, Ahsan, Mallick and Kumar2022), the desire of some communities to stay in place despite the climate risks might actually rise in reaction to solutions they perceived as maladaptive or as a threat to established rights (Farbotko et al., Reference Farbotko, Dun, Thornton, McNamara and McMichael2020); indicating resistance to imposed, top-down policies (Boas et al., Reference Boas, Wiegel, Farbotko, Warner and Sheller2022). Resistance to relocation can signal mistrust in government, especially where previous relocations have led to reduced employment opportunities, limited access to services, and broken social capital (Gunathilake et al., Reference Gunathilake, Jayathilake, Fernando, Jayasinghe, Amaratunga, Haigh, Hamza, Amaratunga, Haigh, Malalgoda, Jayakody and Senanayake2023).
Immobility can thus be a political act, representing resistance and defying expectations of future displacement. These findings contest the dominant policy and media discourse on mass migration induced by climate change (Durand-Delacre et al., Reference Durand-Delacre, Bettini, Nash, Sterly, Gioli, Hut, Boas, Farbotko, Sakdapolrak, de Bruijn, Tripathy Furlong, van der Geest, Lietaer, Hulme, Bohm and Sullivan2021) by demonstrating that despite environmental degradation and climate risks, some may decide to stay put; thus questioning the notion of a universal aspiration to migrate (Mallick et al., Reference Mallick, Rogers and Sultana2022). Such decisions are often constrained by underlying development failures that limit life opportunities and choices.
Recent research highlights the importance of understanding immobility across different scales (Mallick et al., Reference Mallick, Priovashini and Schanze2023b) and a need to better understand how individual, household, familial, and community experiences of immobility interact. Understanding immobility within temporal and political contexts and recognizing it as part of local response to climate risk (including temporary immobility and symbolic resistance) could enable more nuanced interpretations. This can inform human-centered policymaking that enables informed and culturally respectful choices and provides safer options for migrants and non-migrants. The existing literature on participatory decision-making, and its critique, can serve as a foundation for such research and policy innovation.
While climate mobility has been the focus of climate change and human mobility policy, there are now emerging calls for governance of climate immobility (Naser et al., Reference Naser, Mallick, Priodarshini, Huq and Bailey2023; Thornton et al., Reference Thornton, Serraglio and Thornton2023). Addressing involuntary immobility requires policy initiatives that reduce the need to move and measures that increase people's ability to move, for example by reducing legal and political barriers to mobility. Policies on adaptation, mitigation, disaster risk reduction, and resilience building intersect with climate immobility, but largely fail to explicitly address it (Benveniste et al., Reference Benveniste, Oppenheimer and Fleurbaey2022; Farbotko et al., Reference Farbotko, Dun, Thornton, McNamara and McMichael2020; Wiegel et al., Reference Wiegel, Boas and Warner2019). Currently, the global goal on adaptation and discussions surrounding loss and damage exclude the risks and costs associated with immobility. There is a need for further research into the economic and non-economic costs of immobility to guide the development of adaptation strategies and policies, with a particular focus on marginalized groups, in order to mitigate overall risk. Multiple policy approaches are required, respecting the rights of those who want to move, those who want to stay put, and those who resist planned relocation. Viable and effective strategies must be developed considering specific vulnerabilities, risk perceptions, and context-based decision-making processes. Relocation frameworks that are derived from inclusive planning should explicitly recognize and support those who wish to stay (Farbotko et al., Reference Farbotko, Dun, Thornton, McNamara and McMichael2020).
There is some hope that immobility may be recognized in climate finance through the loss and damage fund (Thornton et al., Reference Thornton, Serraglio and Thornton2023); yet, the lack of attention it has received within loss and damage governance in comparison to climate-induced migration, disaster displacement, and international security raises uncertainty about its inclusion (Jackson et al., Reference Jackson, N'Guetta, De Rosa, Scown, Dorkenoo, Chaffin and Boyd2023). This is supported by the recent findings of Mombauer et al. (Reference Mombauer, Link and van der Geest2023), which indicate that only a minority of National Adaptation Plans (NAPs) and Nationally Determined Contributions (NDCs) integrate considerations for populations unwilling or unable to move (Box 6).
Box 6. Voluntary immobility: challenging government relocation expectations
Voluntary immobility is a characteristic of some communities in Fiji who oppose national government relocation planning (Yee et al., Reference Yee, McNamara, Piggott-McKellar and McMichael2022a, Reference Yee, Piggott-McKellar, McMichael and McNamara2022b). In a recent study in a remote village in Tuvalu, it was found that the population was growing due to migration from the urban capital. People were motivated to move to indigenous lands where they held family land rights and sought to nurture indigenous culture. These instances of ‘anti-displacement mobilities’ and ‘re-emplacements’ challenge expectations of Tuvaluans relocating internationally (Farbotko, Reference Farbotko2022).
3.9 Insight 9. New approaches enhance justice of adaptation action
Adaptation opportunities are unevenly distributed and have varying degrees of success, in large part due to injustices related to who receives and controls funding, who designs and implements strategies, and who is affected by them. Justice concerns are increasingly mentioned in the Adaptation Gap Reports (UNEP, 2023), indicating an increased awareness. Justice has emerged as a key condition for effective adaptation, with new empirical work building on previous theoretical developments. While conceptualizations of adaptation justice exist (Juhola et al., Reference Juhola, Heikkinen, Pietilä, Groundstroem and Käyhkö2022; Orlove, Reference Orlove2022), their application in adaptation planning and implementation remains scarce, ambiguous, and rarely accounted for. A global review of policy tools for adaptation found that the most vulnerable and marginalized, who are also the most heavily impacted by climate change, are not considered in the majority of adaptation plans (Ulibarri et al., Reference Ulibarri, Ajibade, Galappaththi, Joe, Lesnikowski, Mach, Musah-Surugu, Nagle Alverio, Segnon, Siders, Sotnik, Campbell, Chalastani, Jagannathan, Khavhagali, Reckien, Shang, Singh and Zommers2022). Globally, there is limited evidence of just outcomes from adaptation strategies and plans (Araos et al., Reference Araos, Jagannathan, Shukla, Ajibade, Coughlan de Perez, Davis, Ford, Galappaththi, Grady, Hudson, Joe, Kirchhoff, Lesnikowski, Alverio, Nielsen, Orlove, Pentz, Reckien, Siders and Turek-Hankins2021) due to a lack of monitoring. More concerns are raised from a review of the effectiveness of adaptation plans. Currently, the attention to social cleavages, such as gender (Roy et al., Reference Roy, Prakash, Some, Singh, Bezner Kerr, Caretta, Conde, Ferre, Schuster-Wallace, Tirado-von Der Pahlen, Totin, Vij, Baker, Dean, Hillenbrand, Irvine, Islam, McGlade, Nyantakyi-Frimpong and Tandon2022), poverty, and ethnicity (Araos et al., Reference Araos, Jagannathan, Shukla, Ajibade, Coughlan de Perez, Davis, Ford, Galappaththi, Grady, Hudson, Joe, Kirchhoff, Lesnikowski, Alverio, Nielsen, Orlove, Pentz, Reckien, Siders and Turek-Hankins2021) in adaptation planning is very limited in scope (principally focused on gender) and rarely takes an intersectional approach that might better capture the ways in which vulnerability and risk take shape.
Recent research on adaptation justice highlights the social and political roots of the unevenness of observed and projected impacts of climate change (Juhola et al., Reference Juhola, Heikkinen, Pietilä, Groundstroem and Käyhkö2022; Orlove et al., Reference Orlove, Sherpa, Dawson, Adelekan, Alangui, Carmona, Coen, Nelson, Reyes-García, Rubis, Sanago and Wilson2023). While acknowledging the physical processes that shape hazards and exposure, adaptation justice research emphasizes the socioeconomic structures that drive climate vulnerability, making adaptation unavailable to many, and destructive to some. These structures have implications for different justice components (Figure 9): distributive, restorative, recognition, procedural, and epistemic.

Figure 9. Components of adaptation justice and implications in adaptation planning and processes. Based on Juhola et al. (Reference Juhola, Heikkinen, Pietilä, Groundstroem and Käyhkö2022) and Orlove et al. (Reference Orlove, Sherpa, Dawson, Adelekan, Alangui, Carmona, Coen, Nelson, Reyes-García, Rubis, Sanago and Wilson2023).
The factors that produce unjust outcomes have been observed at different scales. At the international scale, insufficient funding and structural biases in funding mechanisms reflect a lack of recognitional justice and procedural justice (Ciplet et al., Reference Ciplet, Falzon, Uri, Robinson, Weikmans and Roberts2022; Islam, Reference Islam2022). This prevents funds from reaching those who need them most, in turn hamper distributive and restorative justice (the latter being most relevant regarding compensation for losses and damages). At the local level, communities face structural barriers to implementation (e.g. Klepp & Fünfgeld, Reference Klepp and Fünfgeld2022). Researchers in this field argue for a fundamental reconsideration of how funders operate and how funds are distributed (Browne, Reference Browne2022; Ciplet et al., Reference Ciplet, Falzon, Uri, Robinson, Weikmans and Roberts2022). For example, many communities lack the support needed to apply for funding or to complete the burdensome reporting requirements attached to most sources of funding. Similarly, loss and damage funds should be arranged as a grant-based programmatic financing mechanism, easily accessible by communities in need.
Brink et al. (Reference Brink, Falla and Boyd2023) documented social resistance emerging in the face of adaptation plans that are not perceived as just. Examples include forced relocation plans (see insight 8 in immobility), imposition of food crops and technocratic practices, or the use of labels such as ‘climate refugee’ (Brink et al., Reference Brink, Falla and Boyd2023). Here we highlight three recent conceptual advances for adaptation justice with practical application: the adaptation justice index (Juhola et al., Reference Juhola, Heikkinen, Pietilä, Groundstroem and Käyhkö2022), adaptation rationales (Carr & Nalau, Reference Carr and Nalau2023), and locally led adaptation (LLA) (Rahman et al., Reference Rahman, Falzon, Robinson, Kuhl, Westoby, Omukuti, Schipper, McNamara, Resurrección, Mfitumukiza and Nadiruzzaman2023). Juhola et al. (Reference Juhola, Heikkinen, Pietilä, Groundstroem and Käyhkö2022) developed the ‘adaptation justice index’ and proposed concrete steps toward more just adaptation planning by identifying where in the planning process justice ought to be included. This approach proposes a shift from a narrow model of stakeholder engagement to full and long-term co-produced collaborative partnership (for procedural and distributive justice), operating across scales of social organization and accounting for short- and long-term implications of adaptation actions (Orlove et al., Reference Orlove, Sherpa, Dawson, Adelekan, Alangui, Carmona, Coen, Nelson, Reyes-García, Rubis, Sanago and Wilson2023). Moreover, full, collaborative partnerships bring together the holders of local and Indigenous knowledge systems and values (epistemic justice), while also addressing present-day structural issues, many of which developed historically from colonialism (Orlove et al., Reference Orlove, Sherpa, Dawson, Adelekan, Alangui, Carmona, Coen, Nelson, Reyes-García, Rubis, Sanago and Wilson2023; Whyte, Reference Whyte2021).
‘Adaptation rationales’ are impact pathways that represent the logic of an adaptation action, explicitly guiding the development of pathways that link priorities, actions, and outcomes (Carr & Nalau, Reference Carr and Nalau2023). They are not focused on technical adaptation fixes alone and can incorporate solutions to improve livelihoods and sustainable development, indicating that good rationales avoid worsening inequality and increasing vulnerability. At smaller scales, many adaptation projects suffer from poorly constructed (or non-explicit) adaptation rationales, reflecting gaps in procedural and epistemic justice. To achieve just adaptation, the literature suggests the importance of strengthening the design, implementation, monitoring, and evaluation of adaptation plans (Orlove, Reference Orlove2022). Transparent, well-constructed adaptation rationales with clearly articulated benefits help minimize uneven distribution of those benefits (Carr & Nalau, Reference Carr and Nalau2023). By devising plans for a broad set of adaptation benefits framed around reduced exposure, reduced sensitivity, and increased adaptive capacity, justice can be placed at the fore. Moreover, strong adaptation rationales enable the effective monitoring, evaluation, and learning of the different components of justice (Juhola & Käyhkö, Reference Juhola and Käyhkö2023).
LLA (Rahman et al., Reference Rahman, Falzon, Robinson, Kuhl, Westoby, Omukuti, Schipper, McNamara, Resurrección, Mfitumukiza and Nadiruzzaman2023) hinges on fostering bottom-up initiatives and respecting community autonomy that corrects unequal power distribution (Pisor et al., Reference Pisor, Basurto, Douglass, Mach, Ready, Tylianakis, Hazel, Kline, Kramer, Lansing, Moritz, Smaldino, Thornton and Jones2022) and sharing knowledge and building capacity (Huggel et al., Reference Huggel, Bouwer, Juhola, Mechler, Muccione, Orlove and Wallimann-Helmer2022). Recent examples suggest this approach promotes more just outcomes in adaptation planning and implementation (e.g. Klepp & Fünfgeld, Reference Klepp and Fünfgeld2022). Allowing adaptation decisions to be made with inputs from different scales, while using tools like the adaptation justice index to deepen the evaluation of adaptation rationales, will help prevent maladaptation and facilitate the co-creation of inclusive pathways toward just climate futures.
These three approaches – the adaptation justice index, adaptation rationales, and LLA – illustrate concrete, actionable steps proposed and implemented as ways to assure that adaptation activities are just, addressing the needs of the most vulnerable and marginalized (Box 7).
Box 7. Increases in resilience can be secured in all regions and communities by addressing adaptation justice in adaptation plans
(A) Hurricane Harvey in Harris County, Texas (USA) especially impacted low-income neighborhoods. Those affected by the hurricane who were based outside of floodplains received no prior warning, affecting their preparedness (Smiley et al., Reference Smiley, Noy, Wehner, Frame, Sampson and Wing2022).
(B) Drawing on Indigenous knowledge and scientific information, villagers in Fiji planned their own relocation of coastal villages impacted by coastal erosion and saltwater intrusion (McMichael et al., Reference McMichael, Katonivualiku and Powell2019). The decision was made possible thanks to land-use laws enabling the relocation which in turn supported livelihoods, use of terrestrial and marine resources, and maintained cultural values and connections to ritual sites (Orlove et al., Reference Orlove, Sherpa, Dawson, Adelekan, Alangui, Carmona, Coen, Nelson, Reyes-García, Rubis, Sanago and Wilson2023).
3.10 Insight 10. A justice lens for mitigation strategies in the food sector
Despite mounting evidence and available strategies for mitigation, GHG emissions from food systems still amount to approximately 31% of global emissions (IPCC, 2022b, Ch12, p. 1280). Without significant transformations, current food systems on their own put at risk the 1.5°C target, pushing global warming toward 2°C by 2100 (under ‘business as usual trajectories’) (Clark et al., Reference Clark, Domingo, Colgan, Thakrar, Tilman, Lynch, Azevedo and Hill2020). At the same time, over 700 million people are estimated to face hunger while marginalized groups such as women, racial minority groups, Indigenous communities, and small-scale farmers are disproportionately affected by food insecurity and climate change (FAO et al., 2022; Juskaite & Haug, Reference Juskaite and Haug2023). In the same way as the notion of just transitions has emerged to enable the transformation of energy systems (Heffron & McCauley, Reference Heffron and McCauley2022), it is also increasingly understood as central for the transformation of food systems (Tribaldos & Kortetmäki, Reference Tribaldos and Kortetmäki2022).
The transformation of global food systems for climate action is challenged by the tension to act urgently while ensuring that no one is left behind (Woodhill et al., Reference Woodhill, Kishore, Njuki, Jones and Hasnain2022). This tension is exacerbated by the polarized debate on whether solutions must be localized or coordinated at a more global scale (the local–global food debate) (Wood et al., Reference Wood, Queiroz, Deutsch, González-Mon, Jonell, Pereira, Sinare, Svedin and Wassénius2023) and the siloed decision-making processes dominating policy for the agricultural sector (FAO et al., 2022; McGreevy et al., Reference McGreevy, Rupprecht, Niles, Wiek, Carolan, Kallis, Kantamaturapoj, Mangnus, Jehlička, Taherzadeh, Sahakian, Chabay, Colby, Vivero-Pol, Chaudhuri, Spiegelberg, Kobayashi, Balázs, Tsuchiya and Tachikawa2022). Current agricultural policies show insufficient consideration of social vulnerabilities, regional disparities in geography, culture and socioeconomic conditions (Ambikapathi et al., Reference Ambikapathi, Schneider, Davis, Herrero, Winters and Fanzo2022), technological readiness, vested interests, and power imbalances (Béné, Reference Béné2022; Zurek et al., Reference Zurek, Hebinck and Selomane2022). For decades the agrifood industry, subsidized by national governments and aided by global trade agreements, has created a political economy that reinforces unsustainability and injustices worldwide (Juskaite & Haug, Reference Juskaite and Haug2023; Woodhill et al., Reference Woodhill, Kishore, Njuki, Jones and Hasnain2022). Acknowledging and addressing the injustices (re)produced in contemporary food systems (Tribaldos & Kortetmäki, Reference Tribaldos and Kortetmäki2022) and how structures of power shape socioeconomic change (e.g. Babic & Sharma, Reference Babic and Sharma2023) are crucial prerequisites for realizing the mitigation potential of food system transformation (McGreevy et al., Reference McGreevy, Rupprecht, Niles, Wiek, Carolan, Kallis, Kantamaturapoj, Mangnus, Jehlička, Taherzadeh, Sahakian, Chabay, Colby, Vivero-Pol, Chaudhuri, Spiegelberg, Kobayashi, Balázs, Tsuchiya and Tachikawa2022). This insight highlights research developments on food system transformations with a justice lens, focused on solutions that are more just and climate effective.
First, strategies for low-emission diets and production practices, food waste elimination, among others, to transform food systems cannot be implemented as one-size-fits-all solutions. They must be diverse and embedded in regional heterogeneity. Dietary preferences (Ambikapathi et al., Reference Ambikapathi, Schneider, Davis, Herrero, Winters and Fanzo2022), the needs of small-scale producers (Juskaite & Haug, Reference Juskaite and Haug2023; Tschersich & Kok, Reference Tschersich and Kok2022), inequalities in food loss and waste (FAO et al., 2022), regional socioecological contexts (Dengerink et al., Reference Dengerink, Dirks, Likoko and Guijt2021), and the governance of innovation (De Boon et al., Reference De Boon, Sandström and Rose2022) are just some of the dimensions that need to be considered. As a result, food system transformations require broader, sometimes politically charged, discussions on a mix of multiple solutions. For example, a plurality of different narratives are developing in the debate over sustainable and alternative proteins – synthetic meat, plant-based substitutes, or small-scale farming – which open up critical questions on how existing power asymmetries and industrial control might be replicated or possibly avoided (Béné & Lundy, Reference Béné and Lundy2023; Sexton et al., Reference Sexton, Garnett and Lorimer2019). The literature in the field has also argued convincingly for the importance of re-grounding food systems in regional circuits of production and consumption, including shedding light on food system precarity and trade dependencies (Li et al., Reference Li, Jia, Lenzen, Malik, Wei, Jin and Raubenheimer2022a; Mosnier et al., Reference Mosnier, Javalera-Rincon, Jones, Andrew, Bai, Baker, Basnet, Boer, Chavarro, Costa, Daloz, DeClerck, Diaz, Douzal, Howe Fan, Fetzer, Frank, Gonzalez-Abraham, Habiburrachman and Zerriffi2023), as well as recognizing the importance of social innovations (e.g. informal community gardening) in creating resilient biodiverse food systems (Hebinck et al., Reference Hebinck, Selomane, Veen, de Vrieze, Hasnain, Sellberg, Sovová, Thompson, Vervoort and Wood2021).
Second, decision-making processes must acknowledge and address the existing vested interests of decision-making bodies as well as large private actors (Béné, Reference Béné2022; Zurek et al., Reference Zurek, Hebinck and Selomane2022), that may overpower the perspectives of the stakeholders most vulnerable to the impacts of climate change and food insecurity (e.g. farmers, women, Indigenous communities, workers; Juskaite & Haug, Reference Juskaite and Haug2023). Researchers in this field have argued for implementing strategies to curb corporate influence, such as competition policies that account for the impacts of market concentration and measures to strengthen transparency and deprioritize profit making over the right to food (Clapp, Reference Clapp2021). Actively involving as many food system stakeholders, with deliberate effort to engage marginalized communities and diverse cultures in safe enough spaces (Pereira et al., Reference Pereira, Frantzeskaki, Hebinck, Charli-Joseph, Drimie, Dyer, Eakin, Galafassi, Karpouzoglou, Marshall and Moore2020), is also key in garnering legitimacy and accountability (Juskaite & Haug, Reference Juskaite and Haug2023; Tschersich & Kok, Reference Tschersich and Kok2022)). Participatory and inclusive approaches to food system transitions help recognize the inevitability of trade-offs, with winners and losers, and realize more compensatory and just pathways. Without a transformation of governance processes, where appropriate incentives are aligned with actions, the present trajectories of unsustainability will prevail (Béné, Reference Béné2022). Continuous transdisciplinary engagement with stakeholders starting from the problem definition, evidence gathering, impact monitoring, and solution implementation create co-ownership of policy processes and minimize the potential for negative trade-offs (Zurek et al., Reference Zurek, Hebinck and Selomane2022).
Sustainability transformation research shows that a deep change in food systems might take decades (Bodirsky et al., Reference Bodirsky, Chen, Weindl, Soergel, Beier, Molina Bacca, Gaupp, Popp and Lotze-Campen2022); it cannot be delayed any further. While some progress has been made in recent years toward fostering the mitigation potential of food systems transformation, greater efforts are needed to develop transformative policy mixes that address negative trade-offs and integrate the welfare and wellbeing of people and the planet. For example, WWF (2022) reported an increase in countries with at least one measure related to food systems in their NDCs, from 79 to 93% of the 134 updated NDCs. However, less than 50% of the updated NDCs mention the roles of smallholder farmers and Indigenous peoples and local communities in their food system measures.
Transformations within food systems require deliberate design and recognition of inequalities. Policies and strategies must take a multidimensional approach to transformation, incorporating socioeconomic, political, and environmental dimensions and consider multiple solutions at multiple scales, and how these solutions interact to create meaningful change and avoid reproducing power asymmetries. Moreover, inter-sectoral policy mixes that bridge interrelated spheres of food systems and correct or phase-out harmful and unjust policies will be critical for food governance to make a difference. Along these lines, McGreevy et al. (Reference McGreevy, Rupprecht, Niles, Wiek, Carolan, Kallis, Kantamaturapoj, Mangnus, Jehlička, Taherzadeh, Sahakian, Chabay, Colby, Vivero-Pol, Chaudhuri, Spiegelberg, Kobayashi, Balázs, Tsuchiya and Tachikawa2022) lists sufficiency, regeneration, distribution, commons, and care as principles guiding the restructuring of food systems. Finding the correct resolution of policy mix (national, sub-national, hyperlocal) and engaging deliberative and transparent food governance is critical to establishing the effectiveness of just food system transformations (Figure 10).
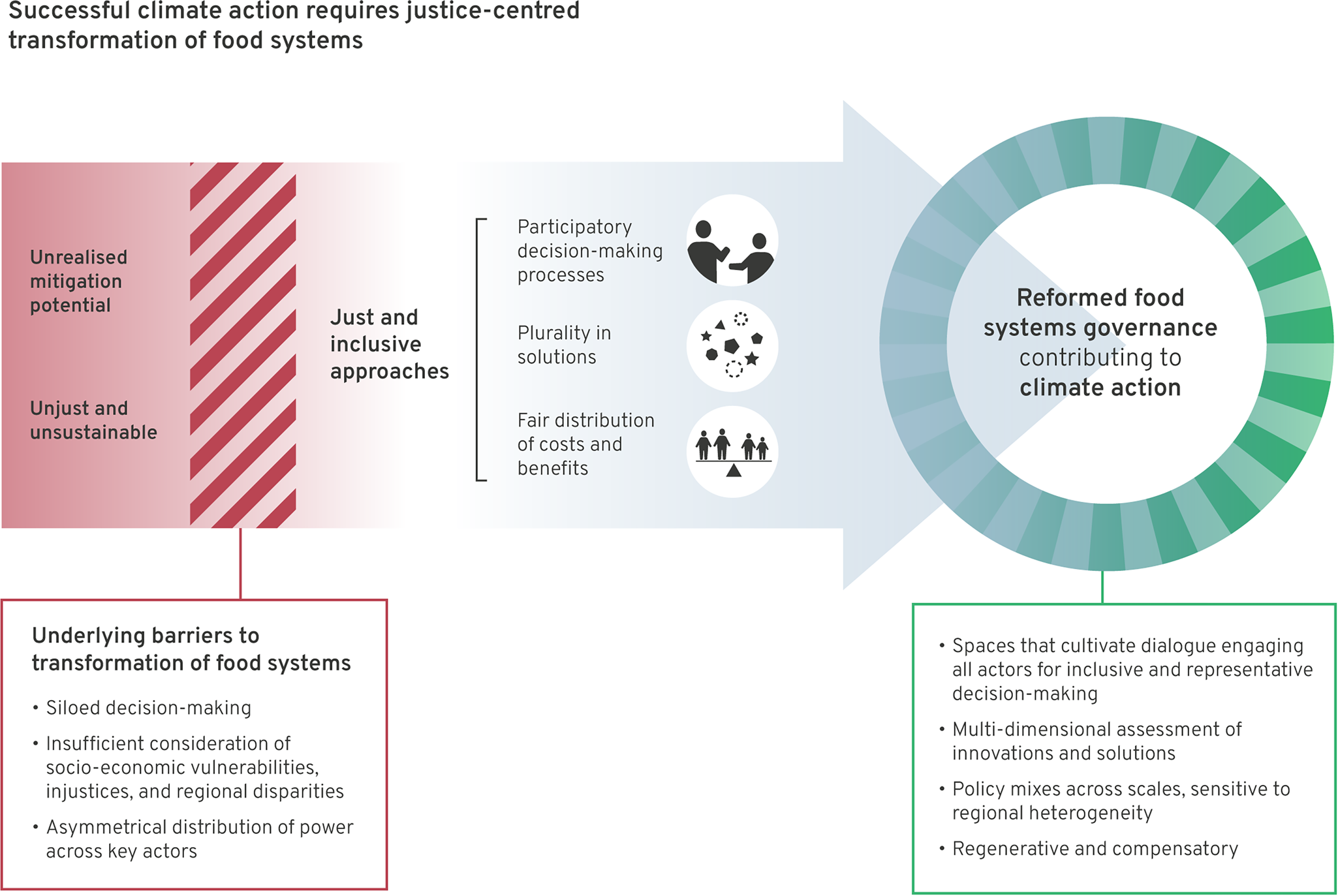
Figure 10. Just climate solutions for food system transformations. Current food system transformations for climate action are constrained by siloed decision-making, insufficient consideration of regional disparities in geographies, innovation, and socioeconomic factors, and power asymmetries across key actors, all of which act as barriers to effective climate action and result in unjust and unsustainable food systems. Integrating more just and inclusive approaches that engage and empower all stakeholders, particularly those most vulnerable to climate change, including co-designing a plurality of solutions with fair distribution of costs and benefits, can help transition toward a governance system more capable of contributing to effcetive climate action across the food sector.
4. Discussion
The current trajectory to overshoot is the result of decades of vastly insufficient action. Overshooting 1.5°C is a dangerous gamble: it will have irreversible impacts on life on Earth, with drastic loss of species and ecosystems (Meyer et al., Reference Meyer, Bentley, Odoulami, Pigot and Trisos2022), and a rising risk of triggering mutliple climate tipping points (Armstrong McKay et al., Reference Armstrong McKay, Staal, Abrams, Winkelmann, Sakschewski, Loriani, Fetzer, Cornell, Rockström and Lenton2022). While the impacts on biodiversity and ecosystems can be abrupt, recovery following the eventual temperature declines will be much slower (Meyer et al., Reference Meyer, Bentley, Odoulami, Pigot and Trisos2022). Unfortunately, the natural carbon sinks, especially on land, might take up less carbon than expected in a warmer future due, among other factors, to increased climate disturbances. Unexpected additional release of GHG from natural carbon sinks might also result from the triggering of climate tipping elements under overshoot, further delaying an eventual warming reversal. This suggests that stabilization and reversal of temperatures might become increasingly difficult. Worryingly, current national mitigation commitments are insufficient to even stay below 2°C of warming (IEA, 2021; Rogelj et al., Reference Rogelj, Fransen, den Elzen, Lamboll, Schumer, Kuramochi, Hans, Mooldijk and Portugal-Pereira2023). Such an outcome would leave a lasting detrimental legacy for life on Earth, unacceptably high risks for human societies and vastly unequal human costs.
The science highlighted in this review makes it clear that overshooting 1.5°C is now all but inevitable in the near term. But the policy implication of this knowledge is decisively not that the Paris Agreement to limit warming to 1.5°C has to be abandoned to focus instead on 2°C or any other higher warming level. All the risks outlined in the above sections are an emphatic call to minimize overshoot, both in absolute magnitude and in duration. New projects to expand fossil fuel infrastructure, in particular the so-called ‘carbon bombs’ (Kühne et al., Reference Kühne, Bartsch, Tate, Higson and Habet2022), advanced with the blessing of parties to the UNFCCC, are fundamentally incompatible with the Paris Agreement. The insufficient pace of mitigation over the last three decades has been largely due to a widespread political unwillingness to confront fossil fuel interests (Stoddard et al., Reference Stoddard, Anderson, Capstick, Carton, Depledge, Facer, Gough, Hache, Hoolohan, Hultman, Hällström, Kartha, Klinsky, Kuchler, Lövbrand, Nasiritousi, Newell, Peters, Sokona and Williams2021). Fossil fuels appeared for the first time in a decision text at COP26 (‘phasedown unabated coal power’) (UNFCCC, 2021), and was repeated at COP27 with an effort to broaden to the other fossil fuels (UNFCCC, 2022b), supported by 80 governments. The science is clear on the need for fossil fuel phase-out, and a political momentum needs to be built in the lead up to COP28 and beyond. It is also important to recognize that for some developing countries and regions, there is the legitimate concern about energy security and lack of readily available financial resources to implement the needed managed transition (Nsafon et al., Reference Nsafon, Same, Yakub, Chaulagain, Kumar and Huh2023; Walton, Reference Walton2022). The phase-out must advance fastest in the countries more capable of absorbing the short-term economic costs, while in poorer countries the transition will require adequate financial support (Muttitt & Kartha, Reference Muttitt and Kartha2020). It is crucial, moreover, that the phase-out is done in a rapid but orderly fashion: in tandem with the phase-in of renewables (Blondeel et al., Reference Blondeel, Bradshaw, Bridge and Kuzemko2021). This is essential for maintaining current energy use and accommodating growing demand from the Global South, through a just energy transition (Heffron & McCauley, Reference Heffron and McCauley2022).
The decarbonization of energy systems is pivotal also given the energy needs of many ‘novel’ CDR options and their expected role in mitigation and eventual stabilization of global temperature (Singh & Colosi, Reference Singh and Colosi2021; Terlouw et al., Reference Terlouw, Bauer, Rosa and Mazzotti2021). The IPCC scenarios consistent with Paris Agreement goals include a substantial deployment of CDR (IPCC, 2022b, Ch12.3). This underscores the need to address the so-called ‘CDR gap’, through policies that foster the scaling up of these technologies. Closing the CDR gap will require advances in the ‘novel’ CDR methods (Figure 3). Most of the current CDR capability, by far, is nature-based. A/R, in particular, is perhaps the most well-known nature-based CDR option, yet there are concerns regarding durability and MRV robustness. Moreover, concerns are also raised for their impacts on specific ecosystems and communities. For example, while some agroforestry systems can offer valuable co-benefits, less thoughtful tree planting initiatives can have substantial negative impacts on livelihoods and biodiversity (Dobson et al., Reference Dobson, Hopcraft, Mduma, Ogutu, Fryxell, Anderson, Archibald, Lehmann, Poole, Caro, Mulder, Holt, Berger, Rubenstein, Kahumbu, Chidumayo, Milner-Gulland, Schluter, Otto and Sinclair2022; Veldman et al., Reference Veldman, Aleman, Alvarado, Anderson, Archibald, Bond, Boutton, Buchmann, Buisson, Canadell, de Dechoum, Diaz-Toribio, Durigan, Ewel, Fernandes, Fidelis, Fleischman, Good, Griffith and Zaloumis2019). Hence, these interventions should be carefully weighed against robust estimations of carbon sequestration potential gains (Seddon, Reference Seddon2022; Seddon et al., Reference Seddon, Smith, Smith, Key, Chausson, Girardin, House, Srivastava and Turner2021). For international policy discussions regarding nature-based CDR, justice considerations have to be front and center: NbS implemented in the Global South cannot be the strategy for continued emissions in the Global North. The CDR gap has to be closed, with more and better CDR, but these technologies should under no circumstance be part of a narrative to excuse or distract from the primary focus of advancing a managed phase-out of fossil fuels. In fact, the uncertainties regarding sequestration potential of CDR options, their scalability and MRV, should rather accelerate the political impetus to reduce CO2 emissions as rapidly as possible.
The urgency for mitigation is reinforced by the urgency for adaptation, especially in the most vulnerable regions and segments of society. We have highlighted recent work on compound events, which are of concern for adaptation, as they pose a particular threat to food security and livelihoods associated with agriculture and fisheries (Gruber et al., Reference Gruber, Boyd, Frölicher and Vogt2021; Yin et al., Reference Yin, Gentine, Slater, Gu, Pokhrel, Hanasaki, Guo, Xiong and Schlenker2023). The new reality of compound events is not yet widely recognized and incorporated into adaptation strategies, a shortcoming that might lead to maladaptive responses (Simpson et al., Reference Simpson, Williams, Mach, Berrang-Ford, Biesbroek, Haasnoot, Segnon, Campbell, Musah-Surugu and Joe2023). Similarly, accelerated deglaciation affects water availability and the livelihoods of mountain populations, which are often marginalized and highly vulnerable (IPCC, 2022b, CCP5; Singh et al., Reference Singh, Tanvir Hassan, Hassan and Bharti2020). Yet, the urgency for adaptation does not justify top-down impositions on what strategies local communities are to implement. Planned relocation, for example, tends to be resisted when it is not the result of highly inclusive and participatory processes (Farbotko et al., Reference Farbotko, Dun, Thornton, McNamara and McMichael2020; Lund, Reference Lund2021). We highlighted recent advances for the operationalization of a justice lens on adaptation planning and implementation, including specific attention to immobility, whether voluntary or involuntary, in the face of heightened climate risks. Among these advances, LLA (Rahman et al., Reference Rahman, Falzon, Robinson, Kuhl, Westoby, Omukuti, Schipper, McNamara, Resurrección, Mfitumukiza and Nadiruzzaman2023) is hailed as a promising approach conducive to adaptation justice. With climate stabilization still nowhere close on the horizon, adaptation challenges will demand a continuous effort of shifting priorities, requiring monitoring and careful assessment to prevent maladaptation.
Food systems are at the center of most sustainability issues, interacting tightly with the climate, biodiversity, human health, and social justice (Gordon et al., Reference Gordon, Bignet, Crona, Henriksson, Holt, Jonell, Lindahl, Troell, Barthel, Deutsch, Folke, Haider, Rockström and Queiroz2017; Turnhout et al., Reference Turnhout, Duncan, Candel, Maas, Roodhof, DeClerck and Watson2021; Willett et al., Reference Willett, Rockström, Loken, Springmann, Lang, Vermeulen, Garnett, Tilman, DeClerck, Wood, Jonell, Clark, Gordon, Fanzo, Hawkes, Zurayk, Rivera, De Vries, Majele Sibanda and Murray2019). The potential contribution of changes in food systems for climate change mitigation and adaptation have to be balanced with socioeconomic needs (Zurek et al., Reference Zurek, Hebinck and Selomane2022) and the protection of biodiversity (Pörtner et al., Reference Pörtner, Scholes, Agard, Archer, Arneth, Bai, Barnes, Burrows, Chan, Cheung, Diamond, Donatti, Duarte, Eisenhauer, Foden, Gasalla, Hanada, Hickler, Hoegh-Guldberg and Ngo2021), enhancing synergies and minimizing trade-offs. Food system transformations have to be a major part of the solution to the climate emergency, but in order to realize their potential, justice needs to be prioritized here as well. This means: broadly inclusive, highly participatory approaches to planning and implementation, grounded on the local and regional specificities of the socioecological context. Such just food system transformations have to be better integrated into climate financing (IFPRI, 2022) and climate action, in particular as part of NDCs (FOLU, 2022; WWF, 2022).
The most celebrated outcome from COP27 was undoubtedly the agreement on creating a Loss and Damage fund, which will be finalized in COP28. But COP27 clearly showed, once again, the difficulties to set a commitment to phase-out fossil fuels, even though the final document reinforced the need for ‘rapid, deep and sustained reductions in greenhouse gas emissions’ by 2030. At COP28, the negotiation will need to start from that statement toward a clear plan for managed phase-out. The blatant contradiction is that parties to the UNFCCC continue to invest in new infrastructure for fossil fuel extraction and consumption, which will directly lead to higher temperatures and longer overshoot, and consequently ever greater losses and damages. The expectations for COP28 will continue around fossil fuel phasing-out, which is now included in the mandate of several governments going into COP28 (Jones et al., Reference Jones, Verkuijl, Cabré and Piggot2023) and is being championed by the UN Secretary General toward the ‘Acceleration Agenda’ at the Climate Ambition Summit in September (UN Press, 2023). For this negotiation to be successful it will be necessary to also have a meaningful unlocking of climate financing in support of just transitions in developing countries.
Looking back at the previous six editions of the ‘10 New Insights in Climate Science’, some areas of stronger emphasis can be identified. A signature of the report has been the understanding of the climate as one among several interacting Earth system domains, and particularly emphasizing the linkages with the biosphere and the implications for the stability and resilience of the planet. The report has regularly paid attention to the emerging science on climate tipping elements, stressing the need to consider more seriously the risks and uncertainties associated with these high-impact destabilizing phenomena. We have been pleased to notice that, in recent years, these issues have gained much more prominence in public debate and climate policy discussions. Regarding the impacts of climate change, our focus has been stronger on health, food, and water security, and extreme events. Different dimensions of human mobility (migration, displacement, immobility) have been highlighted throughout the years, reflecting an increasingly nuanced messaging on this sensitive topic. Different kinds of mitigation strategies (technical, nature-based, and behavioral) have been featured, as well as policy options to deal with government and market failures around fossil fuels (e.g. elimination of subsidies, and implementation of carbon pricing mechanisms). The ‘10 New Insights’ report has also emphasized different ways in which climate change has exacerbated socioeconomic inequalities and stressed the importance of inclusive decision-making for effective and just climate action. The reports have also included reflections on the implications for the climate system and climate action of major developments on the world stage, such as COVID in 2020 and 2021, and the Russian invasion of Ukraine in 2022.
In the most recent editions of the series, we have increasingly spotlighted research on socioeconomic, cultural, and political dimensions of adaptation (and loss and damage), and the political economy barriers to effective and just climate action. We have synthesized scientific knowledge to counter misguided narratives (e.g. ‘endless adaptation’ and ‘readiness of CDR’), to prevent further delay on decisive action to minimize GHG emissions. The ‘10 New Insights’ will continue to provide timely and accessible updates from across the diverse research areas on climate change. As the IPCC begins reconstituting itself for the 7th Assessment, the mission of the ‘10 New Insights’ is to contribute to raising the ‘voice of science’ in the remaining years of this crucial decade for climate action.
Supplementary material
The supplementary material for this article can be found at https://doi.org/10.1017/sus.2023.25.
Acknowledgments
A special thanks to Elsa Wikander and Jerker Lokrantz from Azote for their excellent work on Figures 1–10.
Author contributions
W. B., M. B., J. G. C., D. C., H. A. C., K. L. E., S. F., S. L., A. M., C. O., N. S. O., Å. P., J. Roc., R. R., J. Roy., L. F. S., P. S., Y. S., D. S., and R. S. constituted to the editorial board, conceiving and designing the study, selecting the insights to be highlighted, and providing initial guidance on the outlines. M. B., J. Roy., and D. Osp. led the overall writing. Investigations and writing for each insight: F. L., L. M. P., N. J. S., N. W., and K. Z. (insight 1); P. A., G. M., G. S., and G. T. (insight 2), O. G., Z. H., G. F. N., and J. P. (insight 3); A. B., M. F.-M., N. G., J. H., P. K. P., and A. Ram. (insight 4); D. Obu., T. O., and Y.-J. S. (insight 5); T. L. F., L. J. H., C. Ray., V. T., J. Y., and J. Z. (insight 6); D. R. R., M. H., A. A., and A. P. (insight 7); C. F., B. M., M. P., T. A. S., L. T., and K. G. (insight 8), E. R. C., S. Huq., M. L. P. J., S. J., B. O., and S. E. W. (insight 9); and A. H., V. S., O. S., and S. M. (insight 10). Additional investigation and writing, as well as coordination for each insight: S. Heb. (insight 1), S. S. (insight 2), A. Red. (insights 3 and 6), M. M. (insight 4), T. W. (insight 5), C. Rev. (insight 7), P. M. (insight 8), G. B. S. (insight 9), and N. K. (insight 10). D. Osp. and C. E. coordinated the overall process.
Funding statement
This work was supported by: FORMAS, a Swedish Research Council for Sustainable Development (D. Osp. and W. B.: grant 2021-00273), European Union's Horizon 2020 ERC StG, ForExD (A. B.: grant agreement no. 101039567), Australian National Environmental Science Program – Climate Systems Hub (J. G. C.), Australian Research Council (C. F.: FT210100512), European Research Council (M. F.-M.: StG-2022-101076740, project STOIKOS; and J. H.: StG-2022-101077209, project OceanPeak), Ramón y Cajal fellowship (M. F.-M.: RYC2021-031511-I), Swiss National Science Foundation (T. L. F.: PP00P2_198897), European Union's Horizon 2020 research and innovation program (N. G.: grant 821003, project 4C; and G. F. N.: grant agreement 951542, project GENIE), Ministry for Business, Innovation & Employment of New Zealand (L. J. H.: grant ID: RTVU1906, project Whakahura), Helmholtz Association (J. H.: grant number VH-NG-1301), Ministry of the Environment of Japan (P. K. P.: JPMEERF21S20800, project SII-8), Ministry of Education, Culture, Sports, Science and Technology of Japan (P. K. P.: JPMXD1420318865, project ArCS-II), Research Institute for Humanity and Nature (P. K. P.: NIHU no. 14200133, project Aakash), the German Federal Ministry of Education and Research (J. P.: project CDRSynTra), ERA-Net ForestValue (A. Ram.: project FORECO), National Aeronautics and Space Administration (D. R. R.: grants 80NSSC20K1296 and 80NSSC20K1595), CGIAR (V. S.: NEXUS Gains Initiative), Norway's International Climate and Forest Initiative (NICFI) (V. S.: grant no. SG_SECR_055_00 I), World Resource Institute (V. S.: grant SG_SECR_055_00 I), Bilateral program from the Japan Society for the Promotion of Science (G. T.: grant no. JPJSBP120203502 and JPJSBP120229922), and Helmholtz Initiative and Networking Fund (J. Z.: grant VH-NG-1537, young investigator group COMPOUNDX).
Competing interests
None.
Research transparency and reproducibility
All potential additional resources such as anonymized data and protocols (if not referenced in the manuscript or provided in the Supplementary material) can be requested via e-mail to the corresponding author.