INTRODUCTION
Estimates of global fungal diversity range from 1.5 to 5.1 million species (Blackwell, Reference Blackwell2011; Hawksworth, Reference Hawksworth2012) with the greatest diversity found in tropical lowland and montane environments (Tedersoo et al., Reference Tedersoo, Bahram, Põlme, Kõljalg, Yorou, Wijesundera and Ruiz2014). Fungi perform a vital function in terrestrial ecosystems as symbionts and decomposers of vegetation. Because of this role, a close relationship exists between plant and fungal communities (Hooper et al., Reference Hooper, Bignell, Brown, Brussaard, Dangerfield, Wall and Wardle2000; Peay et al., Reference Peay, Baraloto and Fine2013). Environmental drivers, including climate, fire regime, edaphic factors, and spatial distribution, play a role in fungal community composition (Tedersoo et al., Reference Tedersoo, Bahram, Põlme, Kõljalg, Yorou, Wijesundera and Ruiz2014). The link between fungal community and environment allows fungal remains (ascospores, conidia, and chlamydospores) preserved in sedimentary archives to be used as a proxy in reconstructing palaeoenvironments (van Geel, Reference van Geel1972).
Non-pollen palynomorphs (NPPs), which include fungal remains, zoological remains, plant fragments, and algae, were first utilised to complement fossil pollen in reconstructing palaeoenvironmental records from northern European peat bogs (van Geel, 1972, 1976, Reference van Geel1978; van Geel et al., 1981, Reference van Geel, Hallewas and Pals1983; Kuhry, Reference Kuhry1985). Combining NPPs with fossil pollen records has allowed new insight into past ecosystem functions to be gained and, consequently, has resulted in a more complete understanding of how, and why, ecosystems develop and change through time. Fungal NPPs have been used successfully to establish past trends of anthropogenic impact (van Geel et al., Reference van Geel, Buurman, Brinkkemper, Schelvis, Aptroot, van Reenen and Hakbijl2003; López-Sáez and López-Merino, Reference López-Sáez and López-Merino2007; Williams et al., Reference Williams, Gosling, Coe, Brooks and Gulliver2011) and to provide evidence of herbivore extinctions (Davis, Reference Davis1987; Gill et al., 2009, Reference Gill, McLauchlan, Skibbe, Goring, Zirbel and Williams2013), the role of herbivores in shaping landscapes (Davis and Shafer, Reference Davis and Shafer2006; Raper and Bush, Reference Raper and Bush2009; Baker et al., Reference Baker, Bhagwat and Willis2013, Reference Baker, Cornelissen, Bhagwat, Vera and Willis2016), and past mammalian behaviour (van Geel et al., Reference van Geel, Aptroot, Baittinger, Birks, Bull, Cross and Evershed2008, Reference van Geel, Guthrie, Altmann, Broekens, Bull, Gill, Jansen, Nieman and Gravendeel2011b). Despite the ability of fungal NPPs to provide unique complementary information on past environments, their use in the Neotropics has been limited because of a paucity of studies (Montoya et al., Reference Montoya, Rull and Vegas-Vilarrúbia2012), and within the Andes, their use is restricted to Venezuela (Rull and Vegas-Vilarrúbia, 1998, Reference Rull and Vegas-Vilarrúbia1999; Rull et al., Reference Rull, López-Sáez and Vegas-Vilarrúbia2008; Montoya et al., 2010, Reference Montoya, Rull and Vegas-Vilarrúbia2012) and Colombia (Hooghiemstra, Reference Hooghiemstra1984; Kuhry, Reference Kuhry1988; Grabandt, Reference Grabandt1990).
The generally diverse nature of ecosystems and landscapes in the tropics has long been discussed (Wallace, Reference Wallace1878; MacArthur, Reference MacArthur1969; Connell, Reference Connell1978; Stevens, Reference Stevens1989) and has led to the tropical Andean region being identified as containing some of the most biodiverse ecosystems on the planet (Myers et al., Reference Myers, Mittermeier, Mittermeier, da Fonseca and Kent2000). Within the Andean biodiversity hot spot, the increase in species diversity within midelevation Andean tropical forests has been the focus of particular attention (Terborgh, Reference Terborgh1977; Graham, Reference Graham1983; Gentry, Reference Gentry1988). The reason for high biodiversity in the Andes has been variously attributed to the region’s heterogeneous nature, dynamic environment, and high precipitation (Killeen et al., Reference Killeen, Douglas, Consiglio, Jørgensen and Mejia2007; Hoorn et al., Reference Hoorn, Wesselingh, ter Steege, Bermudez, Mora, Sevink and Sanmartín2010). However, questions remain unanswered about the long-term ecological and environmental processes operating within these biodiverse Andean forests. The development of fungal NPPs as a proxy within the eastern Andean montane forest provides an opportunity to extract a new type of information on long-term ecological and environmental states and processes from the fossil record.
Recently, modern assemblage-environment calibration data sets for two biological proxies have been constructed for the Andes region: (1) pollen (Rull, Reference Rull2006; Moscol-Olivera et al., Reference Moscol-Olivera, Duivenvoorden and Hooghiemstra2009; Flantua et al., Reference Flantua, Hooghiemstra, Grimm, Behling, Bush, González-Arango and Gosling2015) and (2) chironomids (Matthews-Bird et al., Reference Matthews-Bird, Brooks, Holden, Montoya and Gosling2016a, Reference Matthews‐Bird, Gosling, Coe, Bush, Mayle, Axford and Brooks2016b). These quantitative methodologies use modern assemblage data and environmental variables to develop transfer functions with the aim of reconstructing past climatic conditions (Juggins and Birks, Reference Juggins and Birks2012). The modern calibration approach could be adapted to qualitatively investigate the autoecology of fungal NPPs along environmental gradients to provide new insight into the principal environmental controls governing specific fungal morphotypes. However, equivalent studies on the eastern Andean flank are hindered by the dynamic nature of the landscape, which limits the number of suitable locations (e.g., lakes, swamps, and bogs) within midelevation montane forests from which proxies, including fungal remains, can be recovered. Alternative sources of samples, such as surface soil and moss polsters, have been variously used to characterise the fungal NPPs of modern environments (Montoya et al., Reference Montoya, Rull and van Geel2010). However, comparisons of NPP assemblages between different sample types (e.g., lake sediments vs. soil samples) is not optimal because of large differences induced by the local signal and limited dispersal potential of many fungal remains (i.e., a strong “sample effect”) (Wilmshurst and McGlone, Reference Wilmshurst and McGlone2005; Montoya et al., Reference Montoya, Rull and van Geel2010).
To overcome the challenge presented by an absence of suitable depositional environments from which to recover a modern calibration data set for NPPs, we have developed an alternative approach that relates fungal NPP assemblages to independent environmental proxies in sedimentary archives. In this article, we use palaeoenvironmental proxies of forest cover (forest pollen), available moisture (aquatic remains), regional fire regime (microcharcoal), and sediment composition (organic carbon) to provide environmental gradients against which to constrain the autoecological characteristics of fungal NPPs. Using sedimentary archives as a training data set allows us to explore assemblage shifts along longer environmental gradients than would be possible using just modern samples in the Andes. Through using the sedimentary archives, we significantly increase the number of samples from the optimal depositional environments (lakes, swamps, and bogs) and reduce the “sample effect” that can bias comparison with the fossil record. Furthermore, using sedimentary archives prior to, and after, the arrival of humans to the continent allows us to explore how fungal NPP assemblages developed in landscapes without the influence of people. Our novel approach will allow additional information about ecological and environmental processes to be incorporated into future palaeoenvironmental studies.
THE EASTERN ANDEAN FLANK (ECUADOR)
Landscape
The changing topography of the eastern Andean flank is the principal variable controlling vegetation composition and regulating air temperature, precipitation, and cloud cover (Harling, Reference Harling1979; Graham, Reference Graham2009). Uplift of the Andes began during the Miocene, with the collision of the Nazca and South American plates (Allmendinger et al., Reference Allmendinger, Jordan, Kay and Isacks1997), followed by accelerated uplift and widespread volcanic activity during the late Pliocene to early Pleistocene (Coltorti and Ollier, Reference Coltorti and Ollier2000). Throughout the Holocene, Ecuador has experienced a high degree of volcanism with at least 20 active volcanos (Hall et al., Reference Hall, Samaniego, Le Pennec and Johnson2008). This complex geologic history has produced a variety of source rocks that give rise to the diverse range of soil compositions found within the Andes (Neill, Reference Neill1999a; Vera, Reference Vera2013).
Vegetation
Andean montane forests are composed of a narrow band (~30 km) of tropical vegetation covering an altitudinal range of ~2300 m (1300–3600 metres above sea level [m asl]) (Neill, Reference Neill1999b). Montane forests can be split into three vegetation communities: lower montane forest (1300–2000 m asl), cloud forest (2000–2900 m asl), and upper montane forest (2900–3600 m asl) (Sierra, Reference Sierra1999). Today, the study areas fall within the cloud forest type vegetation community (Fig. 1). The cloud forest in this region is composed of a mix of Andean and lowland forest elements including Alnus (Betulaceae), Arecaceae, Fabaceae, Hedyosmum (Chloranthaceae), Lauraceae, Melastomataceae, Meliaceae, Moraceae, Rubiaceae, Solanaceae, and Weinmannia (Cunoniaceae) (Harling, Reference Harling1979; Gentry, Reference Gentry1995; Neill and Palacios, Reference Neill and Palacios1997; Neill, Reference Neill1999b; Valencia et al., Reference Valencia, Cerón, Palacios and Sierra1999). Forest canopy within the cloud forest reaches 15–25 m, and trees are covered by abundant epiphytes including mosses, lichens, ferns, Bromeliaceae, Araceae, and Orchidaceae (Webster, Reference Webster1995; Neill and Palacios, Reference Neill and Palacios1997). Human impact and forest clearance within the cloud forest occurs primarily near the river valley floor. Human activity and deforestation is characterised in the vegetation by an increase in herbaceous taxa, disturbance indicators such as Cecropia (Urticaceae) and large patches of pioneers such as Chusquea (Poaceae) and Gunnera (Gunneraceae), which also occur on steep-sided slopes following frequent landslides (Stern, Reference Stern1995).
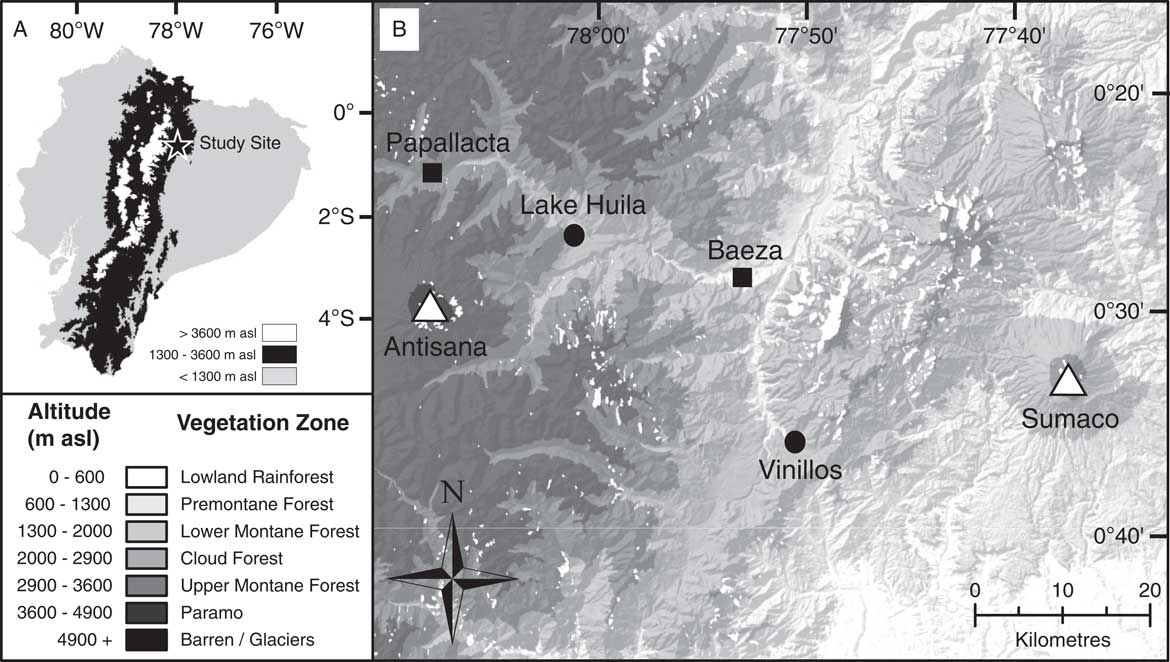
Figure 1 Map showing position of study site and sample locations. (A) Map of Ecuador. Regions coloured black indicate an altitude of between 1300 and 3600 metres above sea level (m asl) corresponding to Andean montane forest vegetation. (B) Map of study location with altitudinal gradient related to vegetation zones as described in the “Introduction” and corresponding to Sierra (Reference Sierra1999). Circles indicate sample locations; squares, local population centres; and triangles, volcanic centres.
Climate
Precipitation on the eastern Andean flank is principally controlled by the South American summer monsoon (Vuille et al., Reference Vuille, Bradley and Keimig2000; Cook, Reference Cook2009). Mean annual precipitation varies with altitude, with approximately 2500 mm of rainfall per year at 2000 m asl (Neill and Jørgensen, Reference Neill and Jørgensen1999). High levels of orographic rainfall and semipermanent ground-level clouds lead to reduced seasonality and persistent moist conditions (Harling, Reference Harling1979). Mean annual temperature ranges from 16–20°C at approximately 2000 m asl (Galeas and Guevara, Reference Galeas and Guevara2012), with an adiabatic lapse rate of ~0.56°C per 100 m (Bush et al., Reference Bush, Silman and Urrego2004). Temperatures remain stable throughout the year; however, diurnal changes in temperature of up to 20°C can occur at higher elevations, acting as a much more significant control on vegetation distribution than seasonal changes in temperature (Neill and Jørgensen, Reference Neill and Jørgensen1999).
STUDY SITES AND SAMPLES
The study area is situated in the Quijos Canton in the Napo Province of Ecuador near the town of Baeza (Fig. 1). Two sedimentary archives were recovered from Lake Huila (hereafter referred to as Huila) and an exposed cliff section near the town of Cosanga (hereafter referred to as Vinillos). The Huila and Vinillos sediments were selected for development of a fungal NPP training data set because they allowed the fungal NPP assemblages to be constrained against environmental variables when humans were present (Huila), and absent (Vinillos), from the landscape—that is, the sites are securely radiocarbon dated to before, and after, the arrival of humans in South America ca. 12,000 yr ago (Rademaker et al., Reference Rademaker, Hodgins, Moore, Zarrillo, Miller, Bromley, Leach, Reid, Álvarez and Sandweiss2014).
Huila
Huila (00°25.39′S, 78°01.06′W) is situated at 2608 m asl on a raised plateau adjacent to the Río Quijos (Fig. 1). The lake consists of a shallow (~1.10 m deep) body of water approximately 800 m2, within a ~4000 m2 closed basin, with no obvious inflow. The lake is situated in a cleared pasture used for cattle and horse grazing. The catchment is dominated by Poaceae and Cyperaceae, with the surrounding region consisting primarily of pastures and secondary forest. In 2013, a 2.11 m sediment core was recovered from the centre of the lake from a floating platform using a Colinvaux-Vohnout modified Livingstone piston corer (Livingstone, Reference Livingstone1955; Colinvaux et al., Reference Colinvaux, de Oliveira and Patiño1999). Once recovered, the cores were returned to the Open University laboratory intact for storage in a cold room (~4°C). Radiocarbon dating has shown that the Huila sediments sampled (upper 49 cm) were deposited during the last millennium (Table 1).
Table 1 Radiocarbon (accelerator mass spectrometry) ages obtained from palynomorph residues from Huila and Vinillos. The dated sample from Huila is from the base of the sediment analysed (i.e., all the samples presented here are younger than the age of this sample). The dated sample from Vinillos is from the upper part of the sedimentary section (i.e.,, all the samples presented here are older than the age indicated). Original dates calibrated in OxCal 4.2.4 (Bronk Ramsey et al., Reference Bronk Ramsey, Scott and van der Plicht2013) using IntCal13 atmospheric curve (Reimer et al., Reference Reimer, Bard, Bayliss, Beck, Blackwell, Bronk Ramsey and Buck2013).

Vinillos
The Vinillos section (00°36.04′S, 77°50.81′W) is located at 2090 m asl, approximately 3 km southeast of the town of Cosanga (Fig. 1). The Vinillos section consists of an outcrop of exposed sediment revealed during the construction of the Troncal Amazónica (E45), a road running parallel to the Río Cosanga. Vinillos is today situated at the boundary between the lower montane forest and cloud forest, with vegetation at the site dominated by disturbance indicators (Cecropia, Gunnera, and Chusquea). In 2012, 50 discrete sediment samples were taken systematically through the dark-brown organic beds at 5 cm intervals along with individual bulk samples from four volcanic tephra layers. Samples were placed in ziplock bags and transported to the Open University to be stored in a cold room (~4°C). Lithologic information was recorded from individual samples in the laboratory. Radiocarbon dating indicates that the Vinillos sediments were deposited prior to ca. 42,000 yr ago (Table 1).
METHODS
A total of 52 samples were examined for fungal NPP remains selected from the two sedimentary archives: Huila (26 samples, designated sample codes starting with “H”) and Vinillos (26 samples, designated sample codes starting with “V”). Four independent proxies were also investigated in order to determine specific environmental variables. Three environmental data sets were obtained from the same samples as the NPP assemblages: (1) forest pollen (forest cover), (2) aquatic remains (available moisture), and (3) microcharcoal (regional fire regime). One data set was obtained from additional, equivalent, samples: organic carbon (sediment composition).
Fungal non-pollen palynomorphs
Samples were processed using standard protocols and included the addition of an exotic marker for the calculation of concentrations (Stockmarr, Reference Stockmarr1971): University of Lund, Lycopodium tablet batch #124961 containing an average of 12,542±931 spores per tablet. Sediment samples of 1 cm3 were sieved at 180 µm and processed using KOH, HCl, HF, and acetolysis (Faegri and Iversen, Reference Faegri and Iversen1989; Moore et al., Reference Moore, Webb and Collinson1991). Eleven samples containing high levels of silica (V90, V160, V210, V250, and H31 to 49) were resampled and processed using density separation (Bromoform; 2 mol) instead of HF to improve visibility of palynomorphs. Previous studies indicate that samples processed using HF and density separation are directly comparable (Campbell et al., Reference Campbell, Fletcher, Hughes and Shuttleworth2016). All sample residues were mounted on glass slides in glycerol and counted on a Nikon Eclipse 50i microscope at 400× and 1000× magnification. NPP remains were counted in conjunction with fossil pollen and are expressed as a percentage relative to the total terrestrial pollen sum—that is, any taxa with a potential aquatic affinity, such as Cyperaceae and Myriophyllum, are excluded from the terrestrial pollen sum. NPPs were counted until a minimum of 300 terrestrial pollen grains had been counted. Deviation from this methodology occurred in sample V5 where fungal spore type HdV-123 was overrepresented, accounting for 698.3% of the pollen sum; here the NPP count was increased until a minimum of 100 non-HdV-123 fungal spores had been counted. Identification of NPPs was undertaken using the available literature (van Geel, Reference van Geel1978; van Geel et al., Reference van Geel, Bohncke and Dee1981, 1983, Reference van Geel, Coope and van Der Hammen1989, Reference van Geel, Buurman, Brinkkemper, Schelvis, Aptroot, van Reenen and Hakbijl2003, Reference van Geel, Gelorini, Lyaruu, Aptroot, Rucina, Marchant, Damsté and Verschuren2011a; Bakker and van Smeerdijk, Reference Bakker and van Smeerdijk1982; Hooghiemstra, Reference Hooghiemstra1984; van Smeerdijk, Reference van Smeerdijk1989; Rull and Vegas-Vilarrúbia, Reference Rull and Vegas-Vilarrúbia1999; van Geel and Aptroot, Reference van Geel and Aptroot2006; Rull et al., Reference Rull, López-Sáez and Vegas-Vilarrúbia2008; Cugny et al., Reference Cugny, Mazier and Galop2010; Montoya et al., 2010, Reference Montoya, Rull and Vegas-Vilarrúbia2012; Gelorini et al., Reference Gelorini, Verbeken, van Geel, Cocquyt and Verschuren2011; López-Vila et al., Reference López-Vila, Montoya, Canellas-Bolta and Rull2014), types were recorded, and their known ecological affinities were described (Supplementary Appendix A). New NPP morphotypes encountered were assigned a unique code with the designation OU (The Open University), and their morphological characteristics were described based on a minimum of five examples (Supplementary Appendix B). New morphotypes, where fewer than five examples could be found, were not described or included in the diagrams.
Environmental variables
Four environmental variables were parameterised using independent proxies: (1) forest cover (forest pollen), (2) available moisture (aquatic remains), (3) regional fire regime (microcharcoal), and (4) sediment composition (organic carbon).
From the complete terrestrial fossil pollen data sets, four pollen types (Alnus, Weinmannia, Hedyosmum, and Melastomataceae) were selected as representatives of an Andean cloud forest vegetation community based on their occurrence in (1) modern vegetation surveys (Gentry, Reference Gentry1995; Jørgensen and León-Yánez, Reference Jørgensen and León-Yánez1999), (2) modern pollen rain (Weng et al., Reference Weng, Bush and Silman2004; Rull, Reference Rull2006; Cárdenas et al., Reference Cárdenas, Gosling, Pennington, Poole, Sherlock and Mothes2014), and (3) late Quaternary sedimentary archives (Hooghiemstra and van der Hammen, Reference Hooghiemstra and van der Hammen1993; Colinvaux et al., Reference Colinvaux, Bush, Steinitz-Kannan and Miller1997; Urrego et al., Reference Urrego, Silman and Bush2005; Niemann and Behling, Reference Niemann and Behling2008; González-Carranza et al., Reference González-Carranza, Hooghiemstra and Vélez2012; Cárdenas et al., Reference Cárdenas, Gosling, Pennington, Poole, Sherlock and Mothes2014). This group of Andean cloud forest pollen taxa is referred to collectively from here on as “forest pollen.”
Aquatic remains consisting of pollen and spores of aquatic taxa (Cyperaceae, Myriophyllum, and Isoetes), algae (Botryococcus, Spirogyra, Concentricystis, Mougeotia, Zygnema, Debarya, HdV-166B, and HdV-333), and the remains of aquatic organisms (Chironomidae head capsules, HdV-179 and HdV-353B) were counted in conjunction with the terrestrial pollen. All aquatic remains were combined and expressed as a percentage relative to the total terrestrial pollen sum to provide information on the available moisture.
The presence of microcharcoal on the palynomorph slides was used to identify periods of regional fire activity (Whitlock and Larsen, Reference Whitlock and Larsen2001). Samples were examined for microscopic charcoal fragments (5–100 µm) using a microscope at 200× magnification. Counts were then expressed as concentration of particles per cubic centimeter (Whitlock and Larsen, Reference Whitlock and Larsen2001).
Sediment samples of ~2 cm3 were taken and processed for loss-on-ignition analysis at depths corresponding to the palynomorph and charcoal samples. Sediment was dried at 40°C for up to 5 days to remove moisture, followed by a controlled burn at 550°C for 4 hours to remove organic carbon (Heiri et al., Reference Heiri, Lotter and Lemcke2001). Weight loss was then converted to a percentage of the dry weight to determine the proportion of organic carbon within the sediment.
Zonation
Samples were first ordered sequentially based on the percentage of forest pollen representatives from the sample (i.e., along an environmental gradient of forest cover) (Fig. 2). Zonation was based on NPP percentage abundance data of all morphotypes that occur in more than one sample and with an abundance of >2% in at least one sample. Zones were then established using “optimal splitting by information content” (OSIC) using the program psimpoll (Bennett, Reference Bennett2008), and the statistical significance of zones was tested using the broken stick method (Bennett, Reference Bennett1996; Fig. 3). All data were plotted using the programme C2 (Juggins, Reference Juggins2007).
Data analysis
Canonical correspondence analysis (CCA) (ter Braak, Reference ter Braak1986) was performed to investigate the influence of environmental variables (forest cover, available moisture, regional fire regime, and sediment composition) on the distribution of fungal NPPs. CCA analysis was performed on NPP percentage data after a square-root transformation had been applied. The purpose of the square-root transformation was to stabilise variance within the assemblages and compensate for the occurrence of overrepresented taxa that can occur with proxies that have a low dispersal potential, such as NPPs (Wilmshurst and McGlone, Reference Wilmshurst and McGlone2005). Within the CCA, fungal NPP data were plotted against untransformed environmental data from forest pollen, aquatic remains, microcharcoal, and organic carbon (Fig. 4). Analysis of variance was used to test the significance of the variation in fungal NPPs explained by the environmental variables. CCA was performed in R (R Development Core Team, 2015) using the package vegan (Oksanen et al., Reference Oksanen, Blanchet, Kindt, Legendre, Minchin, O’Hara and Simpson2016).
RESULTS
Fungal non-pollen palynomorphs
A total of 52 samples were analysed, but 3 samples were determined to be barren of fungal NPPs (concentration <2000 spores per cm3 in samples V90, V135, and V160) and were consequently excluded from subsequent analysis. Analysis of fungal NPPs within the remaining 49 samples identified 54 distinct fungal morphological types, of which 51 met the requirements of the persistence (>1 sample) and presence (>2% abundance in one sample) filters. Twenty-nine morphotypes were assigned to previously described types and are recorded in Supplementary Appendix A along with pertinent ecological information. Twenty-five previously undescribed morphotypes were recorded, photographed (Fig. 5), and described in Supplementary Appendix B.
OSIC analysis of fungal NPP percentage data established three distinct zones that have been described based on their position along the forest cover environmental gradient: (1) low forest cover (<8% forest pollen relative to terrestrial pollen sum), (2) medium forest cover (8–32% forest pollen), and (3) high forest cover (>32% forest pollen). The boundary between the low forest cover and medium forest cover zones was found to be statistically significant based on the broken stick method (Fig. 3).
Low forest cover zone (nine samples)
Within the low forest cover zone, fungal NPP concentrations are low, with only two samples having greater than 10,000 spores per cm3 (H23 and H25). Samples are characterized by relatively high abundances of HdV-201 (0–18.6%), IBB-16 (0–8.2%), HdV-16A (0–5.5%), OU-102 (0–7.5%), and Neurospora (0–2.2%). It is worth noting that morphotype OU-102 only occurs within the low forest cover zone and is present in six of the nine samples.
Medium forest cover zone (22 samples)
Within the medium forest cover zone, NPP concentrations fluctuate (~3400–570,000 spores per cm3; mean 180,000 spores per cm3), although it is worth noting that only 3 of the 22 samples (H11, H21, and H22) have high concentrations (>500,000 percm3). Samples are characterised by high abundances of Xylariaceae (0.3–100.6%), Cercophora-type 1 (0–80.1%), HdV-123 (0–698.3%), HdV-495 (0–19.0%), HdV-16A (0–54.6%), and IBB-259 (0–12.9%). It is also worth noting that OU-100 (0–12.4%), and OU-104 (0–4.3%) are most abundant within the medium forest zone.
High forest cover (18 samples)
Within the high forest cover zone, fungal NPP concentrations range from ~10,000 to 1,000,000 spores per cm3. The most persistent and abundant fungal types include HdV-123 (0–68.7%), Xylariaceae (0.1–20.7%), HdV-495 (0–11.4%), and IBB-259 (0.2–36.3%). It is also worth noting that OU-108 (0–75.3%) is more abundant in the high forest cover zone than any other zone, and that it reaches exceptionally high abundances in one sample (V25).
Environmental variables
The four independent indicators of environmental gradients (forest pollen, aquatic remains, microcharcoal, and organic carbon) have been plotted against increasing forest pollen percentage data (Fig. 2). Forest pollen components (Alnus, Hedyosmum, Weinmannia, and Melastomataceae) occur over a gradient of 2.5–63.5% of the total terrestrial pollen sum. Aquatic remains in the low and medium forest cover zones fluctuate widely in abundance (low=17–130% of the total terrestrial pollen sum, mean 69%; medium=0.5–131%, mean 49%), whereas aquatic remains in the high forest pollen zone have less variance (0.8–56%) and a lower mean (14.5%). Microcharcoal is most abundant within the zone of low forest cover where it occurs in concentrations of up to ~3,300,000 fragments per cm3 (sample H43). In the zones of medium and high forest cover, microcharcoal concentrations progressively decrease (medium=<1,000,000 fragments per cm3; high=<200,000 fragments per cm3). The organic content of the sediment varies along the entire forest cover gradient with similar mean values (low=29%; medium=36%; high=28%) and a range of 3.2–92.0% organic carbon.

Figure 2 Palaeoenvironmental proxies from which environmental variables have been inferred. Samples are ordered based on increasing percentage of forest pollen. Forest pollen is based on the combined percentage of Alnus, Weinmannia, Hedyosmum, and Melastomataceae in the sample relative to the total terrestrial pollen sum. Aquatic remains relate to the percentage abundance of total aquatic remains relative to the terrestrial pollen sum. Microcharcoal plotted as fragments per cubic centimetre of sediment. Organic carbon refers to the percent organic carbon loss during loss on ignition at 550°C.
Canonical correspondence analysis
CCA of the transformed fungal NPP data and the four environmental variables were plotted (Fig. 4). The explanatory (environmental) variables account for ~40% (constrained inertia=0.8187) of the variance in the fungal NPP percentage data (total inertia=2.0438). The first two CCA axes account for 90% of the explained variance (eigenvalues: CCA1=0.4235; CCA2=0.3198). The closest relationship of an environmental variable and a CCA axis is found between the aquatic remains and the positive side of axis 2. The length attained by microcharcoal points to a degree of affinity with the negative values of axis 1. Forest pollen and organic carbon do not present a clear relationship with either of the axes based on the angles formed in the plot, but the direction of the trends suggests an inverse relationship between forest pollen and microcharcoal (Fig. 4).
Fungal NPPs cluster into three broad groups. Morphotypes such as Neurospora, HdV-201, and IBB-16 covary with the microcharcoal gradient. Anthostomella fuegiana, TM-211, and HdV-495 covary with the forest pollen gradient, and Podospora, Gaeumannomyces/Clasteropspriom caricinum, conidiophores, UG-1194, and IBB-25 covary with the aquatic remains and organic carbon gradients. Fungal NPPs that plot in the centre of the ordination are not strongly controlled by any one of the tested environmental variables (e.g., Cercophora-type 2 and HdV-1058) or are ubiquitous throughout the samples (e.g., Xylariaceae-type and HdV-16A).
DISCUSSION
Variance in fungal non-pollen palynomorphs along a gradient of forest cover in the Andes
NPPs show a statistically significant variation along the forest cover gradient sampled (2.5–63.5%; Fig. 3). The occurrence of distinct NPP taxa assemblages in the three forest cover zones suggests that NPP assemblages could be used as indicators of forest cover on the eastern Andean flank.
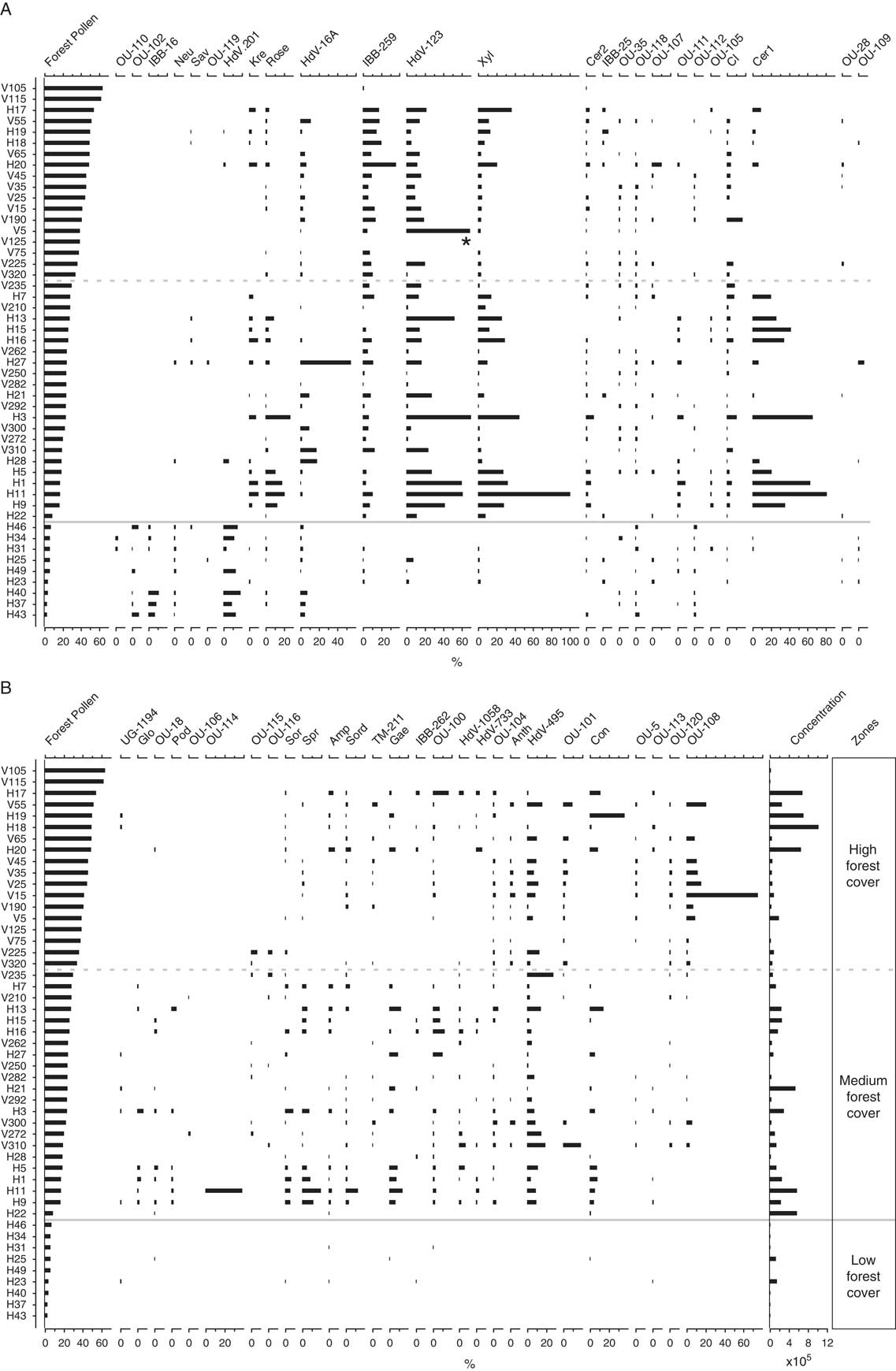
Figure 3 (A, B) Fungal non-pollen palynomorph (NPP) assemblage data for all taxa occurring at >2% abundance in >1 sample. Fungal NPP percentages are calculated relative to the total terrestrial pollen sum. Samples are ordered based on an increasing percentage of forest pollen. Asterisk (*) in panel A indicates that HdV-123 in sample V5 occurs at 698.3%. See Table 2 for definitions of abbreviations.
Table 2 Abbreviations of taxa used in non-pollen palynomorph diagram (Fig. 3) and canonical correspondence analysis diagram (Fig. 4).
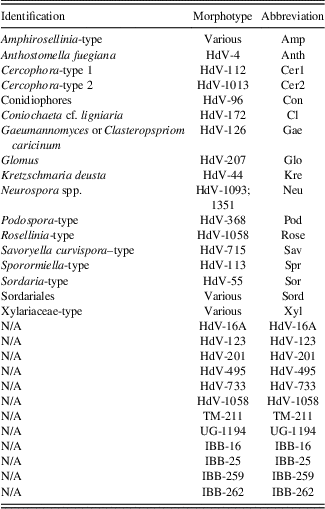
Note: N/A refers to NPP morphotypes with no know affinity.
The NPP assemblage in the low forest cover zone is characterised by an abundance of HdV-201, IBB-16, Neurospora, and HdV-16A. Interestingly, the two samples that do not contain the most abundant NPP taxa in the low forest cover zone (HdV-201) are also unusual in that they have a sediment organic carbon ~60% greater than any other sample (Fig. 2). The link between HdV-201 and organic carbon suggests that perhaps local factors that limit organic input into the sediment are important for HdV-201. The presence of the carbonicolous fungal NPP Neurospora within the low forest cover zone in conjunction with high microcharcoal indicate that regional and local fires likely occurred within areas of low forest cover. The high proportion of aquatic remains, here predominantly the semiaquatic pollen Cyperaceae, might suggest a marsh-type depositional environment. However, the low organic carbon content of the sediment indicates a low productivity environment. Additional fungal NPPs from the low forest pollen zone include types indicative of more open environments (Fig. 3). HdV-201 occurs on helophytes in drying pools (van Geel et al., Reference van Geel, Coope and van Der Hammen1989), and IBB-16 within páramo (high Andean grasslands) vegetation (Montoya et al., Reference Montoya, Rull and van Geel2010). This combination of environmental conditions and fungal NPP morphotypes suggests an open depositional environment, with forest pollen input probably from extralocal sources. All samples from the low forest pollen zone belong to post–human arrival Huila sediments. The low abundance of forest pollen, low organic carbon content, and regional to local fire activity can be interpreted as indicative of landscapes degraded by humans based on comparisons with similar findings in modern studies of managed areas elsewhere in the Andes (Rull, Reference Rull2006; Moscol-Olivera et al., Reference Moscol-Olivera, Duivenvoorden and Hooghiemstra2009).
The statistically significant zonation at ~8% forest cover occurs where the NPP assemblage changes to one containing an increase of morphotypes associated with decaying plant remains and high-elevation grassland (páramo) (Fig. 3). The zone of medium forest cover contains ligneous and saprophytic morphotypes such as Cercophora-type 1, Xylariaceae, Rosellinia-type, Kretzschmaria deusta, Amphirosellinia, and Coniochaeta cf. ligniaria (van Geel et al., Reference van Geel, Buurman, Brinkkemper, Schelvis, Aptroot, van Reenen and Hakbijl2003; van Geel and Aptroot, Reference van Geel and Aptroot2006) indicative of an increase in woody taxa. Morphotypes HdV-123, HdV-495, HdV-733, and Gaeumannomyces/Clasteropspriom caricinum previously identified in marsh-type environments are also abundant (van Geel, Reference van Geel1978; van Geel et al., Reference van Geel, Bohncke and Dee1981; Bakker and van Smeerdijk, Reference Bakker and van Smeerdijk1982). The occurrence of these types in conjunction with the mycorrhizal fungi Glomus, an indicator of increased basin erosion, and the coprophilous fungi Sporormiella and Podospora suggests that the medium forest cover zone has been subjected to a degree of disturbance by herbivores and basin erosion. The transition from the medium to high forest cover zone is not statistically significant; however, some changes in the NPP assemblage can be observed. In the medium forest cover zone, obligate coprophilous fungi (Sporormiella and Podospora) and occasional coprophilous fungi (Cercophora-type 1 and Sordaria-type) are all at their most abundant along the forest gradient, suggesting a relationship between herbivores and medium forest cover. Furthermore, in the high forest cover zone, Anthostomella fuegiana, an ascospore associated with the host plants Cyperaceae and Juncaceae (van Geel, Reference van Geel1978), and new morphotypes OU-5, OU-101, OU-108, and OU-120 are more prevalent than at any other point on the gradient. The reduction in microcharcoal associated with the zone of high forest cover and the loss or reduction in the carbonicolous fungal spore Neurospora, disturbance indicator Glomus, and coprophilous fungi suggest an environment with little evidence of disturbance. Therefore, our data suggest that the fungal NPP assemblage formed by Neurospora, HdV-201, IBB-16, OU-102, and OU-110 could be tentatively used as a proxy for open environments (or degraded lands) within the montane forests of the eastern Andean flank.
Fungal NPP assemblages and environmental variables
The variables tested in this study form three groups of fungal NPPs along environmental gradients related to regional fire regime (microcharcoal), depositional environment (organic carbon and aquatic remains), and forest cover (forest pollen) (Fig. 4). Together, fire, depositional environment, and forest cover explain ~40% of the variance in the NPP data set, indicating that environmental variables not parameterised here (e.g., climate, human impact or disturbance, and plant-fungal interactions) have a strong influence on assemblage composition.

Figure 4 Canonical correspondence analysis (CCA) of non-pollen palynomorph (NPP) morphotypes and environmental variables. NPP morphotypes (black dots) and samples (grey dots) are plotted against palaeoenvironmental proxies of environmental variables (black arrows): forest pollen (forest cover), aquatic remains (available moisture), microcharcoal (regional fire regime), and organic carbon (sediment composition). See Table 2 for definitions of abbreviations.
Fire regime
Five NPP morphotypes have been identified as covarying with regional fire regime (microcharcoal) on the eastern Andean flank (upper left portion of Fig. 4); of these, three have been previously described, and two are newly described in this study. Three previously described morphotypes that covary with regional fire regime are Neurospora, HdV-201, and IBB-16. Neurospora commonly occurs in the tropics on the surface of charred wood remains and is consequently seen as directly indicative of the presence of local fire events (Jacobson et al., Reference Jacobson, Dettman, Adams, Boesl, Sultana, Roenneberg and Merrow2006). In this study, the strong affinity of Neurospora with fire regime supports the interpretation of this NPP type with burning. The two other NPPs associated with fire in this study (HdV-201 and IBB-16) have been previously linked to helophyte remains in drier microhabitats (van Geel et al., Reference van Geel, Coope and van Der Hammen1989) and páramo environments (Montoya et al., Reference Montoya, Rull and van Geel2010), respectively. The association between the sole carbonicolous fungal NPP (Neurospora) and morphotypes indicative of a more open habitat (HdV-201 and IBB-16) fits with the inverse relationship between the fire regime and forest cover gradients in the CCA analysis (Fig. 4). The newly described morphotypes adjacent to the microcharcoal gradient (OU-102 and OU-110) occur only within seven samples (H31 to H49), which contain seven of the eight highest concentrations of microcharcoal in the whole data set (Fig. 3). These seven samples also have low forest cover (<8%) and organic content (<17%). The fungal NPP assemblage of Neurospora, HdV-201, IBB-16, OU-102, and OU-110 is therefore suggested to be broadly indicative of elevated fire regimes and appears in semiopen environments, with poor or degraded soils.
Depositional environment
Aquatic remains (moisture availability) and organic carbon (sediment composition) covary with the greatest abundance of NPP morphotypes (upper right region of the ordination in Fig. 4). The taxa comprise a number of fungal NPP morphotypes with a variety of autoecological characteristics suggested to relate to the dynamic and heterogeneous nature of eastern Andean montane landscape. Morphotypes IBB-262 and IBB-25 have previously been identified from within the cloud forest and páramo environments of Colombia and Venezuela (Hooghiemstra, Reference Hooghiemstra1984; Montoya et al., Reference Montoya, Rull and van Geel2010). Amphirosellinia sp. and Kretzschmaria deusta are parasitic tree fungi often associated with rotting wood (Ju et al., Reference Ju, Rogers, Hsieh and Vasilyeva2004; Innes et al., Reference Innes, Blackford and Simmons2010). Kretzschmaria deusta occurs on common Andean montane forest taxa such as Alnus sp. and Ilex sp. (van Geel and Andersen, Reference van Geel and Andersen1988), and increased abundance has been related to severe rainstorms during the Holocene (van Geel et al., Reference van Geel, Engels, Martin-Puertas and Brauer2013). Morphotype HdV-733 and Savoryella curvispora occur in mesotrophic marshes dominated by wetland grasses (Bakker and van Smeerdijk, Reference Bakker and van Smeerdijk1982), of which Gaeumannomyces sp./Clasteropspriom caricinum are the hyphopodium of leaf parasites on Cyperaceae. The coprophilous fungi Podospora and Sporormiella also occur along the organic carbon gradient suggesting a possible link between the presence of herbivores and increased organic carbon. The newly described morphotypes (OU-18, OU-105, OU-113, and OU-119) that occur in this region of the CCA are from samples that exhibit high organic content (41–75%), but variable forest cover (3–54%). Fungal NPP assemblages containing Cercophora-type 1, Amphirosellinia sp., Kretzschmaria deusta, Gaeumannomyces sp./Clasteropspriom caricinum, IBB-262, IBB-25, UG-1194, OU-18, OU-105, OU-113, and OU-119 are therefore suggested to be broadly indicative of organic-rich depositional environments; however, it is likely that multiple factors are responsible for the elevated organic levels (e.g., elevated sediment organic carbon content could result from high forest cover and/or the presence of herbivores).
Forest cover
The forest pollen gradient covaries closely with two previously identified NPP morphotypes (lower right region in Fig. 4). HdV-495 has been identified within the Andean montane forest and páramo of Venezuela (Montoya et al., Reference Montoya, Rull and van Geel2010), and IBB-259 from within montane settings in Europe (López-Vila et al., Reference López-Vila, Montoya, Canellas-Bolta and Rull2014). The lack of NPP morphotypes closely associated with the forest pollen gradient indicates a weak relationship between forest cover and fungal NPP assemblage. The absence of a close relationship between forest cover and the overall NPP assemblage supports the findings of previous studies that NPPs provide a local signal (Wilmshurst and McGlone, Reference Wilmshurst and McGlone2005; Montoya et al., Reference Montoya, Rull and van Geel2010).
It is interesting to note that the samples most closely aligned with high forest cover are from the prehuman Vinillos sediments and are associated primarily with new morphotypes OU-5, OU-101, OU-106, OU-108, OU-115, OU-116, and OU-120 (lower central region in Fig. 4). However, the lack of a clear association with our environmental variables suggests that unparameterised factors are primarily driving fungal NPP assemblages in the prehuman Andean landscape. Based on the data available, it could be suggested that the inverse relationship of these morphotypes with the gradients for aquatic remains and organic carbon may indicate that this unidentified gradient corresponds to other edaphic factors. The absence of NPP identifications and environmental association along the forest cover gradient illustrates that much research is still required in this area but suggests that further ecological information will be gleaned about prehuman Andean landscapes as the NPP record becomes better understood.
CONCLUSIONS
Here we present 54 distinct fungal NPP types identified from Quaternary sedimentary archives from the eastern Andean flank of Ecuador. This work represents the first record of fungal NPPs from Ecuador and identifies 25 new fungal NPP morphotypes (Supplementary Appendix B). Along an environmental gradient of forest cover, fungal NPPs show a statistically significant change between low and medium levels of forest cover. The NPP assemblage found in low forest cover is characterised by the morphotypes Neurospora, HdV-201, IBB-16, OU-102, and OU-110. Increases in forest cover above this point produce no statistically significant change in NPP assemblage composition. However, preliminary groups of morphotypes more common in medium and high forest cover include Cercophora-type 1, Xylariaceae, Rosellinia-type, Kretzschmaria deusta, Glomus, Podospora, Sporormiella, HdV-16A, and OU-18, and Anthostomella fuegiana, OU-5, OU-101, OU-108, and OU-120, respectively.
The comparison of fungal NPP assemblage data with independent environmental proxy data derived from sedimentary archives within a CCA has demonstrated that this method can be used to improve our understanding of NPP autoecology. This improved understanding of NPPs will, we hope, improve palaeoenvironmental reconstructions from the Andes. However, future work undertaking modern NPP altitudinal transects in conjunction with mycological field studies to understand plant-fungal interactions in these biodiverse forests are required in order to capitalise on the use of NPPs as an effective palaeoecological proxy in the Neotropics. Among the environmental variables considered, fire regime (microcharcoal) and depositional environment (aquatic remains and organic carbon) were found to be the most important explanatory variables. Moreover, the importance of fire occurrence has been related to the only statistically significant zone (<8% forest cover) found, which is characterised by the fungal NPP assemblage Neurospora, HdV-201, IBB-16, OU-102, and OU-110, representing an open and degraded landscape within the montane forests of the eastern Andean flank that only occurs subsequent to human arrival on the continent. Further studies of modern and sedimentary samples along a variety of environmental gradients are required to improve the utility of fungal NPPs as a proxy within the Andes. To progress understanding of NPPs in the Andes, we recommend that all NPP remains should be recorded as part of any palynological investigation, even when the identities of the NPP morphotypes are unknown.

Figure 5 (colour online) New fungal morphotypes described from the eastern Andean flank of Ecuador. Conidia positioned with proximal cell at bottom. 1, OU-5; 2, OU-18; 3, OU-28 (a–c); 4, OU-35; 5, OU-100; 6, OU-101; 7, OU-102; 8, OU-103; 9, OU-104; 10, OU-105; 11, OU-106; 12, OU-107; 13, OU-108; 14, OU-109; 15, OU-110; 16, OU-111; 17, OU-112; 18, OU-113; 19, OU-114; 20, OU-115; 21, OU-116; 22, OU-117; 23, OU-118; 24, OU-119; 25, OU-120.
Acknowledgments
This work was supported by the Natural Environment Research Council (NERC) and the Open University through a scholarship to NJDL (NE/L501888/1), and an NERC fellowship to EM (NE/J018562/1). Radiocarbon dating was funded by the NERC radiocarbon committee (#1810.0414) and undertaken by Pauline Gulliver at the NERC Radiocarbon Facility East Kilbride (NRCF010001). The Huila (2013) fieldwork was undertaken as part of an NERC fellowship (NE/J018562/1). The Vinillos (2012) fieldwork was undertaken as part of an NERC-funded studentship (NE/J500288/1). We thank Annemarie Philip (University of Amsterdam, the Netherlands) for assistance with palynomorph preparation, Patricia Mothes (Instituto Geofísico, Escuela Politécnica Nacional, Ecuador) for guiding us to the Vinillos and Huila sites, and Hayley Keen, Frazer Matthews-Bird, and James Malley (all from the Open University, United Kingdom) for assisting with the collection of the Vinillos samples. The authors wish to especially thank Bas van Geel and an anonymous reviewer for their comments and constructive criticism of the manuscript.
Supplementary material
To view supplementary material for this article, please visit https://doi.org/10.1017/qua.2017.73