The metabolic syndrome (MetS) is a highly complex disorder with multiple definitions. The MetS is not a disease in itself; however, it encompasses an assembly of cardiometabolic risk factors such as visceral obesity, dyslipidaemia and insulin resistance (IR), ultimately leading to type 2 diabetes and CVD. According to the National Cholesterol Education Program clinical criteria, the MetS comprises at least three of the following: a waist circumference >102 cm (40 inches) in men and >88 cm (35 inches) in women; TAG levels ≥ 1·70 mmol/l; HDL-cholesterol levels <1·04 mmol/l in males and <1·30 mmol/l in females; blood pressure of ≥130/≥85 mmHg; and fasting glucose levels of ≥6·10 mmol/l(1). The most recent data on the prevalence of the MetS in the USA come from National Health and Nutrition Examination Survey data from 2007 to 2014(Reference Shin, Kongpakpaisarn and Bohra2). Overall, the prevalence of the MetS in the USA has remained stable over this time period at 34·3 % of the adult population, almost evenly distributed among females and males at 33·3 and 35·3 %, respectively(Reference Shin, Kongpakpaisarn and Bohra2). In Canada, the most recent prevalence rate reported in 2014 is 19·1 % of the Canadian population, approximately one in five Canadians(Reference Rao, Dai and Lagace3). As rates of obesity have nearly tripled since 1975, it is likely that global rates of the MetS will follow simultaneously(4).
A high-fat diet (HFD) or a Western diet (35 % kcal or kJ fat) has shown to propel the body into a lipotoxic state, an accumulation of lipids in plasma and non-adipose tissues(Reference Sokolowska and Blachnio-Zabielska5). These leading lipotoxic lipids commonly include TAG, long-chain NEFA and their acyl-CoA, diacylglycerols and ceramide (Cer). Among these lipids, there is recent interest in the role of Cer in the MetS and its related cardiometabolic risk factors. A very recent study by Choromanska et al.(Reference Choromanska, Mysliwiec and Razak Hady6) reported women with morbid obesity, and the MetS had elevated Cer in visceral adipose tissue, 21 % greater than their lean and healthy counterparts. Additionally, Cer lipotoxicity has been linked to IR, inflammation and dyslipidaemia(Reference Sokolowska and Blachnio-Zabielska5,Reference Gomez-Munoz, Presa and Gomez-Larrauri7,Reference Iqbal, Walsh and Hammad8) . While some evidence suggests Cer lipotoxicity is occurring in the MetS, there is much to learn in regard to the role of Cer in the pathogenesis and various components of this condition.
Although a HFD is shown to promote Cer lipotoxicity, available data indicate that dietary fatty acid quality is likely more influential than total fat consumption. The intake of SFA has shown to up-regulate genes involved in Cer synthesis, thereby promoting Cer accumulation in plasma, skeletal muscle and liver tissue(Reference Frangioudakis, Garrard and Raddatz9–Reference Chocian, Chabowski and Żendzian-Piotrowska11). In contrast, n-3 long-chain PUFA (LCPUFA), such as EPA (C20 : 5n-3) and DHA (C22 : 6n-3) have led to reductions in total Cer and genes involved in Cer synthesis in vitro (Reference Opreanu, Lydic and Reid12–Reference Jin, Lu and Li15). Benefits of n-3 LCPUFA have also been reported in vivo, demonstrating dietary n-3 LCPUFA reduced adipose, skeletal muscle and hepatic tissue Cer in rodents(Reference Kasbi-Chadli, Ferchaud-Roucher and Krempf16–Reference Lanza, Blachnio-Zabielska and Johnson20), and fatty fish consumption reduced plasma Cer in humans with CVD(Reference Lankinen, Schwab and Erkkila21).
Therefore, this review aims to examine the evidence regarding the potential of n-3 LCPUFA, EPA and DHA, to improve Cer lipotoxicity, and whether these changes affect the main characteristics of the MetS including IR, inflammation and dyslipidaemia in vitro and in vivo.
Ceramides
Ceramides structure and metabolism
Cer, a class of sphingolipids, consists of a sphingosine backbone attached to a fatty acid moiety through an amide bond. While the level of Cer in human plasma sphingolipid is only 2·8 %(Reference Hammad, Pierce and Soodavar22), Cer is the central molecule in sphingolipid metabolism. There are three pathways of Cer generation including de novo synthesis, the hydrolytic pathway and the salvage pathway (Fig. 1). (i) The de novo synthesis of Cer begins with the condensation of serine and palmitoyl-CoA to produce 3-ketosphinganine via the enzyme serine palmitoyltransferase in the endoplasmic reticulum (ER). 3-Ketosphinganine is reduced to sphinganine, which is acylated to dihydroceramide by Cer synthase and then oxidised to Cer. Once formed, Cer can be transported from the ER to the Golgi apparatus to form sphingomyelins and other complex sphingolipids, including glucosylceramide and glycosphingolipids, which are synthesised through the addition of various head groups. (ii) In the hydrolytic pathway, Cer is synthesised via the hydrolysis of sphingomyelin and complex sphingolipids. Cer can also be degraded into sphingosine, which can subsequently be phosphorylated via sphingosine kinase to form sphingosine-1-phosphate. (iii) The salvage pathway is the synthesis of Cer from sphingosine.

Fig. 1. Pathways of ceramide synthesis. De novo synthesis of ceramide (Cer) begins with the condensation of serine and palmitoyl CoA by serine palmitoyltransferase producing 3-ketosphingnanine, which is reduced to sphinganine. Sphinganine is acylated to dihydroceramide and then oxidised to Cer. Modification of Cer by various enzymes can form other sphingolipids including sphingomyelins, glucosylceramides, ceramide-1-phosphate, 1-O-acylceramides and sphingosine, all of which can be converted back to ceramide. The hydrolytic pathway produces Cer via the hydrolysis of sphingomyelins and complex sphingolipids such as glucosylceramides, whereas the salvage pathway synthesises Cer from sphingosine.
Cer acts as a second messenger to regulate a variety of cellular functions. For instance, increases in Cer levels induced by stress stimuli can lead to apoptosis(Reference Yabu, Shiba and Shibasaki23). Cer can either interact with intracellular target proteins or create Cer-enriched membrane platforms, which cluster receptors to activate signalling pathways. As Cer has the potential to affect a variety of internal mechanisms, changes in Cer concentrations in plasma and tissues can lead to lipotoxicity, resulting in several diseases including neurodegenerative disorders, cancers and the MetS. Increasing evidence also shows the relevance of acyl chain length of Cer in the pathogenesis of such diseases. The acyl chain length of Cer varies between 14 and 30 carbons, consisting of mainly SFA or MUFA. In general, C18 : 0-Cer is the most abundant specie in the brain, while C24 : 0- and C24 : 1-Cer are abundant in other tissues including liver, muscle, heart and plasma(Reference Manni, Sot and Arretxe24), indicating their tissue specificity and distinct functional roles. This tissue specificity is apparent in various disease conditions, which may need to be considered when assessing the role of Cer in the pathogenesis of disease. Filippov et al.(Reference Filippov, Song and Zhang25) have shown C16 : 0-, C18 : 0- and C20 : 0-Cer to be elevated in the brains of people with neurodegenerative diseases when compared with healthy brain tissues. Whereas plasma C16 : 0-, C18 : 0-, C18 : 1- and C24 : 0-Cer were elevated in patients with colorectal cancer compared with healthy controls(Reference Kurz, Parnham and Geisslinger26). Lastly, diverse differences in specific Cer species have also been found in parameters of the MetS including IR and inflammation, to be described later in this review.
Ceramide lipotoxicity in the metabolic syndrome
It is not surprising that obesity and the MetS are often closely related. One of the main contributing factors of obesity is the consumption of energy-dense diets that exceed one’s needs, often containing excessive amounts of fat, in particular SFA. Over time, this diet and weight gain lead to lipid accumulation in tissues including skeletal muscle and liver, directly inducing the characteristics of the MetS including IR, inflammation and dyslipidaemia. One of these accumulating and biologically active lipids is Cer. Elevations in plasma and skeletal muscle Cer are found in both obese children(Reference Wasilewska, Bobrus-Chociej and Harasim-Symbor27) and adults(Reference Adams, Pratipanawatr and Berria28), when compared with lean individuals. A variety of evidence has emerged suggesting that Cer lipotoxicity contributes to the pathogenesis of chronic disease including the MetS, as the inhibition of Cer synthesis has shown to improve insulin sensitivity and inhibit proinflammatory signalling(Reference Blachnio-Zabielska, Chacinska and Vendelbo10,Reference Jin, Lu and Li15) . Hence, the following will focus on the relationship between Cer and the MetS to identify if and how Cer promotes MetS characteristics including IR, inflammation and dyslipidaemia.
Ceramides and insulin resistance
IR is a reduction in the body’s ability to appropriately respond to insulin action. This aberrant signalling leads to a reduction in peripheral glucose uptake and hepatic gluconeogenesis, resulting in hyperinsulinaemia and hyperglycaemia(Reference Sokolowska and Blachnio-Zabielska5). In both human and animal models, the tissue accumulation of Cer in liver or skeletal muscle is reported to contribute to the development of IR(Reference Sokolowska and Blachnio-Zabielska5,Reference Blachnio-Zabielska, Chacinska and Vendelbo10,Reference Broskey, Obanda and Burton29–Reference Turinsky, O’Sullivan and Bayly32) . Total Cer concentrations in skeletal muscle have shown to be two-fold higher in patients with type 2 diabetes(Reference Broskey, Obanda and Burton29) and were also elevated in insulin resistant patients (homoeostatic model assessment of insulin resistance, HOMA-IR > 3·19) with steatosis or non-alcoholic steatohepatitis(Reference Luukkonen, Zhou and Sadevirta30). However, the multifactorial nature of IR likely complicates this relationship as conflicting evidence suggests IR is not always paired with changes in Cer concentrations(Reference Blachnio-Zabielska, Chacinska and Vendelbo10,Reference Lee, Pinnamaneni and Eo33,Reference Kumashiro, Erion and Zhang34) .
The suggested primary mechanism by which Cer reduces insulin signalling is via the inhibition of protein kinase B (Akt/PKB)(Reference Chavez and Summers35) (Fig. 2). Akt/PKB is an essential protein in the cellular uptake of glucose and glycogen synthesis, and therefore IR. The mechanisms of insulin action have been described in detail elsewhere(Reference Petersen and Shulman36). In short, upon binding of insulin to insulin receptor, a number of proteins are sequentially activated via phosphorylation initiating with insulin substrate receptors, then phosphoinositol 3-kinase, phosphoinositide-dependent kinase and Akt/PKB. Phosphorylated Akt/PKB promotes translocation of GLUT4 to the plasma membrane for glucose uptake and the activation of glycogen synthase. The ability of Cer to inhibit Akt/PKB is likely due to two independent mechanisms as described by Petersen & Shulman(Reference Petersen and Shulman36). Cer activates protein phosphatase A2, which in turn decreases Akt/PKB serine phosphorylation and consequentially inhibits the translocation of GLUT4 to the plasma membrane. Second, Cer activates atypical protein kinase Cζ, which reduces responsiveness of insulin-stimulated Akt/PKB. These mechanisms have been tested using inhibitors of both protein phosphatase A2 and protein kinase Cζ, which reverse Cer-induced Akt/PKB (Fig. 2) inhibition(Reference Hajduch, Turban and Le Liepvre37,Reference Teruel, Hernandez and Lorenzo38) . In addition to Akt/PKB, Cer may hinder insulin signalling by inhibiting insulin receptor substrate 1 through the double-stranded RNA-dependent protein kinase/JNK pathway, as evidenced in muscle cells(Reference Hage Hassan, Pacheco de Sousa and Mahfouz39).

Fig. 2. Ceramide (Cer) in the pathogenesis of insulin resistance. Cer activates either protein phosphatase A2 (PPA2) or protein kinase Cζ (PKCζ), which decreases protein kinase B (Akt/PKB) phosphorylation, resulting in insulin resistance. Cer may also hinder insulin signalling by inhibiting insulin receptor substrate (IRS) via the double-stranded ARN-activated protein kinase (PKR)/c-Jun N terminal kinase (JNK) pathway. Mitochondrial dysfunction leads to decreased β-oxidation and increased reactive oxygen species (ROS) production, which in turn cause Cer accumulation and insulin resistance. Cer further negatively affects mitochondrial function or increases ROS production, which also damages mitochondria and leads to additional Cer accumulation. Endoplasmic reticulum (ER) stress negatively affects mitochondria by triggering Ca2+ uptake in mitochondria, which results in impaired oxidative metabolism, increased ROS production and Cer accumulation, ultimately leading to the inhibition of Akt/PKB and insulin resistance. SMase, sphingomyelinase; SPT, serine palmitoyltransferase; PIP2, phosphatidylinositol diphosphate; PIP3, phosphatidylinositol triphosphate; PI3K, phosphoinositide-3-kinase; PDK1, phosphoinositide-dependent kinase-1.
A mechanism by which Cer may indirectly interfere with insulin signalling is through its dynamic relationship with mitochondrial dysfunction (Fig. 2). Disruptions in mitochondrial function have been found in obese and IR individuals, both of which are associated with excess fat consumption(Reference Sergi, Naumovski and Heilbronn40). The oversupply of fatty acids combined with impaired metabolic substrate oxidation leads to an accumulation of intracellular fatty acids, providing substrate for Cer synthesis(Reference Sergi, Naumovski and Heilbronn40). In a feedforward manner, Cer accumulation has shown to further negatively affect mitochondrial function via alteration of mitochondrial dynamics and promoting reactive oxygen species (ROS) production by adversely affecting mitochondrial respiration(Reference Smith, Tippetts and Brassfield41,Reference Law, Liao and Moore42) . Accumulation of ROS enhances mitochondrial dysfunction by damaging mitochondrial DNA, leading to additional Cer accumulation(Reference Sergi, Naumovski and Heilbronn40). Consequently, Cer promotes additional ROS production by activating enzymes such as NADPH oxidase and NO synthase(Reference Li and Zhang43,Reference Guo, Sun and Chen44) . In turn, this injurious cycle of elevated Cer, ROS and mitochondrial dysfunction leads to the inhibition of Akt/PKB and therefore the induction of IR. In addition, accumulating evidence suggests ER stress also contributes to HFD-induced mitochondrial dysfunction. The contact between mitochondria and the ER, referred to as mitochondria-associated ER membrane, is an important site for exchanging Ca2+, lipids and other metabolites. Overconsumption of fat induces ER stress, which triggers Ca2+ uptake in mitochondria through the mitochondrial-associated ER membrane resulting in impaired mitochondrial oxidative metabolism, ROS production and Cer synthesis(Reference Lepretti, Martucciello and Aceves45).
Some evidence suggests that the relationship between Cer and IR is not only tissue-specific but may also vary depending on the acyl chain length of Cer species. In HFD-fed mice, significant elevations in C18 : 0- and C18 : 1-Cer have been found, along with indications of IR(Reference Blachnio-Zabielska, Chacinska and Vendelbo10,Reference Lanza, Blachnio-Zabielska and Johnson20) . C18 : 0- and C18 : 1-Cer were strongly correlated (r 0·73–0·93) with markers of IR including HOMA-IR, oral glucose tolerance and insulin tolerance tests(Reference Blachnio-Zabielska, Chacinska and Vendelbo10), whereas C14 : 0-, C16 : 0-, C20 : 0-, C22 : 0-, C24 : 0- and C24 : 1-Cer have either reduced or remained unchanged in IR mice fed a HFD(Reference Blachnio-Zabielska, Chacinska and Vendelbo10,Reference Lanza, Blachnio-Zabielska and Johnson20) . In humans, longer chain Cer has been implicated in the development of IR in those with obesity or type 2 diabetes(Reference Broskey, Obanda and Burton29). For instance, elevations in C18 : 1-, C20 : 0-, C22 : 0-, C24 : 0-, and C24 : 1-Cer but not C16 : 0- and C18 : 0-Cer have been found in skeletal muscle of type 2 diabetics(Reference Broskey, Obanda and Burton29). However, hepatic C16 : 0-Cer has shown to be positively associated with HFD-induced obesity and glucose intolerance, and elevations in C14 : 0-, C16 : 0-, C16 : 1-, C18 : 0-, C18 : 1- and C22 : 1-Cer have been found in visceral white adipose tissue of obese IR patients(Reference Turpin, Nicholls and Willmes46). These inconsistencies lead to many questions regarding the role of tissue-specific acyl chain length of Cer in the pathogenesis of IR, and more research is required to understand this complex relationship.
Ceramides and inflammation
Inflammation is the body’s natural immune response to infection or tissue injury, whereas in chronic conditions, consistent low-grade inflammation is often present. This form of inflammation is systemic and a continuous low-level activation of the immune system as evidenced by slightly prolonged increases in inflammatory mediators. The MetS is associated with chronic inflammation as higher levels of proinflammatory indicators including C-reactive protein and platelet:lymphocyte ratio are found among those with the MetS, in comparison with healthy individuals(Reference Akboga, Canpolat and Yuksel47). Currently, the mechanisms by which chronic inflammation is associated with the MetS are not well understood; however, there is evidence to suggest that Cer may play a role in this complex relationship. Increased concentrations of TNFα, total Cer and C18 : 0-, C20 : 0-, C24 : 0- and C24 : 1-Cer species have been reported in obese individuals with type 2 diabetes(Reference Haus, Kashyap and Kasumov48). Among this population, TNFα was positively correlated with increased C18 : 0- and C18 : 1-Cer(Reference Haus, Kashyap and Kasumov48).
There are probably a variety of ways in which sphingolipids are involved in inflammation including Cer. One of the most well-understood mechanisms involves SMases (Fig. 3), the enzymes required for the hydrolysis of sphingomyelin to Cer. Several isoforms of SMases exist and are often categorised by the pH in which they are optimally functional, categorising these enzymes as either acid, neutral or alkaline (aSMase, nSMase or alSMase, respectively)(Reference Goni and Alonso49). Most research has focused on aSMases and nSMases, especially in relation to inflammation. aSMases are either lysosomal or secretory in nature and are most effective at a pH of about 5, whereas nSMases are integral membrane proteins and have an optimal pH of 7(Reference Goni and Alonso49). In vitro studies utilising a variety of cell types have proposed that Cer synthesis via SMases and inflammation interact through feedforward mechanisms(Reference Gomez-Munoz, Presa and Gomez-Larrauri7,Reference Nixon50) . Elevated Cer promotes synthesis of proinflammatory stimuli such as TNFα, interferon-γ, IL-1β or platelet-activating factor(Reference Gomez-Munoz, Presa and Gomez-Larrauri7,Reference Clarke, Guthrie and Hannun51) . These proinflammatory mediators promote the activity of both aSMases and nSMases, thus encouraging Cer synthesis and consequentially stimulate further inflammation(Reference Gomez-Munoz, Presa and Gomez-Larrauri7,Reference Clarke, Guthrie and Hannun51) . In MCF7 breast carcinoma cells, treatment with TNFα and IL-1β increased aSMase secretion and activity, which increased cellular Cer concentrations(Reference Jenkins, Canals and Idkowiak-Baldys52). TNFα treatment has also increased the activity of nSMase2 in A549 epithelial cells(Reference Clarke, Truong and Hannun53).

Fig. 3. Ceramide (Cer) involvement in inflammation. Elevated Cer promotes synthesis of pro-inflammatory cytokines, which stimulate acid sphingomyelinase (aSMase) and neutral sphingomyelinase (nSMase) activity, leading to ceramide synthesis and further inflammation. aSMase and nSMase induce inflammation via activation of either NF-κB or cytosolic phospholipase A2 (cPLA2), respectively. COX-2, cyclo-oxygenase-2.
aSMase-induced Cer synthesis has shown to promote inflammation through activation of the transcription factor NF-κB(Reference Schütze, Potthoff and Machleidt54). Upon activation, NF-κB promotes the up-regulation of genes encoded for many proinflammatory cytokines and enzymes including IL-1β, IL-6, IL-8, monocyte chemoattractant protein-1 and cyclo-oxygenase-2, which in turn promote further Cer synthesis(Reference Nixon50) (Fig. 3). Research by Sakata et al.(Reference Sakata, Ochiai and Shimeno55) has investigated this relationship in macrophages derived from the monocytic cell line THP-1, treated with lipopolysaccharide. Lipopolysaccharide treatment promoted increases in cellular Cer, proinflammatory cytokines including TNFα, IL-1β, IL-6 and IL-8 and nuclear levels of NF-κB. However, by inhibiting SMase using the sphingomyelin analogue (SMA)-7, researchers were able to reduce Cer concentrations and decrease the release of TNFα, IL-1β, IL-6 and nuclear levels of NF-κB. While the activity of aSMase was increased by lipopolysaccharide and inhibited by SMA-7, nSMase activity was unaffected. These findings confirmed in vivo utilising mice with dextran sodium sulphate-induced colitis. Treatment with SMA-7 for 21 d reduced tissue concentrations of TNFα, IL-1β, IL-6 and intestinal inflammation. Thus, Cer synthesis via aSMase may play a substantial role in inflammation, making aSMase a potential therapeutic target for inflammatory and chronic diseases. In addition, there is evidence to suggest nSMases or Cer-1-phosphate synthesis via Cer kinase is able to induce inflammation by promoting the activity of cytosolic phospholipase A2. Cytosolic phospholipase A2 catalyses the release of arachidonic acid (C20 : 4n-6) from phospholipids, thus promoting the synthesis of pro-inflammatory eicosanoids(Reference Gomez-Munoz, Presa and Gomez-Larrauri7,Reference Nixon50) .
Ceramides and dyslipidaemia
Dyslipidaemia is the pathological imbalance of circulating lipids associated with increases in total cholesterol (TC), TAG and LDL-cholesterol, often accompanied with reductions in HDL-cholesterol. Increases in both plasma Cer concentrations and hyperlipidaemia commonly occur in unison in both obese animals and humans(Reference Iqbal, Walsh and Hammad8). Approximately 98 % of circulating Cer are bound to lipoprotein subfractions and are equally distributed on very LDL, LDL-cholesterol and HDL-cholesterol(Reference Boon, Hoy and Stark56). Common species of Cer including C16 : 0-, C22 : 0-, C24 : 0-, C24 : 1-Cer tend to be evenly distributed among these lipoproteins, and circulating lipoprotein-bound Cer is found to differ among lean, obese and obese type 2 diabetic individuals(Reference Boon, Hoy and Stark56). Total circulating Cer concentrations were elevated among obese individuals with and without diabetes. No differences in very LDL-Cer among groups were reported; however, HDL-Cer was elevated in obese individuals without diabetes, whereas LDL-Cer was significantly elevated among obese diabetics. As LDL-Cer was positively correlated with HOMA-IR, it is likely that LDL-Cer plays a role in the pathogenesis of Cer-induced IR.
Traditionally, dyslipidaemia is considered a key risk factor for the development of CVD. More recently, elevated circulating Cer concentrations may be considered a new predictive tool for the development of CVD(Reference Laaksonen, Ekroos and Sysi-Aho57–Reference Meeusen, Donato and Bryant59). In coronary artery disease patients, plasma Cer was predictive of coronary death following adjustment for traditional CVD predictors including LDL-cholesterol, HDL-cholesterol, TC, TAG and C-reactive protein(Reference Laaksonen, Ekroos and Sysi-Aho57). Consistent with research regarding IR and inflammation, distinct long-chain Cer species differ in their ability to predict cardiovascular death(Reference Laaksonen, Ekroos and Sysi-Aho57,Reference Lemaitre, Jensen and Hoofnagle58) . While long-chain Cer such as C16 : 0- and C18 : 0-Cer is associated with an increased risk, very long-chain Cer species C22 : 0- and C24 : 0-Cer may be less harmful or associated with lower risk of cardiovascular death(Reference Laaksonen, Ekroos and Sysi-Aho57,Reference Lemaitre, Jensen and Hoofnagle58) . It is suggested that Cer increases atherogenesis by promoting LDL infiltration into the vessel wall, endothelial dysfunction and by increasing inflammation; however, considering the differences among Cer species, there are likely numerous mechanisms involved. Taken together, a Cer risk score as utilised by Laaksonen et al.(Reference Laaksonen, Ekroos and Sysi-Aho57) and Meeusen et al.(Reference Meeusen, Donato and Bryant59) may be a novel independent predictive tool for CVD, yet more research is required to develop a standardised and validated tool and to understand the unique involvement of distinct Cer species.
Dietary influences on ceramides
High-fat diet on ceramides
The literature describes a typical Western diet as a pattern of eating that commonly consists of high intakes of lipid (35 % of kcal or kJ)(Reference Freedman, King and Kennedy60). Often, a high proportion of lipid intake comprises SFA from animal products and highly processed foods. HFD consumption has been linked to not only the development of MetS and its associated cardiometabolic risk factors but also increases in Cer synthesis. Studies consistently demonstrated that HFD consumption induces Cer accumulation in various tissues including muscle, liver, adipose tissue and plasma by up-regulating both de novo synthesis and the sphingomyelin pathway(Reference Blachnio-Zabielska, Chacinska and Vendelbo10,Reference Chocian, Chabowski and Żendzian-Piotrowska11,Reference Shah, Yang and Lee61) demonstrated by an increase in the gene expression and activity of serine palmitoyltransferase(Reference Shah, Yang and Lee61,Reference Cinar, Godlewski and Liu62) , Cer synthase(Reference Cinar, Godlewski and Liu62) and SMase(Reference Chocian, Chabowski and Żendzian-Piotrowska11,Reference Shah, Yang and Lee61) . As mentioned previously, the overconsumption of fat also promotes Cer synthesis by inducing mitochondrial dysfunction, ER stress and consequential ROS production in skeletal muscle(Reference Lepretti, Martucciello and Aceves45,Reference Zalewska, Ziembicka and Zendzian-Piotrowska63) . These HFD-induced elevations in plasma and tissue Cer promote inflammatory stimuli such as TNFα and IL-1β and IR through Akt/PKB inhibition, suggesting Cer may be a key intermediary linking a HFD to the development of the MetS(Reference Gomez-Munoz, Presa and Gomez-Larrauri7,Reference Clarke, Guthrie and Hannun51) . However, it is well known that not all fats are created equally and various dietary fatty acids impose diverse effects throughout the body. These variable responses are also seen in Cer accumulation following the consumption of specific dietary fatty acids in rodents and humans(Reference Pinel, Rigaudiere and Laillet14,Reference Lankinen, Schwab and Erkkila21,Reference Chavez and Summers35) .
Dietary fatty acids and ceramides
SFA and ceramides
Several in vitro studies have demonstrated that SFA, specifically palmitate (C16 : 0), inhibited insulin Akt-dependent insulin signalling(Reference Pinel, Rigaudiere and Laillet14,Reference Chavez and Summers35) and up-regulated inflammatory signalling(Reference Abildgaard, Henstridge and Pedersen64) associated with Cer accumulation. SFA such as palmitate increases Cer by providing the substrate required for the up-regulation of Cer de novo synthesis(Reference Abildgaard, Henstridge and Pedersen64), which is exacerbated by the adverse effects of SFA on mitochondrial dysfunction-associated reductions in fatty acid β-oxidation(Reference Sergi, Naumovski and Heilbronn40). A HFD (33·9–60·0 % of kcal or kJ) provided to animals consisting of mainly SFA also persistently results in Cer accumulation(Reference Frangioudakis, Garrard and Raddatz9–Reference Chocian, Chabowski and Żendzian-Piotrowska11), suggesting that SFA may promote Cer production more than MUFA or PUFA.
n-3 Fatty acids and ceramides
It is long standing that n-3 LCPUFA, EPA and DHA, consumption is associated with a wide variety of health benefits, as reviewed elsewhere(Reference Shahidi and Ambigaipalan65). These beneficial effects include the prevention and treatment of MetS parameters, such as reducing circulating TAG and reducing inflammation. A vast quantity of evidence also suggests a beneficial role of n-3 LCPUFA in other metabolic diseases such as obesity and type 2 diabetes(Reference Robinson and Mazurak66); however, the effects of n-3 LCPUFA on Cer lipotoxicity have not been fully elucidated. A limited amount of research has shown that n-3 LCPUFA may reduce Cer concentrations, potentially affecting the MetS including mainly IR and inflammation. The following section examined the relationship between n-3 LCPUFA, Cer and characteristics of the MetS in vitro and in vivo including both animal and clinical research.
In vitro
Several in vitro studies, displayed in Table 1, have assessed the effect of n-3 LCPUFA, specifically DHA on Cer. Palmitate-induced Cer accumulation was significantly reversed by either 100 µm or 50 µm of DHA in murine macrophages(Reference Jin, Lu and Li15) and C2C12 muscle cells(Reference Pinel, Rigaudiere and Laillet14), respectively. Specifically, DHA attenuated C16 : 0-Cer production stimulated by palmitate in murine macrophages(Reference Jin, Lu and Li15). DHA (100 µm) has also been reported to suppress aSMase and nSMase expression and activity and reduce Cer:sphingomyelin ratio in human retinal endothelial cells(Reference Opreanu, Lydic and Reid12,Reference Opreanu, Tikhonenko and Bozack13) . In strong contrast, treatment of L6 myoblasts with palmitate plus DHA (0·4 mmol/l) did not alter total Cer concentrations compared with palmitate-treated myoblasts(Reference Lam, Hatzinikolas and Weir67).
Table 1. In vitro studies investigating EPA and DHA treatment on ceramide (Cer) and characteristics of the metabolic syndrome

HREC, human retinal endothelial cells; BSA, bovine serum albumin; LA, linoleic acid; aSMase, acid sphingomyelinase; nSMase, neutral sphingomyelinase; PA, palmitic acid; FA, fatty acid; ALA, α-linolenic acid; Akt/PKB, protein kinase B; PKC, protein kinase C; LPS, lipopolysaccharide; OA, oleic acid; POA, palmitoleic acid; MCP-1, monocyte chemoattractant protein 1; CSF3, colony-stimulating factor 3; COX-2, cyclo-oxygenase-2; SPT-1, serine palmitoyltransferase-1; Ø, no change; ↓, decrease; ↑, increase.
Among the reviewed studies, the types of cells and the concentration of DHA treatment vary, which may have led to conflicting results. As mentioned previously, Cer species are likely tissue-specific and function differently. Unfortunately, not all studies reported individual Cer species, thus it is unclear whether specific Cer species are altered following DHA treatment, despite whether total Cer concentrations remained unchanged. Interestingly, the effect of DHA on Cer synthesis pathways is also inconsistent when the dose of DHA is the same. For instance, Jin et al.(Reference Jin, Lu and Li15) reported that DHA (100 µm) suppressed serine palmitoyltransferase mRNA level in murine macrophages but did not alter aSMase activity, suggesting that DHA may reduce Cer concentrations by inhibiting de novo synthesis. Conversely, Opreanu et al.(Reference Opreanu, Lydic and Reid12) found that DHA (100 µm) inhibited both aSMase and nSMase expression and activity in human retinal endothelial cells, indicating a role for DHA in the inhibition of sphingomyelin hydrolysis. The findings further suggest that DHA may impose various effects on Cer synthesis pathways, which might be dependent on cell type.
In addition to Cer concentration reductions, DHA also prevented cytokine-induced inflammatory responses(Reference Opreanu, Lydic and Reid12,Reference Jin, Lu and Li15) , palmitate-decreased Akt activation and palmitate-induced TAG accumulation(Reference Pinel, Rigaudiere and Laillet14) in different cells. These findings imply that DHA may improve inflammation, IR and TAG lipotoxicity by modulating Cer concentrations. In contrast, Lam et al.(Reference Lam, Hatzinikolas and Weir67) did not find any alternations in Cer concentrations, net glucose uptake or TAG concentrations by DHA (0·4 mmol/l) in L6 myoblasts compared with palmitate-treated myoblasts. Furthermore, two studies reported the effect of EPA on Cer and MetS parameters(Reference Pinel, Rigaudiere and Laillet14,Reference Jin, Lu and Li15) . Although one study found that both EPA (50 µm) and DHA (50 µm) restored palmitate-induced Cer lipotoxicity and IR in C2C12 muscle cells(Reference Pinel, Rigaudiere and Laillet14), the other study indicated that DHA (100 µm) has shown to have the greatest inhibitory effect on inflammatory signalling in murine macrophages compared with EPA (100 µm)(Reference Jin, Lu and Li15). Taken together, evidence suggests DHA reduces Cer concentrations and improves IR and inflammation in various cell types, with the exception of L6 myoblasts(Reference Opreanu, Lydic and Reid12–Reference Jin, Lu and Li15,Reference Lam, Hatzinikolas and Weir67) . Whether these effects are cell-specific or related to other confounding factors is currently unknown.
In vivo
Animals
The effects of n-3 LCPUFA mainly EPA and DHA individually or together (often as fish oil) on Cer concentrations and MetS parameters in animals are summarised in Table 2. Studies using a variety of murine animal models have demonstrated that EPA and DHA supplementation reduces total Cer concentrations including various Cer species in muscle(Reference Kasbi-Chadli, Ferchaud-Roucher and Krempf16,Reference Lanza, Blachnio-Zabielska and Johnson20) , liver(Reference Kasbi-Chadli, Ferchaud-Roucher and Krempf16,Reference Dong, Zhang and Sun18) and adipose tissue(Reference Chacińska, Zabielski and Książek19). Of the reviewed studies, two of eight reported conflicting evidence with no reductions in muscle or liver Cer species(Reference Kuda, Jelenik and Jilkova68,Reference Balogun, Albert and Ford69) . Only two studies reported plasma Cer concentrations, and EPA and DHA supplementation (10 % of total fat(Reference Balogun, Albert and Ford69) or 3·05 % of total kcal or kJ(Reference Kasbi-Chadli, Ferchaud-Roucher and Krempf16) from fish oil) had little to no effect. Although reductions in individual Cer species seem to differ among tissues, EPA and DHA supplementation from fish oil (3·4 % of kcal or kJ(Reference Chacińska, Zabielski and Książek19,Reference Lanza, Blachnio-Zabielska and Johnson20) , unreported dose(Reference Taltavull, Ras and Mariné17)) tends to reduce saturated Cer species, whereas C24 : 1-Cer demonstrated conflicting results with increases in adipose tissue(Reference Chacińska, Zabielski and Książek19) and whole muscle homogenates(Reference Lanza, Blachnio-Zabielska and Johnson20), and decreases in hepatic tissues(Reference Taltavull, Ras and Mariné17).
Table 2. Animal studies investigating EPA and DHA consumption on ceramide (Cer) and characteristics of the metabolic syndrome

SAF, safflower oil; AA, arachidonic acid; HFD, high-fat diet; VLDL, very LDL; TC, total cholesterol; FA, fatty acid; FO, fish oil; SM, sphingomyelin; LCACoA; long-chain acyl CoA; IRS1, insulin receptor substrate 1; GYS1, glycogen synthase 1; HOMA, homoeostatic model assessment; DL-Hcy, DL-homocysteine; HOMA-IR, homoeostatic model assessment of insulin resistance; Ø, no change; ↓, decrease; ↑, increase.
The mechanisms whereby EPA and DHA reduce Cer concentrations have not been fully elucidated. In vitro studies indicate that DHA inhibited either serine palmitoyltransferase or SMase expression and activity, leading to reductions in Cer concentrations(Reference Opreanu, Lydic and Reid12,Reference Jin, Lu and Li15) . As previously stated, inflammatory cytokines such as TNFα promote Cer synthesis(Reference Gomez-Munoz, Presa and Gomez-Larrauri7,Reference Clarke, Guthrie and Hannun51) . Whereas DHA has anti-inflammatory properties, as it regulates transcription factor activity involved in gene expression of inflammatory markers(Reference Balogun, Albert and Ford69). Thus, a potential mechanism of action may be that DHA reduces inflammatory signalling, thereby inhibiting the feedforward mechanism of inflammation induced by Cer synthesis, resulting in Cer reductions.
As stated above, elevated Cer concentrations have been correlated with IR through their potential ability to inhibit Akt/PKB, and thus GLUT4 translocation to the plasma membrane(Reference Blachnio-Zabielska, Chacinska and Vendelbo10). Additionally, in murine animals, results from various studies consistently show that consumption of a HFD promotes increases in fasting plasma glucose, insulin and HOMA-IR scores(Reference Taltavull, Ras and Mariné17,Reference Chacińska, Zabielski and Książek19,Reference Lanza, Blachnio-Zabielska and Johnson20,Reference Kuda, Jelenik and Jilkova68) . However, the provision of EPA and DHA, mainly as fish oil, has shown to improve HFD-induced IR in these animals, paired with reductions in Cer concentrations. For instance, consuming a HFD with fish oil (3·05–3·40 % of kcal or kJ from EPA and DHA) reduced fasting blood glucose(Reference Kasbi-Chadli, Ferchaud-Roucher and Krempf16,Reference Lanza, Blachnio-Zabielska and Johnson20) , fasting plasma insulin and HOMA-IR scores(Reference Chacińska, Zabielski and Książek19) in rodents. Fish oil consumption (3·4 % of kcal or kJ from EPA and DHA) also increased mRNA expression of genes that promote insulin sensitivity including GLUT4, insulin receptor substrate-1 and glycogen synthase-1(Reference Lanza, Blachnio-Zabielska and Johnson20). Mechanistically, n-3 LCPUFA may reduce Cer concentrations and improve insulin sensitivity through the ability of these fatty acids to improve the health of mitochondria found in skeletal muscle and attenuate ER stress associated with HFD consumption(Reference Lepretti, Martucciello and Aceves45,Reference Yang, Chen and Chen70) . Specifically, n-3 LCPUFA have shown to increase mitochondrial β-oxidation, thereby decreasing ectopic lipid accumulation including Cer, thus improving mitochondrial function and insulin sensitivity(Reference Lepretti, Martucciello and Aceves45,Reference Power and Newsholme71) . In contrast, improvements in IR have occurred without alterations in muscle Cer concentrations as demonstrated by Kuda et al.(Reference Kuda, Jelenik and Jilkova68). The provision of EPA and DHA (15 % of total lipid) with a HFD was successful in improving plasma insulin in C57BL6 mice, without significantly reducing soleus muscle Cer after 20 weeks of dietary treatment. Interestingly, in obese mice with impaired glucose tolerance, the same dietary treatment also improved glucose tolerance, with a non-significant trend towards a reduction in plasma insulin concentrations. Considering the complexity of IR, Cer modulation may be one of many pathways involved in insulin signalling.
The evidence is inconsistent in regard to which specific Cer species are more important in the onset of IR; however, it is clear that acyl chain length of Cer influences the pathogenesis of IR. Despite this knowledge gap, SFA-Cer species including C18 : 0-, C20 : 0-, C22 : 0- and C24 : 0-Cer were often lowered by EPA and DHA supplementation from fish oil (3·05–3·4 % of kcal or kJ) in various tissues, occurring with improvements in IR(Reference Kasbi-Chadli, Ferchaud-Roucher and Krempf16,Reference Lanza, Blachnio-Zabielska and Johnson20) . It is clear that EPA and DHA supplementation has beneficial effects on IR by modulating total cer concentrations; however, more research is required to determine the association among EPA and DHA, individual Cer species and IR.
Currently, little research exists regarding the role of EPA and DHA consumption in reducing Cer-induced inflammation and only two of the reviewed studies evaluated markers of inflammation. Kuda et al.(Reference Kuda, Jelenik and Jilkova68) determined that C57BL6 mice consuming a HFD had low-grade inflammation as measured by macrophage infiltration surrounding adipocytes. This was attenuated with the addition of fish oil (15 % of total lipid). Lastly, an earlier study by Jolly et al.(Reference Jolly, Jiang and Chapkin72) determined that consumption of a 2 % safflower oil with 1 % EPA or 1 % DHA diet by C57BL6 mice for 10 d reduced Cer mass along with T-lymphocyte proliferative response and IL-2 secretion in splenocyte cultures. Although these studies suggest EPA and DHA consumption may reduce HFD-associated Cer and inflammation, much further investigation is required to establish this relationship in vivo.
Dyslipidaemia is a hallmark characteristic of the MetS and is often seen in combination with elevated Cer concentrations. Research utilising EPA and DHA, often as fish oil, to improve dyslipidaemia often demonstrates reductions in plasma TAG in normolipidic and dyslipidic individuals(Reference Yanai, Masui and Katsuyama73). Few studies have investigated the role of EPA and DHA on both dyslipidaemia and Cer concentrations. One of the reviewed studies(Reference Kasbi-Chadli, Ferchaud-Roucher and Krempf16) demonstrated a reduction in total and hepatic Cer, plasma TAG, TC and HDL-cholesterol, with no change to plasma very LDL-cholesterol and LDL-cholesterol concentrations following consumption of a HFD with fish oil (3·05 % kcal or kJ). Whereas Kuda et al.(Reference Kuda, Jelenik and Jilkova68) reported no changes to skeletal muscle Cer and plasma TAG with EPA and DHA (15 % of total lipid) consumption, but both NEFA and TC concentrations were reduced. In the same study, HFD-induced obese mice with impaired glucose tolerance consuming a HFD diet with fish oil saw a reduction in TAG and NEFA and no change in TC concentrations. These studies imply that n-3 LCPUFA consumption may synergistically improve both dyslipidaemia and Cer, yet research is highly limited and slightly conflicting. As dyslipidaemia and elevated Cer may both be important risk factors for CVD risk, further investigation could be highly valuable.
Human subjects
The relationship between EPA, DHA and Cer concentrations has been minimally investigated in human studies, which are displayed in Table 3. A randomised controlled parallel study suggested that consuming fatty fish four times/week (100–150 g per meal) for 8 weeks increased plasma EPA and DHA concentrations and lowered total plasma Cer concentrations, compared with those consuming lean fish or no fish(Reference Lankinen, Schwab and Erkkila21). However, there were no changes in plasma Cer concentrations in participants consuming either 8 g of fish oil per d for 7 weeks(Reference Ottestad, Hassani and Borge74), or a Mediterranean diet supplemented with extra-virgin olive oil or nuts, that emphasised foods rich with n-3 LCPUFA for 1 year(Reference Wang, Toledo and Hruby75).
Table 3. Clinical studies investigating n-3 long-chain PUFA consumption on ceramide (Cer) and characteristics of the metabolic syndrome
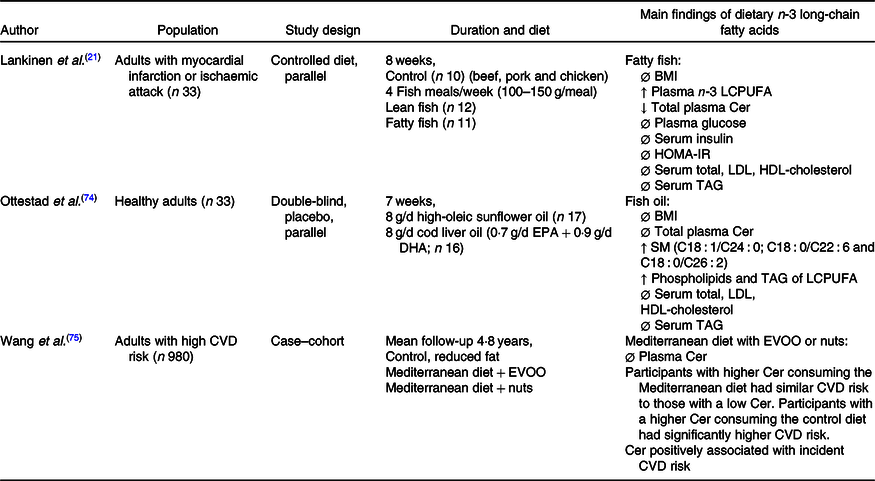
LCPUFA, long-chain PUFA; HOMA-IR, homoeostatic model assessment of insulin resistance; SM, sphingomyelin; EVOO, extra-virgin olive oil; Ø, no change; ↓, decrease; ↑, increase.
Regardless of Cer concentrations, fish or fish oil supplementation did not change serum cholesterol or TAG(Reference Lankinen, Schwab and Erkkila21,Reference Ottestad, Hassani and Borge74) . Conversely, risks of CVD were lowered in participants consuming a Mediterranean diet with either extra-virgin olive oil or nuts compared with those consuming a control diet(Reference Wang, Toledo and Hruby75). This is not surprising as a large variety of literature has praised the Mediterranean diet for its role in improving cardiovascular health.
It is difficult to conclude whether EPA and DHA can influence Cer concentrations and associated MetS in humans, due to limited data availability and differences in the characteristics of participants or doses of EPA and DHA among studies. In animal studies, EPA and DHA are provided in combination with a HFD, whereas clinical trials are not designed in this manner. Furthermore, it is not clear if Cer concentrations are changed in other tissues, such as muscle and liver following fish or fish oil consumption in humans, as research in murine animals demonstrated reductions in tissue Cer, with limited alterations in plasma. At this time, further research is required to examine the ability of EPA and DHA consumption to reduce Cer concentrations and improve parameters of the MetS in humans.
Conclusion
Overall, the evidence from in vitro and animal studies is strong regarding the ability of n-3 LCPUFA EPA and DHA to positively influence Cer concentrations when cells were treated with palmitate or animals consumed a HFD, whereas evidence in humans is greatly lacking. However, plasma Cer concentrations were the only parameter assessed in clinical trials, while evidence from animal studies suggests that EPA and DHA supplementation reduces tissue and not plasma Cer concentrations. Therefore, there is a potential for EPA and DHA to attenuate Cer accumulation in human tissues. In addition, animal studies demonstrated that a HFD with EPA and DHA reduced Cer concentrations, whereas diets utilised in clinical trials were quite opposite and focused on healthier behaviours. Whether EPA and DHA inhibit HFD-induced elevations in Cer concentrations in humans remains unknown. Among studies reviewed, changes in individual Cer species after EPA and DHA supplementation also vary among tissues. These discrepancies may be an implication that Cer with different acyl chain lengths have distinct functions and effects in various tissues.
It is evident that Cer accumulation is related to HFD consumption, IR and inflammation and often occurs concurrently with dyslipidaemia. In North America, the MetS is a highly prevalent condition that is associated with various metabolic diseases including obesity, CVD, diabetes and non-alcoholic fatty liver disease. A common recommendation for the management of these conditions is weight reduction; yet, research consistently demonstrates most (80 %) of individuals will re-gain lost weight within 1 year(Reference Mackie, Samoncha-Bonet and Tam76). By examining the role of Cer in the MetS, this may lead to the development of new therapeutic strategies to ultimately reduce the morbidity, mortality and health care burden associated with these chronic conditions, without focusing solely on weight reduction.
Although still controversial, there is a wide variety of evidence suggesting that EPA and DHA may have many health benefits including those related to the MetS and other diseases such as diabetes, cancer, mental illness and rheumatoid arthritis(Reference Shahidi and Ambigaipalan65). By highlighting the potential for EPA and DHA (fish oil) supplementation to reduce Cer concentrations associated with a HFD, and sequentially improve IR in both cell culture and murine animals, this review has contributed further to the list of potential benefits of EPA and DHA (or fish oil) supplementation. Whether EPA and DHA consumption in combination with a HFD can reduce Cer-associated inflammatory signalling or improve dyslipidaemia remains unknown and requires further investigation. Nonetheless, considering the common and relatively safe nature of fish oil supplementation, this may be a promising, simple and affordable strategy to target Cer-induced MetS.
Acknowledgements
This work was supported by Research Manitoba, Canadian Israel International Fetal Alcohol Consortium, University of Manitoba Graduate Fellowship, Manitoba Graduate Scholarship, and Mitacs Converge.
C. W. and Y. W. contributed to the literature search, analysis of the data published, manuscript writing and revisions of the article. M. S. was responsible for the supervision and revisions of the article.
The authors declare that there are no conflicts of interest.