Introduction
Terahertz communication is a hot topic for its potential to be applied in the next generation of wireless technology, considering the dramatically increased needs of the higher data rate and the extremely broad spectral available bandwidth in the terahertz region. Currently the carrier frequency at over 275 GHz was not allocated for any exclusive application, hence the research with the use of carrier frequencies at around 300 GHz has become very active [Reference Nagatsuma, Hisatake, Fujita, Pham, Tsuruda, Kuwano and Terada1–Reference Taggart and Kumar10]. It is not only because of its wide bandwidth of several tens of GHz makes, but also due to the rapid development of electronic and photonic devices. Most of all, it is possible to reach to the millstone of 100 Gbit/s wireless communication which can fill in the gap between the optical and wireless links.
The highest single-channel data rate achieved with an error-free condition, i.e., a bit error rate (BER) of less than 1011, is 50 Gbit/s with the on–off keying (OOK) modulation using a photonics-based broadband transmitter and a direct diode-detection receiver at 300 GHz [Reference Nagatsuma, Hisatake, Fujita, Pham, Tsuruda, Kuwano and Terada1]. However, due to the hardware limitations on bandwidth and/or sensitivity, it is difficult to further improve the data rate without any significant breakthrough on current devices. In this case, multi-level modulation schemes such as phase-shift keying (PSK), quadrature amplitude modulation (QAM), etc. have been introduced in many testbeds [Reference Song, Member, Kim and Ajito5–Reference Jia, Jacobsen, Galili, Morioka, Zibar, Oxenlowe, Pang, Ozolins, Yu, Hu, Yu, Guan, Da Ros and Popov7]. The highest single-channel data rate with a forward-error-correction (FEC)-limited condition, e.g., BER of less than 10−3, exceeds 100 Gbit/s with 16 QAM modulation and powerful digital signal processing (DSP) techniques [Reference Jia, Jacobsen, Galili, Morioka, Zibar, Oxenlowe, Pang, Ozolins, Yu, Hu, Yu, Guan, Da Ros and Popov7]. Although the excellent data rate has been presented with such off-line processing, it is difficult to apply for the practical real-time communication due to the insufficient processing speed and huge cost. While for the real-time communication, the phase noise of the carrier and/or local oscillator (LO) signal is a critical factor that determines the BER for these multi-level modulation systems [Reference Nagatsuma, Fujita, Yasuda, Kanai, Hisatake, Fujiwara and Kani2, Reference Shao, Shams, Anandarajah, Fice, Renaud, van Dijk, Seeds and Barry8–Reference Taggart and Kumar10]. The optical frequency comb generator (OFCG) has been efficiently employed to stabilize both the frequency and phase in the transmitter compared with simple laser pair [Reference Nagatsuma, Hisatake, Fujita, Pham, Tsuruda, Kuwano and Terada1, Reference Nagatsuma, Fujita, Yasuda, Kanai, Hisatake, Fujiwara and Kani2, Reference Ducournau, Pavanello, Zaknoune, Yoshimizu, Hisatake, Lampin, Peytavit and Nagatsuma11]. However, it is no longer effective for high-level modulation with a carrier frequency at 300-GHz band, since the signal generation scheme is essentially a frequency multiplication, in which the phase noise increases quadratically with the harmonic number [Reference Nagatsuma, Fujita, Yasuda, Kanai, Hisatake, Fujiwara and Kani2].
Against such a background, ultra-low phase noise of a photonic source has been urgently required. One of the most popular low phase noise and narrow linewidth photonic sources is based on the spectral narrowing effect of the stimulated Brillouin scattering (SBS) phenomenon in a fiber cavity [Reference Debut, Randoux and Zemmouri12–Reference Li, Rolland, Iwamoto, Kuse, Fermann and Nagatsuma17]. It is demonstrated in [Reference Li, Rolland, Iwamoto, Kuse, Fermann and Nagatsuma17] that a low phase noise of −90 dBc/Hz at 10 kHz offset can be achieved at 300 GHz. In addition, the phase noise of the generated electrical signal does not scale up as the output frequency increases, since tuning the optical wavelength of one of the CW lasers does not affect its phase noise. Moreover, compared with the OFCG-based THz source, a better carrier to noise ratio (C/N) can be achieved due to significantly higher power efficiency. Although applications of SBS-based photonic sources in wireless communications have been reported at 40 GHz [Reference Dennis, Gross, Clark, Novak and Waterhouse15] and 60 GHz [Reference Nanzer, Callahan, Dennis, Clark, Novak and Waterhouse16], there is no study on the implementation in the 300-GHz-band communications.
In this paper, the phase noise issue against photonic-based sources is briefly summarized together with the introduction of a recently developed SBS-based photonic source [Reference Li, Rolland, Iwamoto, Kuse, Fermann and Nagatsuma17]. The superiority of the low phase noise source is demonstrated by comparing with the OFCG-based source via the 300-GHz-band wireless communication experiments with quadrature phase-shift keying (QPSK) modulation. Its advantages are evaluated carefully with the phase noise measurement results and the transmission characteristics at 300 GHz.
Photonic-based THz sources and phase noise
The photonic-based THz sources are full of potential for realizing high-speed wireless link. Compared with the electronic-based sources, the fiber-optical communication techniques make it much easier to obtain a high modulation index with optical-to-THz conversion using photo-mixing [Reference Nagatsuma, Hisatake, Fujita, Pham, Tsuruda, Kuwano and Terada1, Reference Ishibashi, Muramoto, Yoshimatsu and Ito18]. The THz signals can be generated by launching two optical signals at different frequencies f 1 and f 2 into a uni-traveling-carrier photodiode (UTC-PD) as shown in Fig. 1(a), generating a THz signal whose frequency is equal to the difference of those of the two optical waves, i.e., f 1 − f 2 [Reference Ishibashi, Muramoto, Yoshimatsu and Ito18]. Consequently, the stability of the laser sources will transfer to the THz region after photo-detection, ultimately limiting the performance of the photonic-based THz sources.

Fig. 1. The schematic diagram of (a) free-running laser pair and (b) OFCG-based source. PM, phase modulator; OBPF, optical band-pass filter.
Free-running laser pair
As the most straightforward approach, two continuous-wave (CW) lasers operating at different optical frequencies can be directly injected into an UTC-PD to generate THz signals, as shown in Fig. 1(a). In this case, the carrier frequency of THz signal is unstable due to the relative frequency fluctuation between the two independent seeding lasers. The problem of such unstable carrier frequency can be circumvented by deploying envelope detection at the receiver side, allowing a 40 Gbit/s error-free communication [Reference Nagatsuma, Hisatake, Fujita, Pham, Tsuruda, Kuwano and Terada1]. Escalating to multi-level data modulation schemes can be extremely difficult as it requires a coherent LO at the receiver. Sophisticated DSP techniques, which are indispensable in demodulating the received signal with FEC to mitigate the poor stability in both frequency and phase, are deployed in realizing a 100 Gbit/s communication demonstration with a multi-level modulation scheme at 400 GHz using free-running laser pair [Reference Yu, Asif, Piels, Zibar, Galili, Morioka, Jepsen and Oxenlowe6]. However, it is difficult to achieve real-time communication with such complicated DSP, better photonic source and a coherent LO are necessary.
Optical frequency comb generator (OFCG)
In order to eliminate the relative frequency fluctuation between the two lasers, OFCG technique that generates optical signals at different harmonics with a single laser was introduced for photomixing, as shown in Fig. 1(b). By modulating a single-wavelength laser at an optical frequency of f 0 with an electrical driving signal at frequency f s using electro-optical modulators, symmetric higher optical harmonics separating by f s are generated. By extracting two phase correlated optical tones at frequencies f 1 and f 2 with a spacing of 2n f s using an optical band-pass filter, frequency-controllable terahertz signal can be obtained after subsequent optical-to-Terahertz conversion. Compared with the laser pair method, OFCG offers significantly improved carrier frequency stability, enabling real-time data transmission without off-line DSP [Reference Nagatsuma, Hisatake, Fujita, Pham, Tsuruda, Kuwano and Terada1, Reference Nagatsuma, Fujita, Yasuda, Kanai, Hisatake, Fujiwara and Kani2, Reference Jia, Jacobsen, Galili, Morioka, Zibar, Oxenlowe, Pang, Ozolins, Yu, Hu, Yu, Guan, Da Ros and Popov7]. However, the modulation scheme was still limited to OOK and BPSK because of the insufficient carrier quality, as OFCG is fundamentally an optical version of frequency multiplication that the phase noise increases dramatically at higher optical harmonics.
Phase noise for high-level modulation
Phase information is not necessary for OOK and not dominant for the BPSK scheme, while it is critical for the QPSK scheme and higher level modulation. I–Q constellations shown in Fig. 2 illustrate the significance of carrier stability. When the carrier signal is free of noise, all the received samples will be concentrated at a point, in contrast to the case when phase noise is present, the received signal will smear along a certain amplitude. If the signal constantly shifts into the other quadrant it is equivalent to the communication error [Reference Taggart and Kumar10]. It is noticeable from Fig. 2 that for QPSK modulation, the signal is easier to shift into other quadrants compared with BPSK, indicating the necessity of lower phase noise for preventing errors in higher level modulation.

Fig. 2. I–Q constellation of BPSK and QPSK modulation: (a) noise-free BPSK modulation; (b) BPSK modulation with phase noise; (c) noise-free QPSK modulation; and (d) QPSK modulation with phase noise.
As mentioned previously, the phase noise of the generated THz signal utilizing the OFCG method increases quadratically with the harmonic number n. This implies that with a fixed driving frequency, phase noise of the generated THz signal will increases at higher carrier frequency due to the need for higher harmonics. In addition, the optical power of each comb line decreases when the harmonic number increases, leading to a decreased optical carrier-to-noise ratio. Due to this very reason, our previous wireless communication record using QPSK cannot reach its bandwidth limitation at error-free condition without the support of powerful on-line DSP processing [Reference Nagatsuma, Fujita, Yasuda, Kanai, Hisatake, Fujiwara and Kani2]. Hence, it is crucial to find a photonic source that delivers reduced phase noise at the transmitter side.
Stimulated Brillouin scattering (SBS) source
Stimulated Brillouin scattering
SBS in an optical fiber cavity has been widely used to produce narrow linewidth lasers [Reference Debut, Randoux and Zemmouri12–Reference Chen, Wang, Wang, Luo and Meng14]. SBS is an optical phenomenon in which a SBS beam known as Stokes wave whose frequency is down-shifted from that of a strong pump is generated in the opposite propagation direction to that of the pump as shown in Fig. 3. The down-shifted frequency depends on the wavelength and propagation medium, which in our case is ~10 GHz in the 1550 nm wavelength region in standard optical fiber. By launching a strong CW pump into an optical fiber cavity, a Brillouin gain bandwidth is invoked to select one of the resonant modes of the fiber cavity having a much narrower linewidth compared with that of the pump laser. In this way, the system is lasing at the selected resonant mode with higher beam quality.

Fig. 3. Diagrammatic sketch of the SBS phenomenon in optical cavity.
By pumping the same optical fiber cavity using two CW lasers, two narrow-linewidth optical signals with high coherence (since they are principally two different modes of the same cavity) are generated. Shining these optical waves onto a photo-detector is capable of producing THz signals with much improved phase noise and frequency stability. Moreover, as there's not any variation of frequency multiplication involved in changing the output frequency, the scaling property of the phase noise is removed using the SBS-based source, as confirmed in [Reference Li, Rolland, Iwamoto, Kuse, Fermann and Nagatsuma17]. This is considered to be a key advantage as a THz source.
System configuration
Together with the IMRA Inc., an SBS-based source was developed for 300-GHz band wireless communication. The schematic block diagram of the SBS-based source is illustrated in Fig. 4(a), and the photo of the experimental setup is shown in Fig. 4(b). First, two lasers independently generate different optical pumps, f 1 and f 2, with a difference of around 300 GHz. The optical power launched into the Brillouin cavity is ~10 mW, similar to what standard microwave photonic setup uses. Then, the combined optical signals are injected into the Brillouin fiber cavity through an erbium-doped fiber amplifier (EDFA) and a circulator. The fiber cavity consists of a 90:10 coupler and a spool of fiber which is partially glued to a piezoelectric transducer (PZT). The frequencies of two lasers are locked to two different cavity modes, mf 0 and nf 0 (f 0 is the free-spectral range of the fiber cavity and m, n are two positive integers), through the standard Pound–Drever–Hall locking technique, producing two line-width narrowed laser signals at $f_1^{\rm ^{\prime}} = {m}^{\prime}f_0$ and $f_2^{\rm ^{\prime}} = {n}^{\prime}f_0$
, respectively. After optical to electrical conversion by an UTC-PD, low phase noise THz carrier is generated. The PZT is used to phase-lock the frequency of the generated THz signal to an external reference, which is necessary for long-term measurements such as the phase-noise measurement introduced later. However, this locking stage (including an extra thermoelectric temperature controller which is not shown in Fig. 4(b)) was not deployed for communication experiments, as the powerful on-line DSP is sufficient to tackle the minor carrier frequency drift in the time scale of the communication experiment. More detailed parameters of the system and the long-term frequency locking technique are reported in [Reference Li, Rolland, Iwamoto, Kuse, Fermann and Nagatsuma17].

Fig. 4. The proposed SBS-based source: (a) schematic diagram, the dashed rectangular indicates the frequency locking to external reference. PM, phase modulator; EDFA, erbium-doped fiber amplifier; VOA, variable optical attenuator; SA, spectrum analyzer; PID, proportional-integral-differential controller; PZT, piezoelectric transducer; PD, photodiode. (b) Experimental configuration.
Evaluation of SBS-based source
Many related works for evaluating of SBS-based sources have been reported in microwave and millimeter-wave regions [Reference Dennis, Gross, Clark, Novak and Waterhouse15–Reference Li, Rolland, Iwamoto, Kuse, Fermann and Nagatsuma17], while it has been difficult to perform the evaluation at higher frequencies for the limitation of sources and detectors.
In this work, the phase noise at 300 GHz was measured after the down-conversion with a subharmonic mixer (SHM) and an LO signal from the synthesizer and multiplier as schematically shown in Fig. 5(a). The optical spectrum and radio frequency (RF) spectrum without signal modulation is shown in Figs 5(b) and 5(c) respectively. Due to the superior performance of the SBS-based source, a narrow carrier frequency with ~1 kHz bandwidth was achieved at 299.93 GHz.
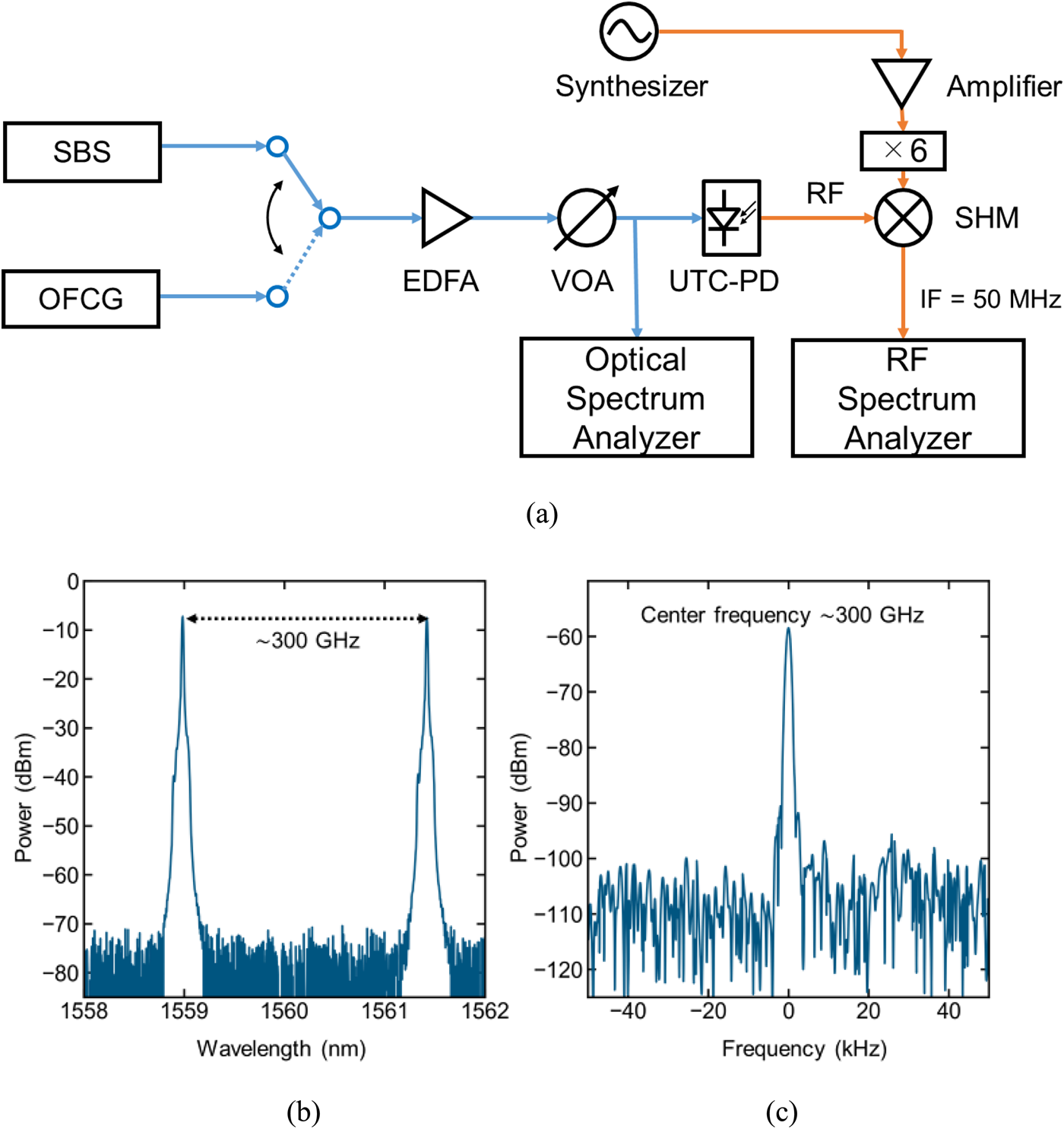
Fig. 5. The experimental setup for phase noise measurement at 300 GHz. (a) Schematic diagram, EDFA: erbium-doped fiber amplifier, VOA, variable optical attenuator; SA, spectrum analyzer. (b) Optical spectrum of the generated two Brillouin laser output when the two pump CW lasers are locked to the fiber cavity. (c) RF spectrum of the generated carrier signal by UTC-PD.
In order to evaluate the phase noise of the SBS-based source, the phase noise was measured with the experimental setup shown in Fig. 5(a) and compared with that of the OFCG-based source [Reference Li, Rolland, Iwamoto, Kuse, Fermann and Nagatsuma17]. As is shown in Fig. 6, red and blue lines indicate the measured phase noise of SBS and OFCG-based sources, respectively, while the yellow line indicates the phase noise of the frequency-multiplied (×12) signals from the synthesizer (25 GHz), which depicts the lower limit of the phase noise in the measurement setup. The phase noise is as low as −90 dBc/Hz at 10 kHz offset for both SBS and OFCG-based sources. It is notably observed that the phase noise below 500 Hz is greatly reduced with the SBS-based source, and it almost overlapped with the phase noise from the synthesizer above 10 kHz frequency offset. Although the SBS-based source has higher phase noise than the OFCG-based source from 500 Hz to 10 kHz since the feedback bandwidth of the locking module is 1 kHz, which means that anything below 1 kHz is determined by the microwave signal using as the reference, the effect of this frequency range is not dominant considering the integrated phase noise along the whole axis of Fig. 6.

Fig. 6. The phase noise measurement at 300 GHz; red line indicates the SBS-based source; blue line indicates the OFCG-based source and the yellow line indicates the phase noise from the synthesizer.
Communication experiment and discussion
In order to study the effect of reduced phase noise of 300-GHz signal generation, we have performed communication experiment using the SBS-based source in the transmitter. Although we face the similar phase noise problem in the receiver, where the SHM pumped by the frequency-multiplied signal source is used, it is fair to compare its performance with the OFCG-based source which was commonly used in our previous research.
Figure 7 shows a schematic diagram of the QPSK communication experiment. Both SBS and OFCG-based sources were applied for comparison, the receiver configuration remained the same. The output of the source was amplified by an EDFA, and was divided into two paths by an optical coupler. Then, an optical band-pass filter (OPBF) was used on each side to obtain high C/N optical sub-carriers with 300-GHz frequency difference. The baseband I/Q signals from the pulse-pattern generator were modulated to one of the optical sub-carriers. The modulated and unmodulated signals were combined with an optical coupler, and were amplified by another EDFA. In order to reduce the amplified spontaneous emission (ASE) noise, another OPBF was used before the 90:10 couplers. Ten percent of the amplified signal was input to an optical spectrum analyzer, while 90% of the signal was input to the UTC-PD to generate the 300-GHz signal. The 300-GHz wave was transmitted and received via a pair of horn-antennas with 10-mm distance without using optical lens. The distance was slightly modified to reach the maximum receiving power and prevent from the effect of standing waves.

Fig. 7. The schematic diagram of the experimental setup. EDFA, erbium-doped fiber amplifier; PPG, pulse-pattern generator; OBPF, optical band-pass filter; OSA, optical spectrum analyzer; VOA, variable optical attenuator.
As for the receiver, an SHM was used for heterodyne detection. The 147.5-GHz LO signal was generated by using a ×6 frequency multiplier. Hence, the 5-GHz IF signal could be acquired, and it was amplified by 30 dB before input into the real-time oscilloscope (Keysight DSAZ504A).
In order to evaluate and compare the two sources, transmission experiments were performed at 5 Gbaud. After the QPSK modulation, optical spectra of both sources were measured as shown in Fig. 8. C/N of the SBS-based source is about 8 dB higher than that of the OFCG-based source. The main reason is that only two optical frequencies are selected from more than 12 combs in the case of OFCG while most of the powers are filtered off, which degrades the C/N. To the contrary, the pump energy was almost exclusively converted to the generated signals in the SBS-based scheme, supporting a much higher power efficiency.

Fig. 8. Comparison on the spectra of the two sources. Red: SBS-based source, blue: OFCG-based source.
BERs were estimated from the error vector magnitude values, which were measured by using the DSP function installed in the commercial real-time oscilloscope. It should be noticed that since the BERs were estimated within a short-terms (less than 160 nanosecond) with our real-time oscilloscope, and the DSP demodulation via the oscilloscope automatically applies frequency tracking, frequency stabilization through phase-locking to an external reference was not mandatory in our experiment and hence benched.
A comparison of BER characteristics of two sources is shown in Fig. 9. It can be seen that the FEC-limit BER (<2 × 10−3) can be achieved at 5 Gbaud (10 Gbit/s) with both sources, and that the performance of the SBS-based source is better than the OFCG-based source. It can be observed that less output power was required to reach FEC-limit with the SBS-based source (−45 dBm) compared with that for the OFCG-based source (−42 dBm). Furthermore, up to 15-Gbaud transmission succeeded with the SBS-based source at the FEC-limit with an output power of only −35 dBm, which cannot be achieved with the OFCG-based source.

Fig. 9. Comparison of the BER characteristics; circle marks: 5 Gbaud with SBS-based source; hollow circle marks with dash line: 5 Gbaud with OFCG-based source; cross marks: 10 Gbaud with OFCG-based source; square marks: 15 Gbaud with SBS-based source.
I–Q constellations in the FEC-limit BER condition are shown in Fig. 10. The slightly elliptical distribution of data points observed in the OFCG case is an indication of higher phase noise. Such distribution is absent in the case of the SBS-based source, validating the significant improvement in phase noise. Moreover, the SBS-based source managed to achieve the same level of constellation concentration despite a ~3 dB lower output power. Amplitude noise, which determines the concentration, of the SBS-based system is expected to be further suppressed by packaging all the fiber-optic components (Fig. 4(a)) into a single box, insulating the environmental perturbations such as acoustic noise and temperature fluctuations

Fig. 10. I–Q constellation recorded in FEC-limit BER condition for 5-Gbaud transmission. (a) OFCG-based source and (b) SBS-based source.
In addition, expected to be originated from light locking enforcement and environmental perturbation, optical signals from the SBS-based source vanishes occasionally. For the protection of the UTC-PD from a dramatic change of photocurrent, the communication experiment was carried out with extremely low output power compared to our previous experiments [Reference Nagatsuma, Fujita, Yasuda, Kanai, Hisatake, Fujiwara and Kani2]. Therefore, the main aim of this work is to enlighten the advantage of the SBS-based source. Further collaborating experiments towards a high-speed wireless link with an improved SBS source is under preparation.
In the end, it must be mentioned that the performance of the current 300-GHz-band wireless link is still limited by the phase noise contributed in the receiver side, which was also observed in the phase noise measurement (Fig. 6). By introducing a fundamental mixer pumped by photonic LO with the SBS-based source, BER characteristics are expected to be dramatically improved.
Conclusion
In this paper, a low phase noise photonic source based on SBS in an optical fiber cavity has been introduced to 300-GHz-band wireless communication for the first time. It has been experimentally demonstrated that the SBS-based source offers lower phase noise and higher C/N compared with the OFCG-based source. An instrumentation-limited phase noise of −90 dBc/Hz at 10 kHz offset has been achieved at 300 GHz. From the QPSK transmission experiments, the SBS-based source has exhibited better performance than the OFCG-based source with respect to BER and constellation at 5 Gbaud. Fifteen-Gbaud data transmission was also achieved with the SBS-based source at the FEC-limit condition. It is worth to mention that the experiments were carried out with very low output power from UTC-PD, which enlighten the potential for a higher data rate record with a properly optimized SBS-based source.
On top of the hardware issue of the SBS-based source that will be fixed in the next round of the experiment, another key issue that needs to be solved is the phase noise introduced at the receiver. The fundamental mixer pumped by the photonic LO signal using the SBS-based source will be an ultimate solution for higher order multi-level modulation schemes such as 32 QAM and beyond. In addition, an application to higher frequency bands such as 600-GHz, where a wider atmospheric window is available, is expected to better showcase the non-scaling phase noise characteristic of the SBS-based photonic THz source.
Acknowledgement
Part of this work is supported by the Ministry of Education, Science, Sports and Culture, through the Grant-in-Aid for Scientific Research program (16H04350), National Natural Science Foundation of China (NSFC) (61801014) and Japan Science and Technology Agency (JST) for funding Industry-Academia Collaborative R&D Program.
Li Yi is an assistant professor at Graduate School of Engineering Science of Osaka university, Japan. Li Yi received his M.E. and Ph.D. degrees in environmental studies from the Graduate School of Environmental Studies, Tohoku University, Japan, in 2014 and 2017, respectively. Then he has been a researcher of AIST (Japan) until 2018. He is currently an assistant professor at Graduate School of Engineering Science of Osaka university, Japan.
His current research interests are photonic based millimeter/terahertz waves devices and applications, which include wireless communication and signal processing techniques and imaging and sensing applications.
Kenta Iwamoto was born in Osaka, Japan, in 1994. He received the B.S. and M.S. degrees in Engineering Science from Osaka University, Osaka, Japan in 2017 and 2019, respectively. He was engaged in the development of the THz wireless communication system based on photonics (in 2019, he joined Hamamatsu Photonics K.K., Shizuoka, Japan).
Takumi Yamamoto was born in Osaka, Japan, in 1996. He received the B.E. degree in engineering science from Osaka University, Osaka, Japan, in 2018. In 2018, he entered the Graduate School of Engineering Science, Osaka University. His current research interests include THz wireless communication.
Fumiya Ayano was born in Okayama, Japan, on October 20, 1996. He received his B.S degree in Engineering Science from Osaka University, Osaka, Japan in 2019. In 2019 he entered the Graduated School of Engineering Science, Osaka University. He is engaged in research on the THz wireless communications system.
Dr. Rolland graduated from Université de Rennes 1 in 2013. He then spent 4 years working as a postdoc at the National Institute of Standards and Technology in Boulder, USA and the National Physical Laboratory in Teddington, UK on optical frequency metrology and optical frequency combs. In 2017, he joined IMRA America, Inc. Boulder Research Labs where his work focus on frequency combs, microcombs, and millimeter-wave and THz generation.
Naoya Kuse received the B.S., M.S., and Ph.D. degrees in Applied Physics from University of Tokyo, Tokyo, Japan, in 2008, 2010, and 2013, respectively. He joined IMRA America Inc., in 2013. He is currently an associate professor in Tokushima University. His interest includes optical frequency combs.
Yihan Li received the Ph.D. degree in electrical engineering from Purdue University, West Lafayette, IN, USA, in 2015. He is currently an Associate Professor of the School of Electrical and Information Engineering at Beihang University, Beijing, China. He was with IMRA America as a Laser Research Scientist in the Boulder Research Lab. He joined Beihang as an Associated Professor in 2018. His research interests include ultrafast optics, optical frequency comb, microwave photonics and photonic wireless of millimeter-wave and terahertz.
Tadao Nagatsuma received B.S., M.S., and Ph.D. degrees in electronic engineering from Kyushu University, Fukuoka, Japan, in 1981, 1983, and 1986, respectively. From 1986 to 2007, he was with Nippon Telegraph and Telephone Corporation (NTT), Atsugi, Kanagawa, Japan. Since 2007, he has been with Osaka University, where he is a Professor at Graduate School of Engineering Science. His research interests include millimeter-wave and terahertz photonics and their applications to wireless communications, sensing, and measurement. He is a Fellow of the IEEE, a Fellow of the Institute of Electronics, Information and Communication Engineers (IEICE), Japan, and a Fellow of the Electromagnetics Academy. He currently serves as an Associate Editor of the IEEE Photonics Technology Letters and the IEEE Trans. Terahertz Science and Technology, and a Vice President of the IEICE and the Terahertz Systems Consortium.