Introduction
Glufosinate is sold commercially as a racemic mixture of d- and l-phosphinothricin but only the l-isomer has herbicidal activity (Beriault et al. Reference Beriault, Horsman and Devine1999). Glufosinate-resistant crops (Liberty Link®) are genetically engineered for phosphinothricin acetyltransferase (pat) expression to metabolize l-phosphinothricin into N-acetyl-l-phosphinothricin, a nonphytotoxic compound (Dröge et al. Reference Dröge, Broer and Pühler1992). To date, there have been only five cases of evolved glufosinate resistance in the world: goosegrass [Eleusine indica (L.) Gaertn.] from Malaysia, rigid ryegrass (Lolium rigidum Gaudin) from Greece, perennial ryegrass [Lolium perenne L. ssp. multiflorum (Lam.) Husnot] from New Zealand, L. perenne ssp. multiflorum from Oregon (USA), and L. perenne ssp. multiflorum from California (USA) (Brunharo et al. Reference Brunharo, Takano, Mallory-Smith, Dayan and Hanson2019; Ghanizadeh et al. Reference Ghanizadeh, Harrington and James2015; Jalaludin et al. Reference Jalaludin, Ngim, Bakar and Alias2010; Karn et al. Reference Karn, Beffa and Jasieniuk2018; Travlos et al. Reference Travlos, Cheimona, De Prado, Jhala, Chachalis and Tani2018). Although glufosinate is an alternative tool to manage multiple herbicide-resistant weeds, it does not translocate well in plants, and weed efficacy is greatly affected by environmental conditions (low light, temperature, and humidity) and plant size (Coetzer et al. Reference Coetzer, Al-Khatib and Loughin2001; Sellers et al. Reference Sellers, Smeda and Johnson2003; Steckel et al. Reference Steckel, Wax, Simmons and Phillips1997). Glufosinate is a fast-acting and broad-spectrum herbicide targeting glutamine synthetase (GS), a key enzyme for amino acid metabolism and photorespiration in plants (Bayer et al. Reference Bayer, Gugel, Hägele, Hagenmaier, Jessipow, König and Zähner1972; Oliveira et al. Reference Oliveira, Brears, Knight, Clark and Coruzzi2002). GS catalyzes the incorporation of ammonia into glutamate to form glutamine (Bernard and Habash Reference Bernard and Habash2009). Glufosinate inhibits GS competing with glutamate for the active site. While GS inhibition leads to ammonia accumulation and changes in amino acid levels, the rapid phytotoxicity results from a massive light-dependent generation of reactive oxygen species (ROS) (Takano et al. Reference Takano, Beffa, Preston, Westra and Dayan2019, Reference Takano, Beffa, Preston, Westra and Dayan2020a).
Fluxomics refers to small molecule fluxes and networks across different metabolic pathways in systems biology of living cells (Winter and Krömer Reference Winter and Krömer2013). Glutamate is an interesting example to study fluxomics, because it occupies a central position in amino acid metabolism. In addition to its role as a substrate for GS, glutamate is also a precursor for proline, arginine, and chlorophyll biosynthesis (Forde and Lea Reference Forde and Lea2007). Protoporphyrinogen oxidase (PPO) is an important enzyme for the chlorophyll pathway, catalyzing the conversion of protoporphyrinogen (protogen) into protoporphyrin (proto) (Lermontova et al. Reference Lermontova, Kruse, Mock and Grimm1997). When PPO is inhibited, protogen leaks out of the chloroplast where the reaction takes place. In the cytoplasm, protogen is converted into proto. Therefore, inhibition of PPO leads to accumulation of proto, the product of the reaction (Lee et al. Reference Lee, Duke and Duke1993; Matringe et al. Reference Matringe, Camadro, Labbe and Scalla1989). Herbicides targeting PPO are also fast acting because proto generates ROS in the presence of light, causing lipid peroxidation (Dayan et al. Reference Dayan, Barker, Bough, Ortiz, Takano, Duke and Moo-Young2019b). Resistance to PPO inhibitors has been reported in 13 species around the world (Heap Reference Heap2020). In a PPO-resistant waterhemp [Amaranthus tuberculatus (Moq.) Sauer] population, a codon deletion (ΔG210) affected herbicide binding to the active site and provided high levels of resistance to lactofen (Dayan et al. Reference Dayan, Daga, Duke, Lee, Tranel and Doerksen2010; Patzoldt et al. Reference Patzoldt, Hager, McCormick and Tranel2006).
Herbicide mixtures are commonly used in agriculture to improve efficacy, increase the spectrum of weed control, and mitigate herbicide resistance (Busi et al. Reference Busi, Powles, Beckie and Renton2019; Zhang et al. Reference Zhang, Hamill and Weaver1995). The combination between two herbicides is synergistic when the combined effect is larger than predicted (Sørensen et al. Reference Sørensen, Cedergreen, Skovgaard and Streibig2007). The fact that glutamate is the precursor for both glutamine and chlorophyll biosynthesis suggests that inhibiting these two pathways at once could lead to catastrophic consequences in plants. We used a fluxomics approach to investigate the fate of glutamate under GS and PPO inhibition. Our hypothesis was that glutamate is diverted to the chlorophyll biosynthesis pathway following GS inhibition. If this is true, glufosinate could enhance the activity of PPO inhibitors by diverting the carbon flow toward proto accumulation in the chlorophyll pathway. Thus, we investigated the interaction between glufosinate and PPO inhibitors, along with the physiological basis for the synergistic effect observed with the herbicide combination.
Material and Methods
Chemical Sources
Glufosinate commercial formulation (Liberty® 280 g L−1) and analytical d, l-glufosinate were provided by Bayer CropScience (Frankfurt, Germany). The PPO inhibitors were purchased from their respective manufacturers: saflufenacil (Sharpen® 297 g L−1, BASF, Raleigh, NC, USA), flumioxazin (Valor® 510 g kg−1, Valent, Walnut Creek, CA, USA), lactofen (Cobra® 240 g L−1, Valent), fomesafen (Reflex® 228 g L−1, Syngenta, Greensboro, NC, USA), pyraflufen (Venue® 20 g L−1, Nichino, Wilmington, DE, USA). Analytical l-amino acids, proto, nitro blue tetrazolium chloride (NBT), potassium phosphate monobasic, sodium azide, 3,3′-diaminobenzidine (DAB) were purchased from Sigma-Aldrich (St Louis, MO, USA) or Fisher Scientific (Waltham, MA, USA).
Plant Growth and Spraying Conditions
Seeds of Palmer amaranth (Amaranthus palmeri S. Watson) were collected in eastern Colorado, and plants were susceptible to both glufosinate and PPO inhibitors. All plant species were grown in 0.3-cm3 pots filled with soil (Sun Gro Horticulture, Agawam, MA, USA). Greenhouse conditions were 25/21 C day/night, 16-h photoperiod with light intensity of 500 μmol m−2 s−1 and 70% relative humidity (RH). For herbicide applications, a commercial chamber track sprayer (DeVries Manufacturing, Hollandale, MN, USA) equipped with an 8002EVS single even, flat-fan nozzle (TeeJet®, Spraying Systems, Denver, CO, USA) calibrated to deliver 187 L ha−1 spray solution at the level of the plant canopy was used.
Levels of Amino Acids and Glufosinate
Plants of A. palmeri (6-leaf stage) were sprayed with 560 g ha−1 glufosinate plus 20 g L−1 ammonium sulfate (AMS). Untreated plants were sprayed with AMS only, and samples were collected at 24 h after treatment (HAT). Leaf tissue (200 mg) was ground in liquid nitrogen with a mortar and pestle. The powder was homogenized with 10 ml methanol-water (75:25 [v/v]) and incubated in ultrasonic bath for 30 min before being centrifuged at 4,000 × g for 10 min. The supernatant (1.5 ml) was filtered through a 0.2 μm nylon filter into an UHPLC vial, and 1 µl was injected for liquid chromatography–tandem mass spectrometry (LC-MS/MS) analysis (Shimadzu Scientific, Columbia, MD, USA).
The LC–MS/MS system consisted of a Nexera X2 UPLC with 2 LC-30 AD pumps, an SIL-30 AC MP autosampler, a DGU-20A5 Prominence degasser, a CTO-30A column oven, and an SPD-M30A diode array detector coupled to an 8040-quadrupole mass spectrometer. Metabolites were separated on an iHILIC-Fusion column (100 by 2.1 mm, 3.5 µm; silica, Hilicon, Tampa, FL, USA) at a flow rate of 0.2 ml min−1 using a linear gradient of acetonitrile (B) and 25 mM ammonium acetate (A): 2 min, 80% B; 8 min, 30% B; 12 min, 30% B; 12.1 min, 80% B. The multiple reaction monitoring (MRM) values were optimized to 181.95 > 136.05, 147.95 >130.10, 147.10 > 130.00, 106.10 > 60.10, 116.15 > 70.15, and 175.00 > 70.10 for glufosinate, glutamate, glutamine, serine, proline, and arginine, respectively. These MRMs were used to identify and quantify each amino acid based on standard curves generated with serial dilutions of authentic standards.
Isobole Analysis
Dose–response experiments were conducted for both the herbicides glufosinate and saflufenacil to estimate the dose for 50% reduction in dry shoot biomass (ED50). The doses were 0, 9, 17, 35, 70, 140, 280, and 560 g ha−1 for glufosinate and 0, 0.5, 1, 2, 4, 8, 16, and 32 g ha−1 for saflufenacil. Each treatment had three replications, and the same experiment was conducted twice. The variable response was shoot dry biomass (percentage relative to untreated plants at 21 d after treatment [DAT]). An isobole shows dose combinations of two herbicides providing the same effect. For example, if x g ha−1 of glufosinate or y g ha−1 of saflufenacil individually provided 50% injury, then 1/2x glufosinate + 1/2y saflufenacil should also provide 50% injury. However, if the effect was synergistic or antagonistic, the output should be different than 50% injury. We tested five dose responses with different mixture ratios (Table 1) that were chosen based on the ED50 values obtained with the two herbicides tested individually. Then, the proportion of each herbicide required to achieve the ED50 was plotted in a scatter graph. An extension of the additive model was used to explain the interaction between the two herbicides and predict the shape of the isobole (Sørensen et al. Reference Sørensen, Cedergreen, Skovgaard and Streibig2007). If the shape is linear, the mixture effect is additive. If the curve is convex, the two herbicides are antagonistic to each other. Finally, a concave shape indicates that the mixture is synergistic (Streibig et al. Reference Streibig, Kudsk and Jensen1998).
Table 1. Proportion of glufosinate and saflufenacil doses used for the isobole analysis.a

a Five different dose–response curves were obtained with different herbicide mixture combinations. X = estimated dose for 50% reduction in dry weight (ED50).
Response of Protoporphyrinogen Oxidase–Resistant Amaranthus tuberculatus and Glufosinate-Resistant Soybean to Glufosinate and PPO Inhibitors
To investigate whether glufosinate enhances PPO inhibitors or PPO inhibitors enhance glufosinate, we tested a PPO-resistant A. tuberculatus biotype and a glufosinate-resistant soybean variety (‘S120090’, Liberty LinkTM, Pioneer, IA, USA). The resistance mechanism in the PPO-resistant A. tuberculatus biotype had been previously described as a codon deletion in PPO2 (ΔG210), providing 23-fold resistance to lactofen, and the seeds were collected in Adams County, IL (Dayan et al. Reference Dayan, Daga, Duke, Lee, Tranel and Doerksen2010; Patzoldt et al. Reference Patzoldt, Hager, McCormick and Tranel2006). A glufosinate-susceptible soybean variety (‘P0007A43R’, Pioneer, IA, USA) and a PPO-susceptible A. tuberculatus biotype from Nebraska were also included for comparison. Plants were grown as described earlier and 6-leaf-stage plants were sprayed with half the rate of glufosinate (280 g ha−1), low dose (<5% field rate) of PPO inhibitor (lactofen at 5 g ha−1 for A. tuberculatus, and saflufenacil at 1 g ha−1 for soybean), or the tank mixture of the two herbicides for each plant species. Lactofen was tested on A. tuberculatus, because the resistance level was higher for this herbicide compared with saflufenacil. Three replicates were evaluated, and the experiments were conducted twice. Response variables were visual injury (scale 0 to 100, where 0 means no effect and 100 implies plant death), ROS accumulation, and proto levels for A. tuberculatus; and visual injury, proto levels, and glufosinate and glutamate concentration for soybean. Glufosinate and glutamate were quantified over time up to 24 HAT, whereas other variables were evaluated at 12 HAT only.
Hydrogen peroxide (H2O2) and superoxide (O2−) were measured by staining leaf disks in solutions containing DAB and NBT, respectively. The DAB solution contained 0.1 g DAB solubilized in 200 ml water at pH 3.8. The NBT solution was composed of 0.1 g NBT, 13.6 g potassium phosphate monobasic, and 1.3 g sodium azide in 200 ml water. Sixteen leaf disks (5-mm diameter) from each treatment were placed in 20-ml glass tubes containing each staining solution. The samples were then shaken under 67 kPa vacuum for 1 h. Leaf disks were washed in distilled water and boiled in 70% (v/v) ethanol, which was replaced every 20 min for four cycles. Leaf disks were stored in 70% (v/v) ethanol for 12 h and scanned (Brother DCP-L2550DW, Bridgewater, NJ, USA). The levels of H2O2 or O2− were quantified using CS3 Photoshop (Adobe Systems, San Jose, CA, USA) by measuring the color intensity in each leaf disk and removing background levels (Dayan et al. Reference Dayan, Barker, Dayan and Ravet2019a; Mendes et al. Reference Mendes, Takano, Adegas, Oliveira, Gaines and Dayan2020; Takano et al. Reference Takano, Beffa, Preston, Westra and Dayan2019). Data were represented as relative intensity of treated samples compared with untreated samples (treated intensity − untreated intensity).
Glufosinate and glutamate were extracted and quantified as previously described. Proto extraction and analysis followed a protocol described elsewhere (Dayan et al. Reference Dayan, Owens, Corniani, Silva, Watson, Howell and Shaner2015). Leaf tissue (0.2 g) was ground to a powder in liquid nitrogen and homogenized in 2 ml methanol:0.1M NH4OH (9:1) and centrifuged at 10,000 × g for 15 min. The supernatant was saved and the pellet rehomogenized in 1 ml methanol:0.1M NH4OH (9:1), then centrifuged again at 10,000 × g for 15 min. Supernatants were pooled and then filtered through a 0.25-μm nylon syringe membrane filter before quantification with the LC-MS/MS system described earlier. Metabolites were separated in a biphenyl column (100 by 4.6 mm, 2.6 µm, 40 C) at a flow rate of 0.4 ml min−1 using a linear gradient of methanol (B) and 10 mM ammonium acetate (A): 0 min, 50% B; 8 min, 70% B; 11 min, 90% B; 13 min, 90% B; 13.5 min, 50% B; 17 min, 50% B. The MRM was optimized to 340.10 > 227.95 (Moulin et al. Reference Moulin, McCormac, Terry and Smith2008). Standard curves generated with serial dilutions of authentic standards were used for quantification.
Field Performance of Glufosinate + PPO Inhibitors on Kochia (Bassia scoparia)
Two separate experiments were conducted to evaluate the burndown effect in the field (Fort Collins, CO, 40.64°N, 104.99°W) between May 10 and June 20 of 2018 and 2019. Plants of kochia [Bassia scoparia (L.) A.J. Scott] were 15-cm tall in both years, and weed densities were 11 and 8 plants m−2 in 2018 and 2019, respectively. Weed density was assessed by randomly counting the number of plants in 1 m2 within each plot before herbicide application. The soil type was Satanta loam (fine-loamy, mixed, superactive, mesic Aridic Argiustolls) with 3.5% organic matter and 7.5 pH in the top 20 cm. Plots were 3-m wide and 7-m long with the central 18 m2 used for evaluations. Glufosinate (420 g ha−1) was applied alone or in tank mix with different PPO inhibitors (g ha−1): flumioxazin (2.5), saflufenacil (1), pyraflufen-ethyl (0.2), lactofen (4.2), and fomesafen (7.1). Methylated soybean oil (0.5% [v/v]) was added to all treatments, which were sprayed using a CO2-pressured backpack sprayer attached to a boom equipped with six flat spray nozzles (TeeJet®, Louisville, KY, USA) spaced 0.5 m from each other. Pressure was maintained at 25 kPa and speed at 3.2 km h−1, providing 160 L ha−1 spray volume. Visual injury (%) was visually graded at 21 DAT using a scale where 0 means no symptoms and 100 means death of all plants (Frans Reference Frans and Camper1986).
Efficacy of Glufosinate + Saflufenacil under Low Temperature and Humidity
Plants of A. palmeri at the 6-leaf stage were incubated under either 25 C and 70% RH or 13 C and 30% RH for 24 h. Plants were sprayed with glufosinate (280 g ha−1), saflufenacil (1 g ha−1), or glufosinate + saflufenacil (280 + 1 g ha−1) and immediately returned to their respective environmental conditions. Visual injury was evaluated at 14 DAT using the same method previously described.
Data Analysis
All experiments were repeated in time. Graphs were created using Prism software (GraphPad v. 8.3.1, San Diego, CA, USA). Statistical analysis was performed with R software (R Studio v. 3.6.1, Vienna, Austria). A four-parameter log-logistic regression was fit for the dose–response experiments considering the goodness-of-fit values and the fact that it is by far the most used model for this type of data set (Ritz and Streibig Reference Ritz and Streibig2014). Data normality and homogeneity of variance were checked by Q-Q plots and residuals versus fitted values, respectively. Treatments were considered fixed effects, and greenhouse experiments were conducted in a complete randomized design, while field experiments were conducted in a randomized complete block design. Differences between the two treatments were compared by t-test (P < 0.05). Data from experiments with more than two treatments were subjected to ANOVA, and means were compared using Tukey’s test (P < 0.05). The isobole analysis was performed based on previous literature (Sørensen et al. Reference Sørensen, Cedergreen, Skovgaard and Streibig2007; Streibig et al. Reference Streibig, Kudsk and Jensen1998).
Results and Discussion
Amino Acid Levels
Inhibition of GS by glufosinate depleted the levels of glutamine and glutamate, but increased proline and arginine at 24 HAT (Figure 1A). In most biochemical reactions, when the enzyme is inhibited, the substrate tends to accumulate, whereas the product is normally depleted. For instance, glyphosate inhibition of 5-enolpyruvylshikimate-3-phosphate synthase leads to the accumulation on shikimate to high levels (Shaner et al. Reference Shaner, Nadler-Hassar, Henry and Koger2005). Thus, glutamine levels are expected to decrease and glutamate to accumulate following glufosinate treatment. Instead, both glutamate and glutamine were depleted at 24 HAT. However, when glutamate levels were tracked over time after glufosinate treatment, a transient accumulation was observed (Figure 1B). Up to 3 HAT, glutamate levels were significantly higher in treated plants compared with the untreated plants. Therefore, glufosinate induces a transient accumulation of glutamate in A. palmeri.

Figure 1. Glufosinate reshapes the levels of amino acids in Amaranthus palmeri. Levels of glutamine, glutamate, proline, and arginine in untreated (gray) and glufosinate-treated (blue) plants at 24 h after treatment (A). Levels of glutamate over time in untreated (gray) and glufosinate-treated (blue) plants (B). Asterisks (*) indicate means are significantly different by t-test (P < 0.05) between untreated and glufosinate-treated within each amino acid or time point. Error bars represent the standard deviations of the means.
Isobole Analysis
The ED50 values for glufosinate and saflufenacil in A. palmeri were 50 and 2.5 g ha−1, respectively (Figure 2A and B). These doses were used in the isobole analysis to evaluate the interaction between these two herbicides (Figure 2C; Supplementary Figure S1). Lower ED50 values were obtained with the mixture compared with the herbicides used individually. This means that lower mixture doses were required to provide the same effect of the two herbicides individually. The concave shape of the isobole indicates strong synergism between glufosinate and saflufenacil. In addition to A. palmeri, we also tested the effect of glufosinate and PPO inhibitors (individually or in combination) on A. tuberculatus, B. scoparia, L rigidum, johnsongrass [Sorghum halepense (L.) Pers.], and junglerice [Echinochloa colona (L.) Link] (Supplementary Figures S2 and S3). In general, the enhanced activity with the mixture was more evident for broadleaf than grass species.

Figure 2. Enhanced herbicidal activity with glufosinate and saflufenacil. Dose response for glufosinate (A) and saflufenacil (B) in Amaranthus palmeri. Isobole analysis for the combination between the two herbicides in different proportions (C). The graph shows the proportion of each herbicide to achieve 50% reduction in dry biomass (ED50). If no synergism had been observed for the two compounds, data points were expected to fall into the red dashed line (additive effect). The concave shape of the isobole curve indicates high levels of synergism between the two herbicides. Error bars represent the standard deviations of the means.
Effect of Glufosinate + Lactofen on Amaranthus tuberculatus resistant to PPO Inhibitors
Half-rate of glufosinate (280 g ha−1) provided 20% injury on both PPO-susceptible and PPO-resistant A. tuberculatus at 12 HAT (Figure 3A–C). An ultra-low dose of lactofen (5 g ha−1) caused less than 5% injury on both biotypes. Conversely, the herbicide mixture provided 94% injury to the susceptible biotype, but only 20% injury to the resistant one. These results were consistent with the accumulation of ROS (Figure 3D). H2O2 and O2− accumulated to high levels in the susceptible biotype treated with glufosinate + lactofen. Low levels of these free radicals were observed in both biotypes after glufosinate treatment, similar to the effect of the herbicide mixture on the PPO-resistant biotype. In contrast, proto levels increased up to 19 nmol g−1 in the susceptible biotype treated with glufosinate + lactofen. Small quantities (<1 nmol g−1) were detected in the PPO-susceptible biotype treated with lactofen alone and in the PPO-resistant biotype treated with the herbicide mixture. Proto did not accumulate in either biotype treated with glufosinate. These results suggest that glufosinate enhances lactofen activity by increasing the accumulation of proto. Complementary data obtained with A. palmeri corroborated these findings (Supplementary Figure S2).

Figure 3. Glufosinate enhances the activity of protoporphyrinogen oxidase (PPO) inhibitors, but the mixture is not effective on PPO-resistant Amaranthus tuberculatus. Response of PPO-susceptible (A) and PPO-resistant (B) A. tuberculatus to glufosinate (Gluf) and lactofen (Lact). Visual injury (C), reactive oxygen species (ROS) (D), and protoporphyrin (E) in PPO-susceptible (gray) and PPO-resistant (blue) A. tuberculatus following herbicide treatments. Asterisks (*) indicate means are significantly different by t-test (P < 0.05) between biotypes within each treatment. Error bars represent the standard deviations of the means.
Effect of Glufosinate + Saflufenacil on Glufosinate-Resistant Soybean
Glufosinate (280 g ha−1) significantly caused more injury to glufosinate-susceptible (37%) than glufosinate-resistant (5%) soybean (Figure 4A). A very low dose of saflufenacil (1 g ha−1) provided less than 3% injury to both soybean varieties. The combination between these two herbicides, however, caused high levels of injury to glufosinate-resistant (68%) and even more to glufosinate-susceptible (82%) soybean. These injury levels provided by saflufenacil alone or in combination with glufosinate are consistent with proto accumulation (Figure 4B). Proto levels were very low (<0.5 nmol g−1) in plants treated with saflufenacil only. The addition of glufosinate to saflufenacil enhanced proto accumulation in both varieties, especially for the glufosinate-susceptible soybean.

Figure 4. Glufosinate + saflufenacil is toxic to glufosinate-resistant soybean due to a transient accumulation of glutamate and subsequent protoporphyrin accumulation. Visual injury (A) and protoporphyrin accumulation (B) in glufosinate-susceptible (red) and glufosinate-resistant (blue) soybean following glufosinate and saflufenacil treatments. Glufosinate (C) and glutamate (D) levels in glufosinate-susceptible (gray) and glufosinate-resistant (blue) soybean following glufosinate (280 g ha−1) treatment. Asterisks (*) indicate means are significantly different by t-test (P < 0.05) between soybean varieties or time point. Error bars represent the standard deviations of the means. HAT, hours after treatment.
Glufosinate levels increased over time in the glufosinate-susceptible soybean following treatment (Figure 4C). In contrast, glufosinate-resistant soybean had increased glufosinate levels until 4 HAT and levels decreased after that, due to the metabolism into N-acetyl-l-phosphinothricin by the acetyltransferase activity. This spike in glufosinate levels at 4 HAT was likely enough to cause some GS inhibition and a transient accumulation of glutamate even for glufosinate-resistant soybean (Figure 4D). Glutamate levels significantly increased (2- to 3-fold) after glufosinate treatment in soybean. These results support the hypothesis that glufosinate synergizes PPO inhibitors by increasing the levels of glutamate, which is diverted into the accumulation of proto in the chlorophyll biosynthesis pathway.
Field Performance of Glufosinate + PPO Inhibitors on Bassia scoparia
Glufosinate (420 g ha−1) provided 25% injury on B. scoparia (Figure 5). Most of the PPO inhibitors (except flumioxazin) applied in low doses caused similar levels of injury compared with glufosinate alone, with no statistical differences (P < 0.05) among them. The addition of any PPO inhibitor to glufosinate significantly increased injury levels compared with glufosinate only. Glufosinate + saflufenacil provided the highest levels of injury on B. scoparia but did not differ from glufosinate + pyraflufen. These results support our findings obtained in the greenhouse and in the lab, demonstrating the enhanced activity with glufosinate + PPO inhibitors compared with the two herbicides applied individually.

Figure 5. Glufosinate enhances the activity of protoporphyrinogen oxidase (PPO) inhibitors in the field. Performance of glufosinate and PPO-inhibiting herbicides applied individually or in tank mix on Bassia scoparia. Gluf, glufosinate; flumi, flumioxazin; saflu, saflufenacil; pyraflu, pyraflufen; lact, lactofen; fome, fomesafen. Means followed by the same letter do not differ according to Tukey’s test (P < 0.05). Error bars represent the standard deviations of the means.
Saflufenacil Alleviates the Lack of Efficacy by Glufosinate under Low Temperature and Low Humidity
Both glufosinate (280 g ha−1) and glufosinate + saflufenacil (280 + 1 g ha−1) provided 100% injury to A. palmeri under 25 C and 70% RH (Figure 6A) at 14 DAT. Glufosinate efficacy decreased to 60% under 13 C and 30% humidity. Less than 10% injury was obtained with saflufenacil under both conditions. The combination between glufosinate and saflufenacil, however, still provided 100% injury, even under low temperature and low relative humidity. These results suggest that a low dose of saflufenacil can help to overcome the negative effect of low temperature and humidity on glufosinate efficacy.
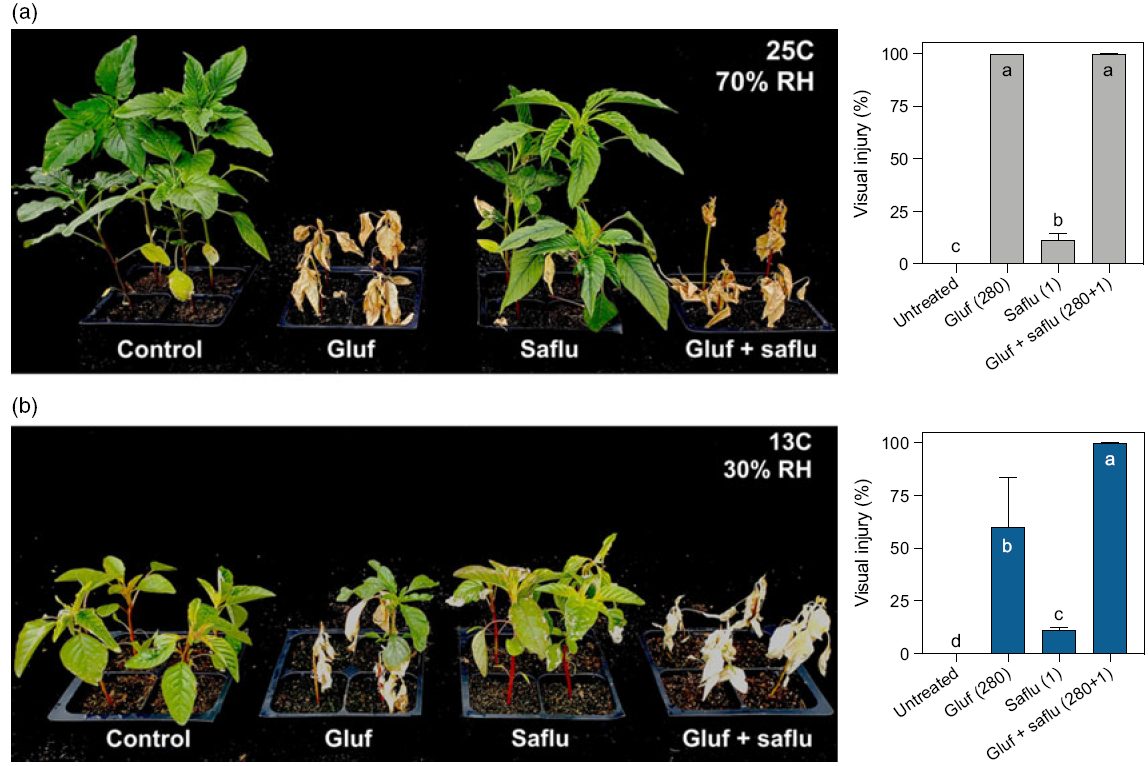
Figure 6. Saflufenacil (Saflu) at 1 g ha−1 can overcome the lack of efficacy by glufosinate (Gluf) at 280 g ha−1 on Amaranthus palmeri under low temperature and humidity. Visual injury provided with glufosinate + saflufenacil compared with the two herbicides applied individually at high (A) and low (B) temperature and humidity. Means with the same letter do not differ according to Tukey’s test (P < 0.05). Error bars represent the standard deviations of the means.
In the light of an increased number of multiple herbicide–resistant weeds, enhancing the efficacy of alternative tools like glufosinate is needed. Glufosinate performance can vary in the field, because it does not translocate well in plants (Beriault et al. Reference Beriault, Horsman and Devine1999; Everman et al. Reference Everman, Mayhew, Burton, York and Wilcut2009; Kumaratilake et al. Reference Kumaratilake, Lorraine-Colwill and Preston2002; Takano et al. Reference Takano, Beffa, Preston, Westra and Dayan2020b). Thus, we took a biochemical approach to enhance the activity of glufosinate. Both glufosinate and PPO inhibitors are fast-acting herbicides due to the accumulation of ROS and therefore could enhance each other’s activity. The isobole analysis confirmed our hypothesis that the mixture glufosinate + saflufenacil is synergistic and stronger for glufosinate enhancing PPO inhibitor than PPO inhibitor enhancing glufosinate (Figure 2C). PPO inhibitors target chlorophyll biosynthesis by inhibiting PPO, a key enzyme in the pathway (Figure 7). Inhibition of PPO leads to proto accumulation, the product of the reaction catalyzed by the enzyme (Duke et al. Reference Duke, Lydon, Becerril, Sherman, Lehnen and Matsumoto1991). The accumulation of proto is toxic, because it can absorb light energy and transfer to molecular oxygen, generating ROS and causing lipid peroxidation followed by cell death (Dayan et al. Reference Dayan, Barker, Bough, Ortiz, Takano, Duke and Moo-Young2019b).

Figure 7. Visual observation and the physiological basis of the synergistic effect between glufosinate and protoporphyrinogen oxidase (PPO) inhibitors on Amaranthus palmeri. Glutamate is the precursor for the biosynthesis of glutamine, arginine, proline, and chlorophyll. Inhibition of glutamine synthetase (GS) diverges the fate of glutamate into proline/arginine and chlorophyll biosynthetic pathways. Inhibition of both GS and PPO leads to increased protoporphyrin accumulation and subsequent formation of reactive oxygen species (ROS).
The biochemical basis for the synergism observed with glufosinate + saflufenacil relies on a change in glutamate flux by glufosinate, driving this amino acid toward chlorophyll synthesis, resulting in enhanced accumulation of proto with the addition of the PPO inhibitor (Figure 7). The synergism effect is stronger for glufosinate enhancing the activity of PPO inhibitors than the reverse. This is supported by the fact that PPO-resistant A. tuberculatus plants were not killed with the herbicide mixture and showed similar injury levels when glufosinate was sprayed alone (Figure 3). Glutamate, the substrate for GS, transiently accumulates shortly after glufosinate treatment, and it is diverted into other metabolic pathways including proline, arginine, and chlorophyll biosynthesis (Figures 1, 4, and 7). Proline and arginine accumulate after GS inhibition, supporting our hypothesis.
Glufosinate-resistant soybean express the pat gene and metabolize glufosinate by adding an acetyl group to the herbicide molecule (Dröge et al. Reference Dröge, Broer and Pühler1992; Strauch et al. Reference Strauch, Wohlleben and Pühler1988). Even though these plants are resistant to the herbicide, glufosinate concentration in the leaf tissue increased over the first 4 HAT and caused partial GS inhibition (Figure 4A). This is because uptake is probably faster than metabolic rates during the first few hours after treatment, leading to glufosinate accumulation (Carbonari et al. Reference Carbonari, Latorre, Gomes, Velini, Owens, Pan and Dayan2016). Consequently, glutamate levels also increased up to 4 HAT, enhancing proto accumulation in the presence of saflufenacil (Figure 4). An unknown compound with the same mass as glutamate-1-semialdehyde and glutamate-4-semialdehyde accumulates at high levels in glufosinate-treated plants (Supplementary Figure S4). These two metabolites are precursors for chlorophyll and proline/arginine biosynthesis, respectively, supporting the fact that glufosinate synergizes PPO-inhibitor activity by a transient glutamate accumulation. Proto accumulation at high levels leads to ROS generation and the catastrophic consequences of lipid peroxidation (Dayan et al. Reference Dayan, Barker, Bough, Ortiz, Takano, Duke and Moo-Young2019b).
To evaluate the applicability of the herbicide mixture, we tested glufosinate in combination with low doses of different PPO inhibitors to control B. scoparia in the field. A tank mix with PPO inhibitors provided increased injury levels compared with glufosinate only (Figure 5). The highest levels of injury were obtained with glufosinate + saflufenacil, similar to levels seen with glufosinate + pyraflufen-ethyl. While glufosinate efficacy can be strongly affected by low temperature and low humidity (Coetzer et al. Reference Coetzer, Al-Khatib and Loughin2001; Kumaratilake and Preston Reference Kumaratilake and Preston2005), this can be overcome with the addition of a low dose of saflufenacil to the tank (Figure 6). Future research will investigate potential opportunities to mitigate resistance to PPO inhibitors with the herbicide mixture. Furthermore, while the tank mix of glufosinate and PPO inhibitors seems to be very promising for burndown applications, it was not safe for glufosinate-resistant soybean POST. However, if used on crops tolerant to both glufosinate and PPO inhibitors, this approach could offer new opportunities for better weed control, especially where resistant weeds are present. In this study, we used reduced doses of both glufosinate and PPO inhibitors because we wanted to prove the concept. Under real-world circumstances, full doses of the herbicides must be tested, especially when managing resistant weeds. Therefore, more data are needed to better understand the most appropriate uses for this herbicidal composition as well as potential technical adjustments (e.g., doses, active ingredients, weed species, cropping systems).
The combination of glufosinate and PPO inhibitors provided enhanced herbicidal activity compared with the products applied individually. Inhibition of GS by glufosinate leads to a transient accumulation of glutamate, the precursor for chlorophyll biosynthesis in plants. Inhibition of chlorophyll biosynthesis by PPO inhibitors causes accumulation of proto, which is enhanced in the presence of glufosinate. Thus, the synergism observed with the mixture results from proto accumulation to higher levels when both GS and PPO are inhibited simultaneously. The herbicide combination provided improved weed control in the field and may help to overcome negative effects of environmental conditions on glufosinate performance.
Supplementary material
To view supplementary material for this article, please visit https://doi.org/10.1017/wsc.2020.39
Acknowledgments
This research was funded by BASF Corporation. No conflicts of interest have been declared. We thank Jens Streibig for his assistance with the isobole data analysis.