Introduction
The co- or post-translational addition of glycans to proteins takes various forms in all kingdoms of life (Varki, Reference Varki2011); amongst the most common is N-glycosylation, by which asparagine residues are modified. In eukaryotes, most commonly a Glc3Man9GlcNAc2 precursor is transferred from dolichol to proteins in the endoplasmic reticulum (Aebi, Reference Aebi2013); however, some protists utilise shorter precursors or even do not N-glycosylate at all (Samuelson et al., Reference Samuelson, Banerjee, Magnelli, Cui, Kelleher, Gilmore and Robbins2005). The fates of protein-linked N-glycans are varied and depend on the types of glycosidases and glycosyltransferases expressed in the Golgi apparatus. It is this variability that makes glycan analysis a challenge, as so many possibilities occur by which N-glycans are trimmed and then built up again.
Other than the first steps in the endoplasmic reticulum, the final size and form of N-glycans differ between protists, fungi, plants and animals (whether invertebrate or vertebrate), although some modifications are found in more than one of these groups of organisms. Unlike plants whose N-glycomes are similar from mosses through to Arabidopsis, there is high variability between non-vertebrate eukaryotes (Schiller et al., Reference Schiller, Hykollari, Yan, Paschinger and Wilson2012). Here, we will concentrate on primarily structural aspects of invertebrate N-glycans, not only due to the parasitological relevance (as many invertebrates are either hosts, vectors or themselves parasites), but also because only recently have mass spectrometric analyses revealed a previously unrealised range of modifications, some of which are shared with O- and lipid-linked glycans. A few years ago, one would probably have read that invertebrates only produce oligomannosidic (Man5–9GlcNAc2) and paucimannosidic (Man1–4GlcNAc2Fuc0–2) N-glycans (Williams et al., Reference Williams, Wormald, Dwek, Rademacher, Parker and Roberts1991); this may be due to insensitive methods and low expectations, but it is now known that even complete glycomes of some mammalian cell types are dominated by oligomannosidic forms present within the secretory pathway (Hamouda et al., Reference Hamouda, Kaup, Ullah, Berger, Sandig, Tauber and Blanchard2014).
Oligomannosidic N-glycans
Even within the glycans containing primarily mannose residues (hence oligomannosidic or high mannose), there is variation arising from the different orders of processing by so-called class I α1,2-mannosidases, also in parasitic metazoa. Most eukaryotes have multiple forms of these α1,2-mannosidases (Wilson, Reference Wilson2012), which also include enzymes known as EDEMs (ER degradation-enhancing α-mannosidases) acting as part of the quality control pathway in the endoplasmic reticulum. The result is that there are multiple isomers of oligomannosidic structures (e.g. three isomers of glycans with the composition Man8GlcNAc2; Fig. 1) just depending on which mannosidase acts first on particular terminal mannose residues; the final product is the ‘Golgi’ isomer of Man5GlcNAc2. These structures can be differentiated by, e.g. RP-HPLC in combination with MS/MS and thus it is only appropriate to annotate specific isomers based on such information; for instance, a Hex8HexNAc2 structure could also be Glc1Man7GlcNAc2 and not necessarily one of three typical forms of Man8GlcNAc2. Oligomannosidic glycans may also be the ‘final’ processed forms in the cases where protein folding prevents a specific glycosylation site from being accessible to enzymes in the Golgi apparatus (Thaysen-Andersen and Packer, Reference Thaysen-Andersen and Packer2012). On the basis of the universality of oligomannosidic glycans in metazoa, it is not surprising that these glycans have been observed in a wide range of invertebrates including trematodes, nematodes, molluscs and insects.

Fig. 1. Simplified biosynthetic scheme for N-linked glycans in animals. Starting with the Glc3Man9GlcNAc2 precursor, various glycosidases result in different isomers of oligomannosidic glycans with the maximal degree of processing by class I mannosidases yielding Man5GlcNAc2. This is the substrate for N-acetylglucosaminyltransferase I (GlcNAc-TI) which generates a ‘hybrid’ structure which can be further modified by the action of Golgi mannosidase II, GlcNAc-TII and Golgi hexosaminidase. The maximum number of antennae (three or four) depends on the presence of GlcNAc-TIV and GlcNAc-TV; example hybrid, pseudohybrid, paucimannosidic and tri-/tetra-antennary glycans are shown as known from various model, host, vector or parasitic invertebrates. For simplicity, fucosylation and other modifications are not included. Glycans are depicted according to the Standard Nomenclature for Glycans (see also box).
Hybrid and pseudohybrid N-glycans
The classical ‘hybrid’ structure is a ‘Golgi-type’ Man5GlcNAc2 modified on the ‘lower’ α1,3-mannose by β1,2-specific N-acetylglucosaminyltransferase I (GlcNAc-TI; encoded by the mammalian MGAT1 gene and its homologues in multicellular eukaryotes, including parasitic invertebrates) to yield Man5GlcNAc3 (Fig. 1) which may be the substrate for further modification. This is a key intermediate in N-glycan biosynthesis (Fig. 1) in terms of the routes to processed structures as well as in biological terms (Schachter, Reference Schachter2010), as ablation of this gene results in large glycomic shifts as well as a range of phenotypes (Shi et al., Reference Shi, Tan and Schachter2006; Sarkar et al., Reference Sarkar, Iliadi, Leventis, Schachter and Boulianne2010; Yan et al., Reference Yan, Wang, Schachter, Jin, Wilson and Paschinger2018b), most dramatically, the embryonic lethal phenotype in mammals (Ioffe and Stanley, Reference Ioffe and Stanley1994; Metzler et al., Reference Metzler, Gertz, Sarkar, Schachter, Schrader and Marth1994).
Once GlcNAc-TI has acted, Golgi mannosidase II will remove one or two of the ‘upper’ mannose residues and also represents a potential biosynthetic bottleneck (Paschinger et al., Reference Paschinger, Hackl, Gutternigg, Kretschmer-Lubich, Stemmer, Jantsch, Lochnit and Wilson2006). If there is no transfer by GlcNAc-TII thereafter to the α1,6-mannose, then the glycan remains in a hybrid state (i.e. sharing aspects of oligomannosidic and complex structures); also even if GlcNAc-TIV (β1,4-specific) modifies the α1,3-mannose, then the glycan is still classified as being hybrid (Kornfeld and Kornfeld, Reference Kornfeld and Kornfeld1985). The lower arm β1,2- and β1,4-GlcNAc residues on hybrid glycans can be modified in different ways; elongation by β1,4-N-acetylgalactosamine and β1,3- or β1,4-galactose are known in insects, molluscs, nematodes and trematodes, whether these be host or parasitic organisms (Nyame et al., Reference Nyame, Smith, Damian and Cummings1989; Kurz et al., Reference Kurz, Jin, Hykollari, Gregorich, Giomarelli, Vasta, Wilson and Paschinger2013, Reference Kurz, Aoki, Jin, Karlsson, Tiemeyer, Wilson and Paschinger2015; Martini et al., Reference Martini, Eckmair, Neupert, Štefanić, Jin, Garg, Jiménez-Castells, Hykollari, Yan, Venco, Varón Silva, Wilson and Paschinger2019; Smit et al., Reference Smit, van Diepen, Nguyen, Wuhrer, Hoffmann, Deelder and Hokke2015). If however, GlcNAc-TII acts and then the ‘lower’ arm β1,2-GlcNAc, transferred by GlcNAc-TI, is removed by a Golgi hexosaminidase such as fdl (fused lobes) in insects or HEX-2 in nematodes (Gutternigg et al., Reference Gutternigg, Kretschmer-Lubich, Paschinger, Rendić, Hader, Geier, Ranftl, Jantsch, Lochnit and Wilson2007b; Geisler and Jarvis, Reference Geisler and Jarvis2012), then the resulting glycans can be referred to as ‘pseudohybrid’. Such structures are also found in protist parasites lacking GlcNAc-TI, but having GlcNAc-TII-like enzyme activities (Paschinger et al., Reference Paschinger, Hykollari, Razzazi-Fazeli, Greenwell, Leitsch, Walochnik and Wilson2012b; Damerow et al., Reference Damerow, Rodrigues, Wu, Güther, Mehlert and Ferguson2014). The core of hybrid glycans in animals can also be modified, most commonly by α1,6-fucose.
Paucimannosidic N-glycans
The term ‘paucimannosidic’ glycans was introduced to cover those glycans which have been processed serially by GlcNAc-TI, Golgi mannosidase II and a Golgi hexosaminidase to result in Man3–4GlcNAc2 (Gutternigg et al., Reference Gutternigg, Kretschmer-Lubich, Paschinger, Rendić, Hader, Geier, Ranftl, Jantsch, Lochnit and Wilson2007b). Such structures are well known in invertebrates and plants, but also occur due to the action of acidic glycosidases on glycoproteins in the secretory granules in some mammalian cells (Loke et al., Reference Loke, Ostergaard, Heegaard, Packer and Thaysen-Andersen2017). A significant portion of paucimannosidic glycans are core fucosylated and carry the ‘mammalian-like’ α1,6-fucose and the ‘plant-like’ α1,3-fucose either alone or in combination on the reducing-terminal (proximal) GlcNAc of the core region of the N-glycan as found first on bee venom glycoproteins (Kubelka et al., Reference Kubelka, Altmann, Staudacher, Tretter, März, Hård, Kamerling and Vliegenthart1993). In nematodes, the second (distal) GlcNAc can also be modified (Haslam et al., Reference Haslam, Coles, Munn, Smith, Smith, Morris and Dell1996; Hanneman et al., Reference Hanneman, Rosa, Ashline and Reinhold2006).
Both proximal core α1,3-fucose and substitution of the β-mannose by β1,2-xylose (see Fig. 2 for example structures) are immunogenic in mammals and antibodies raised against plant and invertebrate glycoproteins often recognise these epitopes, the best known example of which is anti-horseradish peroxidase (anti-HRP); both structural elements are epitopes for IgE or IgG in parasite-infected animals or children as well as in individuals allergic to plant pollen, food or insect venom, although the clinical relevance is controversial (van Die et al., Reference van Die, Gomord, Kooyman, van der Berg, Cummings and Vervelde1999; Altmann, Reference Altmann2007; Paschinger et al., Reference Paschinger, Rendić and Wilson2009; Brzezicka et al., Reference Brzezicka, Echeverria, Serna, van Diepen, Hokke and Reichardt2015; Amoah et al., Reference Amoah, Asuming-Brempong, Obeng, Versteeg, Larbi, Aryeetey, Platts-Mills, Mari, Brzezicka, Gyan, Mutocheluh, Boakye, Reichardt, van Ree, Hokke, van Diepen and Yazdanbakhsh2018). While core α1,3-fucose is widespread in invertebrates, xylosylation of N-glycans is known from gastropods and, in a stage-specific manner, Schistosoma spp. (Khoo et al., Reference Khoo, Chatterjee, Caulfield, Morris and Dell1997; Gutternigg et al., Reference Gutternigg, Bürgmayr, Pöltl, Rudolf and Staudacher2007a; Lehr et al., Reference Lehr, Frank, Natsuka, Geyer, Beuerlein, Doenhoff, Hase and Geyer2010; Smit et al., Reference Smit, van Diepen, Nguyen, Wuhrer, Hoffmann, Deelder and Hokke2015). This is interesting as some gastropods (specifically snails such as Biomphalaria glabrata) are intermediate hosts for schistosomes. The activities of core-modifying fucosyl- and xylosyltransferases have been detected in extracts of various species, but only for core α1,3/α1,6-difucosylation have relevant genes been identified and recombinant forms of the enzymes characterised (Fabini et al., Reference Fabini, Freilinger, Altmann and Wilson2001; Paschinger et al., Reference Paschinger, Staudacher, Stemmer, Fabini and Wilson2005; Rendić et al., Reference Rendić, Klaudiny, Stemmer, Schmidt, Paschinger and Wilson2007; Kurz et al., Reference Kurz, King, Dinglasan, Paschinger and Wilson2016).
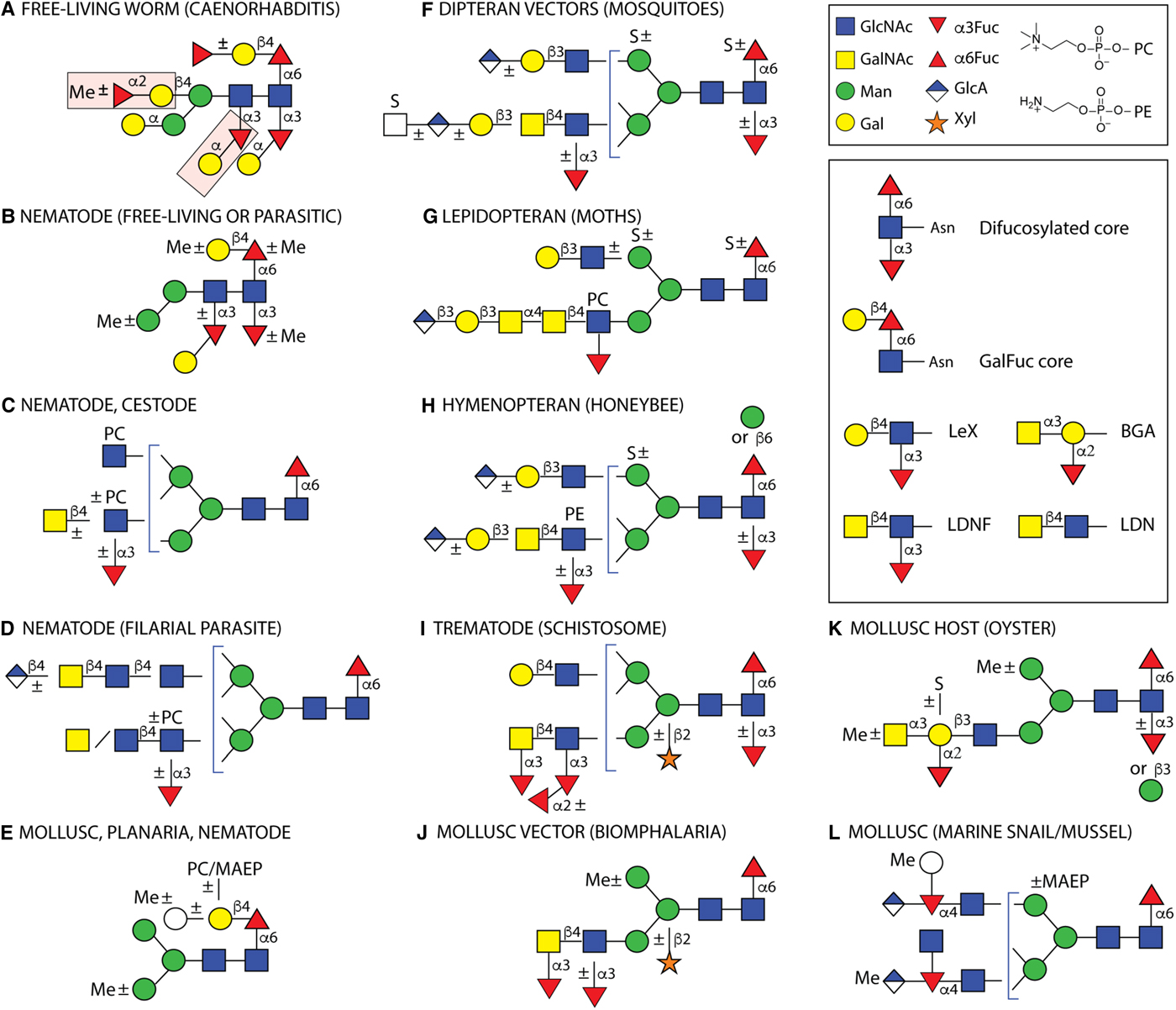
Fig. 2. Example N-glycans from invertebrates. Structures are depicted from either parasitic or free-living organisms, whereby some of the latter are hosts or vectors for parasites. Some types of structures are species- or class-specific, but others are found in more than one phylum. Only a non-exhaustive selection of core and antennal epitopes is shown in the inset: core difucosylation, core ‘GalFuc’, Lewis X (LeX), fucosylated and non-fucosylated LacdiNAc (LDN) and blood group A (BGA). (A) The bisecting and distal core modifications found in the free-living C. elegans are indicated by pink boxes; (B) free-living C. elegans, the necromenic P. pacificus and the parasites H. contortus, H. polygyrus and O. dentatum express di- and/or tri-fucosylated cores with species-specific galactosylation and methylation; (C) varying antennal modifications are found in all nematodes as well as the cestodes E. granulosus and T. crassiceps, (D) while filarial species have up to four long antennae including D. immitis, which has in addition glucuronylated structures; (E) galactosylated core fucose (GalFuc) is found in many invertebrates, sometimes in substituted form; (F, G and H) selected complex glycans from larvae of different insect phyla; (I) selected S. mansoni N-glycan modifications which are partly stage-specific; (J, K and L) selected gastropod and bivalve glycans, including those of Crassostrea virginica, B. glabrata, Volvarina rubella and Mytilus edulis. Note that some modifications, such as core β-mannosylation, are at low abundance in the relevant glycomes. Glycans are depicted according to the Standard Nomenclature for Glycans; undefined hexoses/N-acetylhexosamines are shown as white circles/squares. Me, methyl; MAEP, N-methyl-aminoethylphosphonate; PC, phosphorylcholine; PE, phosphoethanolamine (2-aminoethylphosphate); S, sulphate. Broken lines,±or brackets indicate structure-, species- or stage-dependent variations in these elements.
Modified N-glycan cores
In addition to fucosylation and xylosylation, some invertebrates attach further monosaccharide units to the basic paucimannosidic core. Recently joining the list of core modifications alongside galactosylation of core α1,6-fucose (‘GalFuc’), first detected in squid and then in keyhole limpet, planaria and nematodes (Takahashi et al., Reference Takahashi, Masuda, Hiraki, Yoshihara, Huang, Khoo and Kato2004; Wuhrer et al., Reference Wuhrer, Robijn, Koeleman, Balog, Geyer, Deelder and Hokke2004; Titz et al., Reference Titz, Butschi, Henrissat, Fan, Hennet, Razzazi-Fazeli, Hengartner, Wilson, Künzler and Aebi2009; Paschinger et al., Reference Paschinger, Razzazi-Fazeli, Furukawa and Wilson2011; Subramanian et al., Reference Subramanian, Babu, Palakodeti and Subramanian2018), are α-galactosylation of the proximal and distal core α1,3-fucose residues (Yan et al., Reference Yan, Vanbeselaere, Jin, Blaukopf, Wols, Wilson and Paschinger2018a), elongation of the GalFuc unit by galactose (Wuhrer et al., Reference Wuhrer, Robijn, Koeleman, Balog, Geyer, Deelder and Hokke2004; Subramanian et al., Reference Subramanian, Babu, Palakodeti and Subramanian2018), fucose (Yan et al., Reference Yan, Jin, Wilson and Paschinger2015b), phosphorylcholine or methylaminoethylphosphonate (Eckmair et al., Reference Eckmair, Jin, Abed-Navandi and Paschinger2016), β-mannosylation of the proximal GlcNAc (Eckmair et al., Reference Eckmair, Jin, Abed-Navandi and Paschinger2016; Hykollari et al., Reference Hykollari, Malzl, Eckmair, Vanbeselaere, Scheidl, Jin, Karlsson, Wilson and Paschinger2018) and the galactosylation of the core β-mannose to form a bisected structure, which can also be modified by methylated or nonmethylated fucose (Yan et al., Reference Yan, Brecker, Jin, Titz, Dragosits, Karlsson, Jantsch, Wilson and Paschinger2015a) (Fig. 2). While the latter bisecting modifications have only been found in the non-parasitic nematode Caenorhabditis elegans, the zwitterionic modifications of the GalFuc have been detected uniquely in a marine gastropod; however, galactosylation of the proximal α1,6-fucose and distal α1,3-fucose residues has also been found in the parasitic nematodes Oesophagostomum dentatum and Haemonchus contortus (Paschinger and Wilson, Reference Paschinger and Wilson2015; Sutov, Reference Sutov2016; Jiménez-Castells et al., Reference Jiménez-Castells, Vanbeselaere, Kohlhuber, Ruttkowski, Joachim and Paschinger2017).
Some of the reason for the apparent restriction in what is found might be methodological. For instance, the presence of α-galactose on the proximal α1,3-fucose was only detected in C. elegans when using hydrazine or the newly-developed PNGase Ar enzyme to release the N-glycans, whereby the maximal degree of core fucosylation in this worm (five fucoses) was only found after hydrazinolysis (Yan et al., Reference Yan, Vanbeselaere, Jin, Blaukopf, Wols, Wilson and Paschinger2018a). Only in the case of O. dentatum can we say that these modifications are absent, since hydrazinolysis was also performed with samples from this organism and MS/MS did not reveal any glycan with the relevant fragmentation pattern (Jiménez-Castells et al., Reference Jiménez-Castells, Vanbeselaere, Kohlhuber, Ruttkowski, Joachim and Paschinger2017). On the other hand, H. contortus glycans were only ever analysed after ‘classical’ PNGase F and A digestion and so it can only be speculated as to whether it shares more complex cores with C. elegans.
The enzymatic basis for only some of these modifications is known. Three core-modifying α-fucosyltransferases (FUT-1, FUT-6 and FUT-8) are known from C. elegans as is the α1,6-fucose-modifying GALT-1 galactosyltransferase from the same organism (Paschinger et al., Reference Paschinger, Rendić, Lochnit, Jantsch and Wilson2004, Reference Paschinger, Staudacher, Stemmer, Fabini and Wilson2005; Titz et al., Reference Titz, Butschi, Henrissat, Fan, Hennet, Razzazi-Fazeli, Hengartner, Wilson, Künzler and Aebi2009; Yan et al., Reference Yan, Serna, Reichardt, Paschinger and Wilson2013). The in vitro activity data is complemented by glycomic studies on mutants showing the absence of the relevant epitopes (Butschi et al., Reference Butschi, Titz, Wälti, Olieric, Paschinger, Nöbauer, Guo, Seeberger, Wilson, Aebi, Hengartner and Künzler2010; Yan et al., Reference Yan, Jin, Wilson and Paschinger2015b). Some of these glyco-mutants have altered susceptibility to nematoxic fungal lectins (Butschi et al., Reference Butschi, Titz, Wälti, Olieric, Paschinger, Nöbauer, Guo, Seeberger, Wilson, Aebi, Hengartner and Künzler2010; Schubert et al., Reference Schubert, Bleuler-Martinez, Butschi, Walti, Egloff, Stutz, Yan, Wilson, Hengartner, Aebi, Allain and Künzler2012), which are also toxic to H. contortus (Heim et al., Reference Heim, Hertzberg, Butschi, Bleuler-Martinez, Aebi, Deplazes, Künzler and Stefanic2015). For all the other modifications around the core, e.g. the addition of various α-galactose residues or of bisecting β-galactose in C. elegans we have no clues as to which enzymes may be responsible. The same lack of knowledge applies to β-mannosylation of the proximal core GlcNAc in molluscs and insects.
Complex N-glycans
The definition ‘complex N-glycan’ is based on the knowledge of mammalian glycosylation and refers to glycans with at least one GlcNAc modifying both α-mannose residues of the trimannosyl core. Thus, both GlcNAc-TI and -TII (MGAT1 and MGAT2) have acted and these can be supplemented by GlcNAc-TIV, GlcNAc-TV and in some species ‘GlcNAc-TVI’ (Schachter, Reference Schachter1986). The other common N-acetylglucosaminyltransferase, GlcNAc-TIII, is a bisecting enzyme found in vertebrates. The result of the action of these various enzymes (see Fig. 1) is the various bi-, tri- and tetra-antennary glycans (even penta-antennary in birds and fish), which are well known from the serum glycomes of mammals.
It may come as a surprise that even relatively primitive animals have tri- or tetra-antennary N-glycans as found in Hydra, molluscs, insects and nematodes (Kang et al., Reference Kang, Cummings and McCall1993; Morelle et al., Reference Morelle, Haslam, Olivier, Appleton, Morris and Dell2000; Kurz et al., Reference Kurz, Jin, Hykollari, Gregorich, Giomarelli, Vasta, Wilson and Paschinger2013, Reference Kurz, Aoki, Jin, Karlsson, Tiemeyer, Wilson and Paschinger2015; Sahadevan et al., Reference Sahadevan, Antonopoulos, Haslam, Dell, Ramaswamy and Babu2014; Eckmair et al., Reference Eckmair, Jin, Abed-Navandi and Paschinger2016). The exact nature of the tri-antennary glycans varies, as GlcNAc-TIV products occur in molluscs and insects (Kurz et al., Reference Kurz, Jin, Hykollari, Gregorich, Giomarelli, Vasta, Wilson and Paschinger2013, Reference Kurz, Aoki, Jin, Karlsson, Tiemeyer, Wilson and Paschinger2015), but GlcNAc-TV acts in glycan biosynthesis in a number of nematodes such as C. elegans and Pristionchus pacificus (Yan et al., Reference Yan, Wilson and Paschinger2015c). Other nematodes, though, do have both GlcNAc-TIV and -TV homologues and so can have up to four branches on their N-glycans as found in filarial species or in Trichinella (Haslam et al., Reference Haslam, Houston, Harnett, Reason, Morris and Dell1999; Kang et al., Reference Kang, Cummings and McCall1993; Morelle et al., Reference Morelle, Haslam, Olivier, Appleton, Morris and Dell2000; Martini et al., Reference Martini, Eckmair, Neupert, Štefanić, Jin, Garg, Jiménez-Castells, Hykollari, Yan, Venco, Varón Silva, Wilson and Paschinger2019).
Amongst trematodes, triantennary glycans have been long established to exist in S. mansoni males (Nyame et al., Reference Nyame, Smith, Damian and Cummings1989); it has also been suggested that up to four branches may also be on N-glycans of S. mansoni eggs or in Opisthorchis viverrini (Talabnin et al., Reference Talabnin, Aoki, Saichua, Wongkham, Kaewkes, Boons, Sripa and Tiemeyer2013; Smit et al., Reference Smit, van Diepen, Nguyen, Wuhrer, Hoffmann, Deelder and Hokke2015); however, as in another trematode Fasciola hepatica (McVeigh et al., Reference McVeigh, Cwiklinski, Garcia-Campos, Mulcahy, O'Neill, Maule and Dalton2018), BLAST searching of the available genomes only shows an obvious GlcNAc-TV homologue and none of GlcNAc-TIV (unpublished data). However, only for C. elegans GlcNAc-TI and GlcNAc-TII is there in vitro evidence from recombinant enzymes to verify the predicted activities (Chen et al., Reference Chen, Tan, Reinhold, Spence and Schachter2002), while C. elegans GlcNAc-TV has been shown to complement a relevant Chinese hamster ovary mutant cell line in terms of lectin sensitivity (Warren et al., Reference Warren, Krizius, Roy, Culotti and Dennis2002); the activity of an invertebrate GlcNAc-TIV has still to be proven.
Subsequent to the initial transfer of up to four non-reducing terminal GlcNAc residues, further elongation events can occur and these are extremely variable and, in non-vertebrates, include substitutions with β1,3-galactose, β1,4-N-acetylgalactosamine, α1,4-N-acetylgalactosamine or fucose as well as anionic, zwitterionic or methyl groups (Fig. 2). The typical mammalian form of galactosylation (β1,4) is not so widespread in lower animals in general, but it can, e.g. be found in Schistosoma spp. (Khoo et al., Reference Khoo, Chatterjee, Caulfield, Morris and Dell1997; Smit et al., Reference Smit, van Diepen, Nguyen, Wuhrer, Hoffmann, Deelder and Hokke2015); it can only be distinguished from β1,3-galactosylation by use of specific galactosidases and, if amounts allow, by NMR spectroscopy or GC-MS methods. Antennal GlcNAc residues modified with β1,3Gal or even β1,3Galβ1,4GalNAc are found on N-glycans from, e.g. mosquitoes acting as intermediate hosts for parasites and viruses (Kurz et al., Reference Kurz, Aoki, Jin, Karlsson, Tiemeyer, Wilson and Paschinger2015) and β1,3-Gal is also found in the oyster Crassostrea virginica, which is a host for the Perkinsus marinus protist parasite (Kurz et al., Reference Kurz, Jin, Hykollari, Gregorich, Giomarelli, Vasta, Wilson and Paschinger2013). On the other hand, GalNAcβ1,4GlcNAc (LacdiNAc) is a known motif from various insects and nematodes (see also the section on fucosylated antennae below) and a stage-specific bias in its expression is known from trematode parasites (Talabnin et al., Reference Talabnin, Aoki, Saichua, Wongkham, Kaewkes, Boons, Sripa and Tiemeyer2013; Smit et al., Reference Smit, van Diepen, Nguyen, Wuhrer, Hoffmann, Deelder and Hokke2015). Longer chito-based (GlcNAcβ1,4GlcNAc) antennae are a feature of filarial nematodes as well as of H. contortus and O. dentatum (Haslam et al., Reference Haslam, Houston, Harnett, Reason, Morris and Dell1999; Sutov, Reference Sutov2016; Jiménez-Castells et al., Reference Jiménez-Castells, Vanbeselaere, Kohlhuber, Ruttkowski, Joachim and Paschinger2017; Martini et al., Reference Martini, Eckmair, Neupert, Štefanić, Jin, Garg, Jiménez-Castells, Hykollari, Yan, Venco, Varón Silva, Wilson and Paschinger2019).
For some of these terminal modifications, the relevant enzymes have been identified and characterised in recombinant form, such as β1,4-N-acetylgalactosaminyltransferases from C. elegans and Trichoplusia ni, a β1,3-galactosyltransferase from the honeybee and an α1,4-N-acetylgalactosaminyltransferase from Drosophila (Kawar et al., Reference Kawar, van Die and Cummings2002; Mucha et al., Reference Mucha, Domlatil, Lochnit, Rendić, Paschinger, Hinterkörner, Hofinger, Kosma and Wilson2004; Vadaie and Jarvis, Reference Vadaie and Jarvis2004; Ichimiya et al., Reference Ichimiya, Maeda, Sakamura, Kanazawa, Nishihara and Kimura2015). However, although some relevant enzyme activities have been detected in crude extracts, the identities of relevant genes in parasites are yet to be established.
Antennally fucosylated N-glycans
Fucose as a deoxyhexose rather than a standard hexose may well, due to its chemical properties, be pre-destined to act as a recognition element. Indeed, fucose is the basis for mammalian histo-blood group antigens such as ABO and Lewis motifs (Fig. 2). Fucosylated LacNAc (Lex) and LacdiNAc (LDNF) epitopes are well known from S. mansoni (Khoo et al., Reference Khoo, Chatterjee, Caulfield, Morris and Dell1997; Wuhrer et al., Reference Wuhrer, Koeleman, Deelder and Hokke2006; Smit et al., Reference Smit, van Diepen, Nguyen, Wuhrer, Hoffmann, Deelder and Hokke2015) and may contribute to the lectin-dependent immunomodulatory activity of secreted schistosome proteins (Wilbers et al., Reference Wilbers, Westerhof, van Noort, Obieglo, Driessen, Everts, Gringhuis, Schramm, Goverse, Smant, Bakker, Smits, Yazdanbakhsh, Schots and Hokke2017). Also, some nematodes (e.g. Dictyocaulus viviparus, Trichuris suis or H. contortus) and insects (e.g. the honeybee) express these epitopes (Kubelka et al., Reference Kubelka, Altmann, Staudacher, Tretter, März, Hård, Kamerling and Vliegenthart1993; Haslam et al., Reference Haslam, Coles, Morris and Dell2000; Paschinger and Wilson, Reference Paschinger and Wilson2015; Wilson and Paschinger, Reference Wilson and Paschinger2016), while fucosylated chito-oligomers are a feature of the antennae of some N-glycans from Dirofilaria immitis (Martini et al., Reference Martini, Eckmair, Neupert, Štefanić, Jin, Garg, Jiménez-Castells, Hykollari, Yan, Venco, Varón Silva, Wilson and Paschinger2019) and Fucα1,3GlcNAc as a terminal motif is also known from the cestode Taenia crassiceps (Lee et al., Reference Lee, Dissanayake, Panico, Morris, Dell and Haslam2005). Less familiar may be the occurrence of blood group A on oyster glycans (Kurz et al., Reference Kurz, Jin, Hykollari, Gregorich, Giomarelli, Vasta, Wilson and Paschinger2013), which are probable ligands for noroviruses in the marine environment, but which are also recognised by the oyster's own galectins (Feng et al., Reference Feng, Ghosh, Amin, Giomarelli, Shridhar, Banerjee, Fernandez-Robledo, Bianchet, Wang, Wilson and Vasta2013). Interestingly, though, these galectins also mediate entry of P. marinus into oyster haemocytes, despite the apparent lack of blood group antigens on the parasite.
Generally, fucose on glycan antennae is unsubstituted, but branched fucose (i.e. disubstituted) is known in some molluscs (Zhou et al., Reference Zhou, Hanneman, Chasteen and Reinhold2013; Eckmair et al., Reference Eckmair, Jin, Abed-Navandi and Paschinger2016) and fucosylated fucose (Fucα1,2Fucα1,3) occurs in S. mansoni (Jang-Lee et al., Reference Jang-Lee, Curwen, Ashton, Tissot, Mathieson, Panico, Dell, Wilson and Haslam2007; Smit et al., Reference Smit, van Diepen, Nguyen, Wuhrer, Hoffmann, Deelder and Hokke2015) (Fig. 2). Interestingly, the various fucosylated antennal modifications of S. mansoni are epitopes for various natural and monoclonal antibodies (van Remoortere et al., Reference van Remoortere, Hokke, van Dam, van Die, Deelder and van den Eijnden2000; van Diepen et al., Reference van Diepen, Smit, van Egmond, Kabatereine, Pinot de Moira, Dunne and Hokke2012) and may mediate interactions of parasitic proteins with cells of the host immune system (Meevissen et al., Reference Meevissen, Driessen, Smits, Versteegh, van Vliet, van Kooyk, Schramm, Deelder, Haas, Yazdanbakhsh and Hokke2012). The schistosome genome encodes a number of fucosyltransferases, but only one has proven enzymatic activity in recombinant form, specifically as a LeX synthase (Mickum et al., Reference Mickum, Rojsajjakul, Yu and Cummings2016b). Other defined invertebrate Lewis-type fucosyltransferases include the FucTC from the honeybee and a mosquito (Kurz et al., Reference Kurz, King, Dinglasan, Paschinger and Wilson2016; Rendić et al., Reference Rendić, Klaudiny, Stemmer, Schmidt, Paschinger and Wilson2007).
Methylated N-glycans
Substitution of glycans by methyl groups is known in bacteria, plants and invertebrates. In the case of N-glycans from mollusc, planaria and free-living or parasitic nematodes, examples include methylation of mannose, fucose, galactose and N-acetylgalactosamine residues (van Kuik et al., Reference van Kuik, Sijbesma, Kamerling, Vliegenthart and Wood1986, Reference Van Kuik, Sijbesma, Kamerling, Vliegenthart and Wood1987b; Gutternigg et al., Reference Gutternigg, Bürgmayr, Pöltl, Rudolf and Staudacher2007a; Paschinger et al., Reference Paschinger, Razzazi-Fazeli, Furukawa and Wilson2011; Kurz et al., Reference Kurz, Jin, Hykollari, Gregorich, Giomarelli, Vasta, Wilson and Paschinger2013; Hewitson et al., Reference Hewitson, Nguyen, van Diepen, Smit, Koeleman, McSorley, Murray, Maizels and Hokke2016; Jiménez-Castells et al., Reference Jiménez-Castells, Vanbeselaere, Kohlhuber, Ruttkowski, Joachim and Paschinger2017; Yan et al., Reference Yan, Vanbeselaere, Jin, Blaukopf, Wols, Wilson and Paschinger2018a) (Fig. 2). If analysing the glycans using standard permethylation conditions, such natural methyl groups are lost; thus, perdeuteromethylation has to be employed (Wohlschlager et al., Reference Wohlschlager, Butschi, Grassi, Sutov, Gauss, Hauck, Schmieder, Knobel, Titz, Dell, Haslam, Hengartner, Aebi and Künzler2014). For standard exoglycosidase sequencing, methylation normally prevents removal of a residue, but the methylated GalNAc on oyster glycans could be removed with chicken α-N-acetylgalactosaminidase (Kurz et al., Reference Kurz, Jin, Hykollari, Gregorich, Giomarelli, Vasta, Wilson and Paschinger2013), while methylated α1,2- or α1,3-fucose residues on nematode glycans can be partially or fully released by hydrofluoric acid treatment (Yan et al., Reference Yan, Vanbeselaere, Jin, Blaukopf, Wols, Wilson and Paschinger2018a). The type of methylation can also vary within a species, as methylation of mannose was more common in male O. dentatum parasites as opposed to the methylfucose residues found in the female (Jiménez-Castells et al., Reference Jiménez-Castells, Vanbeselaere, Kohlhuber, Ruttkowski, Joachim and Paschinger2017).
Glucuronylated and sialylated N-glycans
By separating neutral from anionic glycans early in the analyses, we have been able to find glucuronic acid on the termini of N-glycans from a number of species, including mosquitoes, moths and the honeybee, as well as a marine snail (Kurz et al., Reference Kurz, Jin, Hykollari, Gregorich, Giomarelli, Vasta, Wilson and Paschinger2013, Reference Kurz, Aoki, Jin, Karlsson, Tiemeyer, Wilson and Paschinger2015; Eckmair et al., Reference Eckmair, Jin, Abed-Navandi and Paschinger2016; Stanton et al., Reference Stanton, Hykollari, Eckmair, Malzl, Dragosits, Palmberger, Wang, Wilson and Paschinger2017; Hykollari et al., Reference Hykollari, Malzl, Eckmair, Vanbeselaere, Scheidl, Jin, Karlsson, Wilson and Paschinger2018) (Fig. 2). Like methylated hexose residues, the presence of glucuronic acid results in a mass increment of 176 Da, but GlcA-containing glycans can be detected by negative mode mass spectrometry (Hykollari et al., Reference Hykollari, Paschinger, Eckmair and Wilson2017). Using permethylation, others also detected glucuronic acid on N-glycans of Drosophila (Aoki and Tiemeyer, Reference Aoki and Tiemeyer2010), whereas we have also used glucuronidases to help prove its occurrence on oligosaccharide structures from other insects (Stanton et al., Reference Stanton, Hykollari, Eckmair, Malzl, Dragosits, Palmberger, Wang, Wilson and Paschinger2017; Hykollari et al., Reference Hykollari, Malzl, Eckmair, Vanbeselaere, Scheidl, Jin, Karlsson, Wilson and Paschinger2018). Except for Dirofilaria immitis (Martini et al., Reference Martini, Eckmair, Neupert, Štefanić, Jin, Garg, Jiménez-Castells, Hykollari, Yan, Venco, Varón Silva, Wilson and Paschinger2019), there are no reports to date of GlcA on N-glycans of nematodes or trematodes, but glycosaminoglycan chains and O-glycans from these species do contain this residue (Palaima et al., Reference Palaima, Leymarie, Stroud, Mizanur, Hodgkin, Gravato-Nobre, Costello and Cipollo2010; Vanbeselaere et al., Reference Vanbeselaere, Yan, Joachim, Paschinger and Wilson2018), including the circulating anodic antigen of S. mansoni (Bergwerff et al., Reference Bergwerff, Van Dam, Rotmans, Deelder, Kamerling and Vliegenthart1994).
As glucuronic acid is a major component of glycosaminoglycans and these are known to play roles in host-pathogen interactions (Pinzon-Ortiz et al., Reference Pinzon-Ortiz, Friedman, Esko and Sinnis2001; Armistead et al., Reference Armistead, Wilson, van Kuppevelt and Dinglasan2011), one can speculate that glucuronic acid on N-glycans may be another ligand involved in, e.g. Plasmodium transmission by mosquitoes. The role of glucuronylation of Dirofilaria N-glycans is also unclear. The actual transfer of glucuronic acid to N-glycans has not been proven for any invertebrate glucuronyltransferase, other than for two enzymes of broad specificity from Drosophila (Kim et al., Reference Kim, Tsuchida, Lincecum, Kitagawa, Bernfield and Sugahara2003).
In terms of sialylation, for which there is no hint in most invertebrates, its occurrence in insects has been controversial. Other than mass spectrometric studies on N-glycans from Drosophila embryos (Aoki et al., Reference Aoki, Perlman, Lim, Cantu, Wells and Tiemeyer2007; Frappaolo et al., Reference Frappaolo, Sechi, Kumagai, Robinson, Fraschini, Karimpour-Ghahnavieh, Belloni, Piergentili, Tiemeyer, Tiemeyer and Giansanti2017), there is no firm proof to date for sialylation in any other insect; this is despite genome sequencing typically indicating the presence of one sialyltransferase homologue per insect species, some of which have proven in vitro activities (Koles et al., Reference Koles, Irvine and Panin2004; Kajiura et al., Reference Kajiura, Hamaguchi, Mizushima, Misaki and Fujiyama2015). Higher up the evolutionary tree, however, there is good evidence for sialic acids on the O-glycans of Echinodermata (Miyata et al., Reference Miyata, Sato, Kumita, Toriyama, Vacquier and Kitajima2006). Lectin binding data, although suggestive, is too ambiguous to be considered proof of the presence of sialic acid in unknown glycomes, as the ‘summarised’ specificities of many lectins are probably a simplification, but also contamination must be considered if detecting sialylation in glycans of a parasite derived from a mammalian host.
Sulphated and phosphorylated N-glycans
Another surprisingly widespread anionic modification is sulphate, which results in signals in negative mode mass MS and a Δm/z of 80 mass units. Thereby, for many instruments, sulphate cannot be differentiated from phosphate; however, some very high resolution mass spectrometers can be used to distinguish these. Other proofs include the ionisation of phosphate in both positive and negative mode or the susceptibility of phosphate (and not of sulphate) to hydrofluoric acid or phosphatase treatments (Hykollari et al., Reference Hykollari, Paschinger, Eckmair and Wilson2017). By pre-separating neutral and anionic glycans prior to off-line LC-MS, we have detected sulphate in marine molluscs (including oyster) and in insects (including mosquitoes). On the other hand, standard permethylation procedures will result in loss of sulphated glycans, but modified solid phase extraction methods are compatible with subsequent detection of permethylated sulphated glycans as performed with mosquito or royal jelly N-glycans (Kurz et al., Reference Kurz, Aoki, Jin, Karlsson, Tiemeyer, Wilson and Paschinger2015; Hykollari et al., Reference Hykollari, Malzl, Eckmair, Vanbeselaere, Scheidl, Jin, Karlsson, Wilson and Paschinger2018).
Sulphation of invertebrate N-glycans may occur at different positions, e.g. of mannose or core fucose in arthropods or of galactose as in oyster (van Kuik et al., Reference van Kuik, Breg, Kolsteeg, Kamerling and Vliegenthart1987a; Kurz et al., Reference Kurz, Jin, Hykollari, Gregorich, Giomarelli, Vasta, Wilson and Paschinger2013, Reference Kurz, Aoki, Jin, Karlsson, Tiemeyer, Wilson and Paschinger2015) (Fig. 2), but we have yet to definitely prove sulphation in a parasite. Others have detected phosphorylation of mannose residues in F. hepatica (Ravida et al., Reference Ravida, Aldridge, Driessen, Heus, Hokke and O'Neill2016). The mannose-6-phosphorylation system known for trafficking of lysosomal enzymes in vertebrates is not proven in any invertebrate; strangely, though, a mannose phosphorylation mediated by a homologue of the relevant GlcNAc-1-phosphotransferase enzyme is found in an amoeba (Qian et al., Reference Qian, West and Kornfeld2010). There is no information regarding any N-glycan-modifying sulpho- or phosphotransferase from any invertebrate.
Zwitterionic N-glycans
Phosphodiester and phosphonate modifications such as phosphorylcholine, phosphoethanolamine and aminoethylphosphonate may be familiar to many from bacterial lipopolysaccharides and glycosylphosphatidylinositol anchors or related molecules, but have been reported on a number of invertebrate N-, O- and lipid-linked glycans. While detection of these modifications is incompatible with permethylation procedures, they can all be released with hydrofluoric acid (HF) and so some earlier reports for their presence were based partly on detection of permethylated forms of ‘stripped’ glycans as well as of perdeuteroacetylated structures without HF treatment (Haslam et al., Reference Haslam, Houston, Harnett, Reason, Morris and Dell1999; Morelle et al., Reference Morelle, Haslam, Olivier, Appleton, Morris and Dell2000). However, when conducting more ‘native’ mass spectrometric analyses, phosphorylcholine (PC; Δm/z 165 mass units) ionises very well in positive mode and is a widespread modification of nematode N-glycans (Hanneman et al., Reference Hanneman, Rosa, Ashline and Reinhold2006; Pöltl et al., Reference Pöltl, Kerner, Paschinger and Wilson2007; Paschinger and Wilson, Reference Paschinger and Wilson2015; Hewitson et al., Reference Hewitson, Nguyen, van Diepen, Smit, Koeleman, McSorley, Murray, Maizels and Hokke2016; Wilson and Paschinger, Reference Wilson and Paschinger2016; Jiménez-Castells et al., Reference Jiménez-Castells, Vanbeselaere, Kohlhuber, Ruttkowski, Joachim and Paschinger2017; Martini et al., Reference Martini, Eckmair, Neupert, Štefanić, Jin, Garg, Jiménez-Castells, Hykollari, Yan, Venco, Varón Silva, Wilson and Paschinger2019), but has also been found in a cestode (Echinococcus granulosus) and more recently on moth N-glycans (Paschinger et al., Reference Paschinger, Gonzalez-Sapienza and Wilson2012a; Stanton et al., Reference Stanton, Hykollari, Eckmair, Malzl, Dragosits, Palmberger, Wang, Wilson and Paschinger2017) (Fig. 2).
Phosphoethanolamine (PE; Δm/z 123), aminoethylphosphonate (AEP; Δm/z 107) and methylaminophosphonate (MEAP; Δm/z 121) are detected in both positive and negative modes (Paschinger and Wilson, Reference Paschinger and Wilson2016). PE is found on N-glycans of royal jelly, AEP on those of a locust glycoprotein and MEAP on the antennae and core regions of N-glycans from a marine snail (Hård et al., Reference Hård, Van Doorn, Thomas-Oates, Kamerling and Van der Horst1993; Eckmair et al., Reference Eckmair, Jin, Abed-Navandi and Paschinger2016) (Fig. 2); other reports have shown PC, PE and MEAP on glycolipids or O-glycans of various invertebrates, including Ascaris suum (Hayashi and Matsubara, Reference Hayashi and Matsubara1989; Sugita et al., Reference Sugita, Fujii, Inagaki, Suzuki, Hayata and Hori1992; Lochnit et al., Reference Lochnit, Dennis, Ulmer and Geyer1998; Seppo et al., Reference Seppo, Moreland, Schweingruber and Tiemeyer2000; Maes et al., Reference Maes, Garenaux, Strecker, Leroy, Wieruszeski, Brassart and Guerardel2005; Urai et al., Reference Urai, Nakamura, Uzawa, Baba, Taniguchi, Seki and Ushida2009).
PC and PE are ligands for pentraxins and so binding of Echinococcus Ag5 or of Dirofilaria glycans to C-reactive protein or of royal jelly N-glycans to serum amyloid P have been shown (Paschinger et al., Reference Paschinger, Gonzalez-Sapienza and Wilson2012a; Hykollari et al., Reference Hykollari, Malzl, Eckmair, Vanbeselaere, Scheidl, Jin, Karlsson, Wilson and Paschinger2018; Martini et al., Reference Martini, Eckmair, Neupert, Štefanić, Jin, Garg, Jiménez-Castells, Hykollari, Yan, Venco, Varón Silva, Wilson and Paschinger2019). On the other hand, PC modifications of glycoconjugates are associated with immunomodulation; a well-known example of this being the ES-62 excretory-secretory protein from the filarial worm Acanthocheilonema viteae (Pineda et al., Reference Pineda, Lumb, Harnett and Harnett2014). The biosynthesis of zwitterionic N-glycans remains unresolved, other than a requirement for the prior action of GlcNAc-TI in C. elegans (Houston et al., Reference Houston, Sutharsan, Steiger, Schachter and Harnett2008), but comparisons with pathways in bacteria and fungi may help in the future to decipher the molecular basis for these reactions.
N-glycan arrays
Glycans mediate function when they can be recognised and glycan arrays have become an established method for determining which proteins can bind them. However, other than S. mansoni (van Diepen et al., Reference van Diepen, Smit, van Egmond, Kabatereine, Pinot de Moira, Dunne and Hokke2012; Mickum et al., Reference Mickum, Prasanphanich, Song, Dorabawila, Mandalasi, Lasanajak, Luyai, Secor, Wilkins, Van Die, Smith, Nyame, Cummings and Rivera-Marrero2016a), studies using natural structures are in their relative infancy for invertebrates, but pools or fractions of natural N-glycans from royal jelly, Dirofilaria and C. elegans have been tested recently in an immobilised format with pentraxins, selected antibodies or standard lectins (Hykollari et al., Reference Hykollari, Malzl, Eckmair, Vanbeselaere, Scheidl, Jin, Karlsson, Wilson and Paschinger2018; Jankowska et al., Reference Jankowska, Parsons, Song, Smith, Cummings and Cipollo2018; Martini et al., Reference Martini, Eckmair, Neupert, Štefanić, Jin, Garg, Jiménez-Castells, Hykollari, Yan, Venco, Varón Silva, Wilson and Paschinger2019). The bias in the literature towards schistosome arrays is probably due to a number of factors, such as availability of the various stages of the life-cycle and of monoclonal antibodies as well as three decades of relevant glycomic research. Thus, it has been possible to construct arrays of N-, O- and lipid-linked glycans derived from different stages of the schistosome life-cycle and screen them, e.g. with antibodies or antisera (van Diepen et al., Reference van Diepen, Smit, van Egmond, Kabatereine, Pinot de Moira, Dunne and Hokke2012, Reference van Diepen, van der Plas, Kozak, Royle, Dunne and Hokke2015; Yang et al., Reference Yang, Li, Brzezicka, Reichardt, Wilson, van Diepen and Hokke2017, Reference Yang, van Diepen, Brzezicka, Reichardt and Hokke2018). Otherwise, some anti-helminth antibody responses have been tested against the primarily mammalian array of the Consortium for Functional Glycomics, remodelled glycans or conjugates with shorter saccharides to identify potential protective or diagnostic epitopes (van Stijn et al., Reference van Stijn, van den Broek, Vervelde, Alvarez, Cummings, Tefsen and van Die2009; Aranzamendi et al., Reference Aranzamendi, Tefsen, Jansen, Chiumiento, Bruschi, Kortbeek, Smith, Cummings, Pinelli and Van Die2011; Luyai et al., Reference Luyai, Heimburg-Molinaro, Prasanphanich, Mickum, Lasanajak, Song, Nyame, Wilkins, Rivera-Marrero, Smith, Van Die, Secor and Cummings2014).
Another option is to use chemoenzymatic synthesis to replicate natural glycostructural motifs and so some structures akin or identical to those of schistosomes or nematodes have been prepared, in part with our defined C. elegans FUT-1, FUT-6 and FUT-8 core fucosyltransferases (Yan et al., Reference Yan, Serna, Reichardt, Paschinger and Wilson2013). The resulting synthetic arrays, which can also be studied in parallel to natural arrays, have been probed with, e.g. human lectins, anti-Schistosoma monoclonal antibodies or with the sera of Schistosoma-infected humans or macaques (Brzezicka et al., Reference Brzezicka, Echeverria, Serna, van Diepen, Hokke and Reichardt2015; Yang et al., Reference Yang, Li, Brzezicka, Reichardt, Wilson, van Diepen and Hokke2017, Reference Yang, van Diepen, Brzezicka, Reichardt and Hokke2018; Echeverria et al., Reference Echeverria, Serna, Achilli, Vives, Pham, Thepaut, Hokke, Fieschi and Reichardt2018). Thereby, some detailed insights into recognised structures can be obtained; for instance, antibodies recognising fucosylated antennae may correlate with the stage of parasite infection, while the presence of xylose or the exact antennal N-glycan configuration may have a negative role on lectin binding or be associated with skewed IgG subtype reactivity.
Conclusion
With this brief summary of the different categories of N-glycan modifications in invertebrates, we hope the reader will appreciate the great glycomic variety. Comparing parasitic, non-parasitic and host species is still far from complete; thus, it is still difficult to state whether certain N-glycans or epitopes are themselves hallmarks for parasitism or tropism. It may well be that each parasite has adopted aspects of its ancestors' or its hosts' glycomic capacity in order to fill a specific patho-ecological niche. On the other hand, knowledge about the glycomic status of hosts for recombinant protein production (e.g. insect cell lines) is important before, or may even aid, their use as factories for production of vaccines against parasites. In any case, only carefully performed glycomics can yield the deepest knowledge about invertebrate glycans, including exclusion of host glycans from the analyses, and is a pre-requisite for binding and other functional studies. Here, one challenge is to isolate sufficient natural glycans from parasites or related species or to recreate the structures in vitro. Another is to identify relevant ‘glycozyme’ genes, which will allow more recombinant glycosyltransferases to be used in chemoenzymatic synthesis and, as CRISPR/Cas9-based genetic engineering is beginning to be used in metazoan parasites (Gang et al., Reference Gang, Castelletto, Bryant, Yang, Mancuso, Lopez, Pellegrini and Hallem2017; McVeigh and Maule, Reference McVeigh and Maule2019), enable the switching on/off of certain glycosylation pathways. Indeed, a mix of analytical, biological and chemical tools will certainly prove valuable in the future to not only define the binding partners of specific glycans, but to predict their wider evolutionary occurrence and determine their function in host-parasite interactions.
Author ORCIDs
Katharina Paschinger, 0000-0002-3594-7136; Iain B. H. Wilson, 0000-0001-8996-1518.
Financial support
Work on our laboratory relevant to this review has been funded by the Austrian Science Fund (FWF; grants P21946 and P25058 to K.P. and P23922 and P29466 to I.B.H.W.) and the European Union (Glycopar EU FP7 Marie Curie Initial Training Network; PITN-GA-2013-608295).
Conflict of interest
None.
Ethical standards
Not applicable.