Introduction
Cryopalynology, or the analysis of palynomorphs found in snow, firn and ice, is emerging as an important and active subfield within traditional palynology. Since its inception in the mid20th century, cryopalynology has evolved spatially from its origins in the European Alps (e.g. Reference VareschiVareschi, 1935; Reference GodwinGodwin, 1949; Reference Ambach, Bortenschlager and EisnerAmbach and others, 1966), to the poles (e.g. Reference HeusserHeusser, 1954; Reference Fredskild and WagnerFredskild and Wagner, 1974; Reference Lichti-FederovichLichti-Federovich, 1974, Reference Lichti-Federovich1975a,Reference Liu, Yao and Thompsonb; Reference McAndrewsMcAndrews, 1984; Reference Bourgeois, Koerner and AltBourgeois and others, 1985, 2000, 2001; Reference Short and HoldsworthShort and Holdsworth, 1985; Reference BourgeoisBourgeois, 1986, Reference Bourgeois1990, Reference Bourgeois2000; Reference Koerner, Bourgeois and FisherKoerner and others, 1988; Reference Gajewski, Garneau and BourgeoisGajewski and others, 1995; Reference Andreev, Nikolaev, Bol’sheiyanov and PetrovAndreev and others, 1997), then the tropics (e.g. Reference Reese and LiuReese and Liu, 2002, 2005a,b; Reference Reese, Liu and MountainReese and others, 2003; Reference Liu, Reese and ThompsonLiu and others, 2005, Reference Liu, Reese and Thompson2007; Reference HerrerosHerreros and others, 2009) and finally to midlatitude snow and ice (e.g. Reference NakazawaNakazawa and others, 2004, Reference Nakazawa2005, Reference Nakazawa2011, Reference Nakazawa, Konya, Kadota and Ohata2012; Reference UetakeUetake and others, 2006, Reference Nakazawa and SuzukiNakazawa and Suzuki, 2008). Fossil pollen found in snow and ice is a direct biological link to past environments and thus has been used in a variety of research genres throughout the discipline’s maturation process, including history and paleoecology. Examples include the reconstruction of plant assemblages (e.g. Reference Lichti-FederovichLichti-Federovich, 1975a; Reference McAndrewsMcAndrews, 1984; Reference Short and HoldsworthShort and Holdsworth, 1985; Reference Bourgeois, Koerner, Gajewski and FisherBourgeois and others, 2000), vegetation responses to climate change through time (e.g. Reference Liu, Reese and ThompsonLiu and others, 2005; Reference Reese, Liu and ThompsonReese and others, 2013) and atmospheric circulation research (e.g. Reference Bourgeois, Koerner and AltBourgeois and others, 1985, 2001; Reference Short and HoldsworthShort and Holdsworth, 1985; Reference Reese and LiuReese and Liu, 2002, 2005a, Reference Liu, Reese and Thompsonb; Reference Reese, Liu and MountainReese and others, 2003; Reference Liu, Reese and ThompsonLiu and others, 2007; Reference HerrerosHerreros and others, 2009). However, little attention has been given to understanding the modern pollen processes of deposition, entrainment and transport and other postdepositional processes on snowfields and how they affect pollen stratigraphy within snow and ice records. Such a theoretical framework is essential to accurate environmental reconstructions with pollen archived in ice cores. Therefore, the main objective of our study is to address one aspect of these modern processes by investigating whether meltwater percolation can effectively transport pollen within a snowpack.
We know of only one study that has attempted to address this question. Reference Nakazawa and SuzukiNakazawa and Suzuki (2008) concluded that pollen is indeed concentrated at the surface of a melting snow cover. Their research was conducted in the field, on a snowpack located in the Norikura Highland, central Japan. A total of three snow pit samples (which extended temporally back to the start of that year’s accumulation season) were taken between March and April, 2005, with an additional five pits analyzed between March and May 2006. These pits were sampled stratigraphically and analyzed for pollen. Their results show that, as the snow melts, the pollen resists movement and rides the deflating snow surface downward, concentrating the pollen in the process, much like pollen in a sublimating, not melting, snowpack (Reference Reese and LiuReese and Liu, 2005a; Reference Liu, Reese and ThompsonLiu and others, 2007). However, this finding runs counter to several icecore pollen studies that show a large disparity in pollen concentrations between the surface snow and the samples found deeper within the firn and glacial ice (e.g. Reference Liu, Yao and ThompsonLiu and others, 1998; Reference Reese, Liu and MountainReese and others, 2013). In addition to this, our ongoing research on McCall Glacier, Brooks Range, northeast Alaska, has shown that samples collected in pollen traps above the glacier surface and in surface snow can have an order of magnitude more pollen than core samples recovered from the firn.
A potential problem with the study of Reference Nakazawa and SuzukiNakazawa and Suzuki (2008) is that they did not directly investigate horizontal relocation due to slope angle; no details on the environmental setting at the pit sites (e.g. slope) were given. Also, their study did not include meltwater runoff collection, so pollen removal via meltwater runoff (thus quantifying pollen loss) could not be tested.
Our experiment builds upon this initial study by investigating both vertical and horizontal pollen transport, while also capturing the meltwater for pollen analysis. Our study questions are as follows:
-
1. Can meltwater percolation effectively transport pollen from the surface downward into deeper layers of the snow?
-
2. Do surface slope and meltwater induce horizontal movement of pollen or spores?
-
3. Do ice lenses block pollen/spore transport or change its direction?
-
4. Do pollen/spores flow with subsurface meltwater or become entrained in/on the ice beneath the firn?
Materials and Methods
Sampling procedure
We chose an experimental laboratory design because it was not cost-or time-efficient to observe a natural melting snow cover over a period of weeks, or to conduct a controlled experiment on a melting glacier, as we could not adequately control the experimental variables (radiation, melt time, etc.). This study was conducted outdoors from 28 February to 2 March 2012 in Fairbanks, AK, USA, before the start of winter snowmelt. The aim of the experiment was to maximize control and isolate the variables being observed, in order to focus on the fundamental relationship between meltwater percolation and pollen transport. Fairbanks also provided easy access to tools, supplies, electricity and snow.
To simulate a natural snowpack, nine Styrofoam coolers of the same brand and model were placed outside in October 2011 and allowed to fill naturally with snow. Snow densities were qualitatively assessed through visual inspection during sampling. Similar to snow profiles on McCall Glacier, dense snow layers were located near the surface and hoar layers were found at the bottom of each cooler. The dimensions of the coolers were 60 cm × 30 cm × 30 cm (Figs 1–4). Three of the coolers were filled with 5 cm liquid water, which was allowed to freeze before accumulation began. These three coolers, with ice lenses at the bottom, were used in the ice-tilted (IT) experiment outlined below.

Fig. 1. Basic SF setup.

Fig. 2. Basic SF setup with samples removed.

Fig. 3. Basic ST setup.
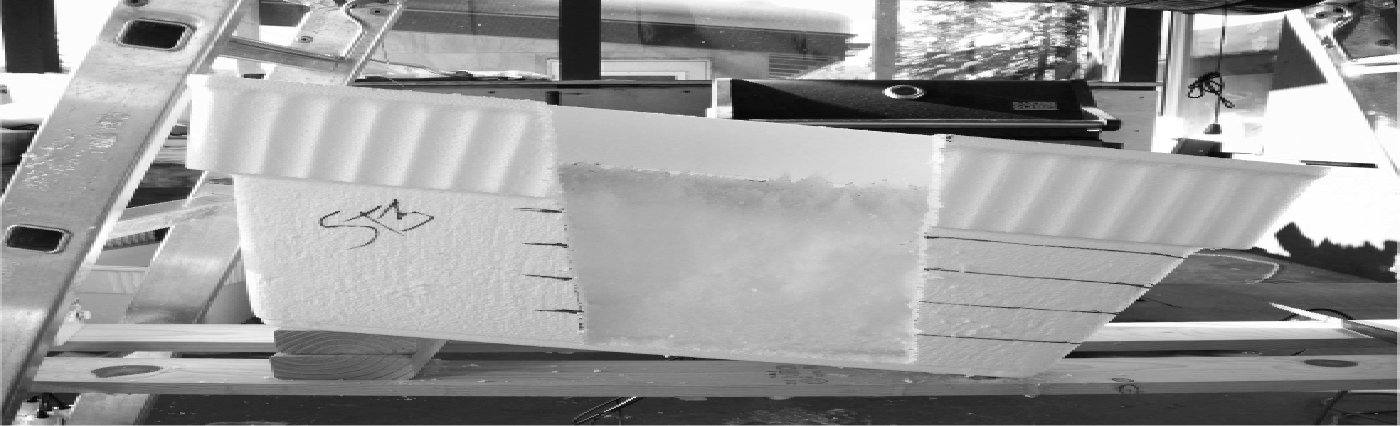
Fig. 4. Basic ST setup with sidewall removed.
Immediately prior to use, individual coolers were dug out of the snowpack with a shovel and scraped level along the plane of the cooler rim to ensure consistent starting volumes. Five Lycopodium tablets (batch 1031 from Lund University, Sweden) were pulverized using a porcelain mortar and pestle (20 848 spores tablet–1, 104 242 total spores cooler–1, std dev. = ±3457, variance = ±3.3%) (Reference MaherMaher, 1981). At the time of the experiment, Lund University was the only known source of standardized marker tablets, and thus our only choice for the experiment. Lund University uses a Coulter ZB particle counter to ensure consistency among tablets (variance is reported above). These Lycopodium spores vary between 25 and 40 μm, statistically similar to the average size of pollen from vascular plants.
The five pulverized capsules, now in powder form, were spread over a 20 × cm × 20 cm (400 cm2) study area on the surface of each cooler prior to melting. Physical pulverization of the tablets was chosen over dissolving the tablets in a liquid because a liquid solution would have been very difficult to distribute evenly over the entire study area. In addition, we did not want to introduce any liquid water to the snowpack, which may have artificially hastened downward transport of the spores.
The 20 × cm × 20 cm study area was located in the center of each cooler’s surface using a cardboard stencil to reduce edge effects. Using a 1500 W (5120 BTU) radiant heat lamp (anchored ~25 cm away from, and parallel to, the surface), the contents of each cooler were melted down to ~67% of the original volume and allowed to percolate for 30 min after heating. All meltwater was collected from each cooler through a single small aperture (~ 1.25 cm diameter) located at the lowest possible point. To gain access to the snow profiles after melting, a saw was used to remove the Styrofoam sidewall of each cooler. Ambient air temperature throughout the experiment was between –24°C and –12°C.
The snow within three of the coolers was melted with the bottom of the cooler perfectly level (along a 0° plane) and the heat lamp directly overhead (perpendicular) to the snow surface. These snow/flat (SF; Fig. 1) coolers were used to simulate snow resting on a horizontal surface, as might be found on a mountain summit, a plateau or in certain areas of a col. The contents of the remaining six coolers (three completely filled with snow and three with snow overlaying a 5 cm layer of ice) were melted inclined at 11–12° (Fig. 3). These six coolers (snow/tilted (ST) and ice/tilted (IT)) were used to simulate snow on an inclined glacier surface, subjected to significant downhill forces that may transport pollen (via meltwater) more efficiently.
For the SF coolers (Figs 1 and 2), the surface samples represent the top 1–2 cm, consisting mostly of the penitentelike prisms of snow that formed at the surface during each melt. The remaining snow profiles were then sampled in 5 cm increments within the cooler to test for vertical spore transport. To test whether the spores remained within the confines of the column of snow directly beneath the study area, the snow border (~5 cm) immediately surrounding this designated area was tested for spores as well and divided into two samples, ‘border’ and ‘16–20 cm border’. The border samples contain the snow surrounding the surface–15 cm column, and the 16–20 cm border samples contain only the snow surrounding the bottommost sample (16–20 cm). The process was repeated for the ST coolers (excluding the border samples) (Figs 3 and 4) with the addition of the downhill column of snow, which was also sampled in 5 cm increments to test for horizontal relocation of spores downslope of the study area.
The IT coolers were melted until at least 95% of the ice surface under the snow was revealed. All meltwater and spores that ran off the surface were collected during this process. The remaining ice block, from the study area to the drain hole, was also melted, collected and tested for spore concentrations to determine whether any spores remained on the ice surface or became entrained in the ice.
All samples were transferred to 1 L leakproof polypropylene Nalgene bottles using a small metal shovel and plastic funnel, tagged and shipped back to the Biogeography Laboratory, University of Southern Mississippi, for processing and counting.
Laboratory processing techniques
Chemical processing
Meltwater samples were processed using methods first outlined by Reference Liu, Yao and ThompsonLiu and others (1998). The samples were poured into 1 L glass beakers through a 2 mm mesh screen to filter residual Styrofoam and detritus collected during the experiment. The meltwater and spores were then concentrated to ~50 mL via evaporation, and transferred into 15 mL test tubes by centrifuging and decanting the supernatant 10–12 mL at a time. Each sample was then subjected to a series of acid washes and distilled water baths, centrifuged and decanted to remove extraneous organic and inorganic constituents. 10% hydrochloric acid (HCl) was used to remove carbonates, 100% glacial acetic acid (GA) was used to remove water and acetalize the contents, and finally the samples were subjected to acetolysis (9 parts acetic anhydride: 1 part sulfuric acid) in a 2 min boiling bath to deflocculate and dissolve cellulose. Pollen grains and spores (e.g. Lycopodium) are composed of a unique natural copolymer known as sporopollenin, which is highly resistant to decay and the chemicals used during laboratory processing (Reference Fægri and IversenFægri and Iversen, 1950). In effect, the strong acids remove all extraneous materials, leaving behind only spores (or pollen grains).
The residual was then stained with red safranin dye, cleaned with tertiary butyl alcohol (TBA), transferred into 1 dram (3.7 mL) vials, centrifuged and decanted. The remaining concentrate was suspended in 1 mL silicone oil for 24 hours with the caps off. Silicone oil is denser than TBA and settles to the bottom, allowing the TBA to evaporate out of the vial. Thus, the final product, assuming minimal loss occurred, was the total amount of spores from each sample depth.
Slide preparation
Once the TBA evaporation was complete, each vial was filled to 1 dram (3.7 mL) with silicone oil and mixed thoroughly vertically and upside down using a vortex mixer. 100 μL of thoroughly mixed, homogeneous sample was then extracted from each dram using a variable volume micropipette (Accumax 20–200 μL, Cat. No. S902432F), mounted on a 75 mm × 55 mm microscope slide (Corning, Lot 19312009) and covered with a 45 mm × 50 mm glass coverslip (Fisherbrand, Lot 0416129). Samples of 100 μL provided sufficient volume to fill the area of the oversized coverslip without excessive leakage. A reverse pipetting technique was implemented for sample extraction from the vials because of the high viscosity of silicone oil. In reverse pipetting, a volume larger than the target volume is initially extracted into the tip, but the precise targeted volume is discharged onto the slide when the plunger is depressed to the first stop (Thermo Scientific, 2007). All Lycopodium spores suspended in the standardized (3.7 mL) and homogeneous (thoroughly mixed) volume of silicone oil (100 μL) were counted using a Nikon Eclipse E400 light microscope at 100× magnification. Pollen that naturally accumulated in the coolers and showed up on the slides was disregarded in the analysis.
Concentration and percentage calculations
Lycopodium spores typically range from 25 to 40 μm and are well representative (in terms of size) of common palynological spectra found in glacial ice (aside from Abies spp., Picea spp. and Pinus spp. grains, which range from 60 to 130 μm). The spores are commonly used among the palynological community as markers for quantifying taxa and sample concentrations against a known number (Reference StockmarrStockmarr, 1971). Typically, samples are spiked with a tablet of a known quantity after sampling but prior to laboratory processing, and are counted against the identified pollen, spores and other microfossils. Using a simple mathematical formula ({[grains counted/markers counted] × markers added}/volume), the identified sample and taxa concentrations can be calculated. This way, losses encountered during the decanting and transfer processes are experienced by both the spike and pollen grains, effectively preserving the ratio between the two. For this experiment, a known amount of marker spores (104 242) was added at the beginning of the sampling procedure and concentrated under the assumption that minimal losses occurred during container transfers and laboratory processing, thus theoretically leaving the original total number of spores after melting at each depth (i.e. surface, 1–5 cm, 6–10 cm, 11– 15 cm, 16–20 cm, meltwater) in their respective vials. Accordingly, snow samples with more spores should equate to vials containing more spores. By standardizing the volume of each vial to 1 dram (3.7 mL), a 100 μL (1/37th dram) sample of homogeneous mixture should represent the original total number of spores (when multiplied by 37) in that layer and thus can be counted against the original meltwater volumes, similar to a serial dilution. Accordingly, spore concentrations (spores mL–1) were calculated by multiplying the number of spores counted in 100 μL by 37 (100 μL × 37 = 3.7 mL or 1 dram) and dividing the product by the original meltwater sample volume (mL).
Given that the original number of spores introduced to the surface of each cooler was known (104 242) and the overarching objective was to determine where those spores were relocating regardless of sample volume, spore percentages of the accounted total provide a useful look at the underlying phenomenon as well. The spore percentages discussed in the results were calculated using the total accounted (excluding losses) spores extrapolated from the measurements of each dram. Estimated losses for each cooler and total accounted spores are reported in the results as well.
Potential error
Viscous solutions, such as silicone oil, can be difficult to vortex mix and pipette, and this could have been a source of error. However, each sample was harvested using precisely the same procedure so any error should be consistent across samples. A sharpened wooden applicator was used to suspend the sediment-like ring (spores) at the bottom of each vial. Each sample was then mixed for 30 s upside down, vertically, and upside down again immediately prior to pipetting.
This novel technique for quantifying spore concentrations does not account for spores lost during the chemical processing. It is possible that more spores were lost in some samples than others within an individual cooler during the decanting processes; however, it is unlikely that these biases could have been repeated three times. The collective results from the three repetitions, when viewed simultaneously, conveyed very little variability.
There were several unresolvable issues with the sampling procedure that must be noted, namely randomness and sample size. Random sampling was unachievable within the confined working space of the coolers. However, from each vial, a random sample (100 μL) was mounted on a microscope slide to estimate spore concentrations, thereby inserting some randomness to the study. The sample sizes were also theoretically small, but pragmatically as large as possible without being cumbersome: the SF coolers, when repeated three times, yielded 24 samples, the ST coolers yielded 33 samples and the IT coolers yielded 6 samples, for a total of 63 samples (Table 1). Owing to contemporary laboratory processing and counting methods, the sample size had to be pragmatically small. It was not cost-or time-effective to analyze large sample pools using contemporary pollen analysis techniques. Accordingly, results from statistical analyses are not included, as only three coolers were analyzed from each group (SF, ST and IT), which would lead to marginal statistical results at best.
Table 1. List of samples from each stratum
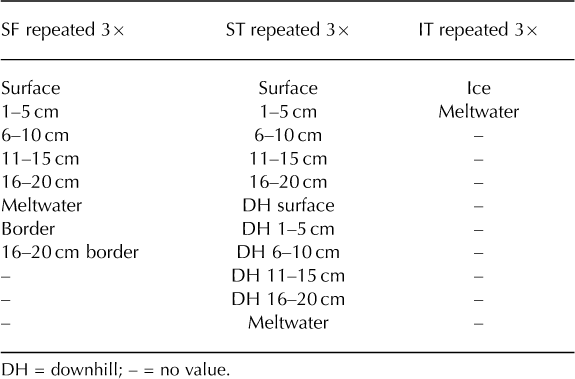
Results
Overall, the melting process went well. The melting front contained observable penitente-like prisms as are found on a naturally melting snow surface. Minor preferential melting occurred near the cooler edges due to the thermal differences between the snow and the Styrofoam. A few of the coolers exhibited minor (1–2 cm) slouching toward the center compared with the edges. The average melting rate was ~8 cm h–1.
Snow/flat stratum
Overall, spore concentrations within the SF stratum (Fig. 5) ranged from 1.2 to 16.8 spores mL–1, exhibiting some within-group variability. The highest spore concentration (16.8 spores mL–1) occurred in the 16–20 cm and meltwater samples from cooler SF3. The lowest concentration (1.2 spores mL–1) occurred in the 6–10 cm sample from SF3. In terms of ranges and averages, surface samples ranged from 5.3 to 16.3 spores mL–1 (11.2 average), midlevel samples (1–15 cm) contained 1.2–7.1 spores mL–1 (3.5 average), the bottommost samples (16–20 cm) contained 13–16.8 spores mL–1 (15 average) and the meltwater samples ranged from 5.3 to 16.8 spores mL–1 (9.9 average). Samples outside the 20 cm × 20 cm study area (border and 16–20 cm border) contained relatively low spore concentrations (1.4–4.5 spores mL–1; 2.9 average) comparable with the midlevel samples. The lower 16–20 cm border snow was approximately twice as concentrated as the upper border samples in each cooler.

Fig. 5. SF coolers 1–3. Results exhibit significant downward transfer due to meltwater percolation.
Peak spore concentrations within the SF stratum occurred at the surface only in cooler SF1. Coolers SF2 and SF3 both peaked in the two bottommost samples (SF2: 16–20 cm; SF3: 16–20 cm and meltwater).
Again, because a known number of spores were introduced to the surface of each cooler, and the objective was to document the horizontal and vertical movement, spore percentages (of the accounted spores) provide a meaningful look at where the majority of those spores relocated during melt, regardless of volume. Within the SF stratum, 50–62% of 104 242 spores introduced to each cooler’s surface were lost during processing.
In terms of the accounted spores (Fig. 5), 11–25% were found outside the 20 cm × 20 cm study area in the bordering snow (border and 16–20 cm border samples), meaning most of the spores (75–89%) stayed within the vertical confines of the surface application area. Of the spores that were transported horizontally, the highest percentages were found in the lower 16–20 cm border samples (7–16%).
Excluding the upper border sample, only 4–13% of spores were found in the SF surface layers, while the remaining 79–92% exhibited downward movement (i.e. 1–20 cm, meltwater and 16–20 cm border). Furthermore, 11–60% of the accounted spores were found in the meltwater samples alone and, when combined with the 16–20 cm layers, make up 47–80% of the total accounted spores. In other words, the majority of the spores left the surface and travelled into or through the subsurface.
Snow/tilted stratum
Overall, the ST coolers (Fig. 6) exhibited very little withingroup variability. Concentrations within the ST stratum ranged from 0 to 22.9 spores mL–1. Among all three coolers, the highest concentrations were found in the downhill 16–20 cm layers (16.2–22.9 spores mL–1) while the lowest concentrations were located in the upper reaches of the downhill column of snow (downhill surface 10 cm; 0–0.5 spores mL–1). The second highest concentration was found in the meltwater samples (12.4–17.6 spores mL–1) and third highest at the bottom of the study area snow column (16–20 cm; 6–8.5 spores mL–1). In other words, the three most concentrated samples were found in the bottom-downhill portions and meltwater of each cooler. Surface samples contained between 2.0 and 5.3 spores mL–1.

Fig. 6. ST coolers 1–3. Results exhibit significant downward and downhill transfer due to meltwater percolation.
Within the ST stratum of coolers, 29–61% of the 104 242 spores introduced to each cooler’s surface were lost during processing. As for the accounted spores (Fig. 6), the majority was found in the meltwater samples (72–80%). Surface layers (surface and downhill surface) contained only 0.2–1.7% of the accounted spores, meaning the remaining 98% moved downward in some fashion. Excluding the surface 20 cm profile, 83–91% of spores exhibited downhill or horizontal relocation during melt. Collectively, the samples that exhibited both downward (vertical) and downhill (horizontal) movement (downhill 1–20 cm and meltwater) represent 82–91% of all accounted spores.
Ice/tilted stratum
Overall, the IT coolers (Fig. 7) exhibited only slight within-group variability. Spore concentrations ranged from 2.1 to 15.1 spores mL–1. Among all three coolers, peak spore concentrations occurred in the meltwater samples (10.2–15.1 spores mL–1), leaving the ice blocks with the lowest concentrations (2.1–5.2 spores mL–1). The average spore concentration for the IT meltwater samples was 12.3 spores mL–1, while the IT ice samples averaged 3.3 spores mL–1. Thus the meltwater samples were almost four times as concentrated as the ice samples.

Fig. 7. IT coolers 1–3. Results exhibit significant spore loss within the meltwater runoff.
Within the IT set of replicates, 24–36% of the original 104 242 spores introduced to each surface were lost during processing. As for the accounted spores (Fig. 7), 67–94% were found in the meltwater samples while the ice samples contained 6–33%. IT3 varied most from the group (meltwater 67%; ice 33%) yet still corroborated the general results from IT1 and IT2.
Summary
Overall, peak spore concentrations occurred at the surface in only one of nine coolers (SF1). In the other eight coolers, peak concentrations were found in either the bottommost snow layers (16–20 cm) or meltwater runoff. As for the accounted spore percentages, the majority of Lycopodium spores introduced at the surface made their way downward (and downhill for ST coolers) into the 16–20 cm snow layers and meltwater samples during each melt. Tables 2–4 contain all the raw data used to create the spore diagrams.
Table 2. SF stratum: raw data for coolers 1–3

Table 3. ST stratum: raw data for coolers 1–3
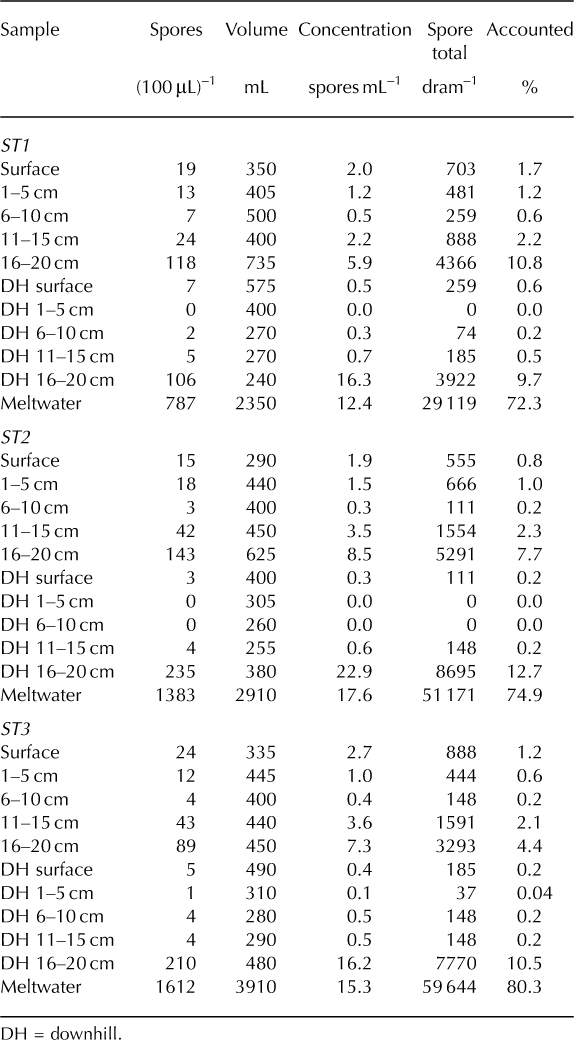
Table 4. IT stratum: raw data for coolers 1–3

Discussion
The cryopalynological community has been operating under the assumption that post-depositional meltwater incursion has very little impact on pollen stratigraphy (Reference Ambach, Bortenschlager and EisnerAmbach and others, 1966; Reference Nakazawa and SuzukiNakazawa and Suzuki, 2008; Reference Nakazawa, Konya, Kadota and OhataNakazawa and others, 2012). Based on the reviewed literature, this notion was mainly deduced through tangential observations, such as seasonal banding within the snowpack or the presence of summer ice layers rather than direct testing (e.g. Reference VareschiVareschi, 1935; Reference Ambach, Bortenschlager and EisnerAmbach and others, 1966; Reference Nakazawa, Konya, Kadota and OhataNakazawa and others, 2012). The prevailing viewpoint is that pollen grains are concentrated at the melting surface (Reference Nakazawa and SuzukiNakazawa and Suzuki, 2008; Reference Nakazawa, Konya, Kadota and OhataNakazawa and others, 2012). In other words, the melting surface picks up grains from below as it diminishes, thereby decreasing the relative proportion of pollen to water volume and thus increasing surface concentrations. Contrary to chemical and isotopic stratigraphies that are highly susceptible to degradation by meltwater (e.g. Reference Eichler, Schwikowski and Ga¨ggelerEichler and others, 2001; Reference Moran and MarshallMoran and Marshall, 2009), the unique stratigraphic preservation of pollen has been attributed to grain size by some authors (i.e. Reference NakazawaNakazawa and others, 2004, 2012), with little evidence to substantiate that claim.
Conversely, ongoing investigations at McCall Glacier reveal a discrepancy between pollen concentrations found in the air above the surface (i.e. pollen trap collections), in the surface snowpack and firn zone and in the glacial ice. In general, pollen concentrations are highest (up to 26 000 grains L–1) above the surface (airborne pollen in trap samples), second highest in the snow and firn layers (up to 15 000 grains L–1) and lowest in the ice core (up to 3500 grains L–1). Furthermore, pollen concentrations diminish downcore (depth decay), potentially due to increased exposure of older ice to meltwater events rather than to distance decay from vegetation during glacial periods as observed on ice caps in northern Canada, Greenland and Russia (e.g. Reference Fredskild and WagnerFredskild and Wagner, 1974; Reference McAndrewsMcAndrews, 1984; Reference BourgeoisBourgeois, 1986; Reference Gajewski, Garneau and BourgeoisGajewski and others, 1995; Reference Andreev, Nikolaev, Bol’sheiyanov and PetrovAndreev and others, 1997). On McCall Glacier, some of the deeper ice layers are at their pressuremelting point and may produce influxes of meltwater within the glacier that may transport pollen. Another logical culprit could be laminar flow as the glacial ice is compressed, giving the appearance of loss. Regardless, the pollen is being transported somewhere and the genesis of this project was to identify whether meltwater percolation is capable of such transport.
We believe this is the first experiment to directly investigate the effects of meltwater percolation on pollen records in both vertical and horizontal dimensions, while simultaneously capturing and quantifying spore concentrations in meltwater runoff. Given that Lycopodium spores were introduced only on the surface of each cooler inside a 20 cm × 20 cm surface area, the discovery of spores in lower layers must be attributed to vertical spore transport from meltwater percolation. Furthermore, any spores found downhill of the study area must be a product of horizontal transport due to slope angle. Overall, results from this melting experiment strongly suggest significant vertical and horizontal spore relocation due to meltwater percolation, with slight variability between the SF and IT coolers.
Snow/flat stratum
All three coolers within the SF stratum (Fig. 5) exhibited significant downward transfer of Lycopodium spores, with some variability among coolers. Although the peak spore concentration in SF1 occurred at the surface, the lower layers (1–20 cm) and meltwater contained 89% of accounted spores introduced at the surface, meaning the majority of spores moved downward. This anomalous surface peak is most likely due to the low volume of the sample and a result of sampling error. For example, the surface sample for SF2 contained more spores than that for SF1, but was suspended in over twice the volume and thus was less concentrated. This general concept is the reason both spore concentrations and accounted percentages are reported, a unique luxury only possible because the number of introduced spores was known.
A potential culprit for the variability in the meltwater and 16–20 cm samples could be the drain hole through which meltwater was supposed to flow. Visible freezing that occurred around the drain hole during each melt was knocked loose to ensure unimpeded drainage. Pooling in the 16–20 cm layers may have also occurred from such drainhole blockages, potentially diluting the meltwater samples and concentrating the 16–20 cm border snow samples. For example, of the three coolers, SF2 contained the lowest spore concentration in the meltwater, the second highest concentration of the 16–20 cm layer samples and the highest concentration of the 16–20 cm border samples. In other words, a number of spores might have begun to move laterally at the bottom of the cooler instead of through the drain hole and into the meltwater collection bottle. If the drain holes had been larger, it is possible that more spores would have drained out of the cooler with the meltwater. This might have happened in all three SF coolers, accounting for the majority of the spores that moved beyond the designated bounds of the study area. In nature, nightly refreezing episodes can produce similar impermeable ice lenses within a snowpack, which may promote the collection of pollen above the lenses. This phenomenon could explain some of the observed seasonal banding. However, during warm summer days, the lenses can also disappear, allowing the pollen to continue percolating downward via the meltwater.
Regardless of this possibility, a composite look at all three SF coolers strongly suggests vertical spore transport and not concentration at the surface as observed by Reference Nakazawa and SuzukiNakazawa and Suzuki (2008). This means that seasonal or intraannual resolution may be impossible to achieve on glaciers that experience significant melting. Furthermore, partially melted profiles may also contain stratigraphic breaks or contamination in the pollen signal from the layers above.
Snow/tilted stratum
All three coolers within the ST stratum (Fig. 6) were remarkably consistent, showing significant downward and downhill spore transport due to meltwater percolation. Although all the downhill 16–20 cm samples contained the concentration peaks, the meltwater samples contained the highest proportion of introduced spores. These findings imply that spore (pollen) loss due to meltwater runoff may be increased by slope.
In physical terms, the downward forces acting upon an individual spore or pollen grain within the snow and/or firn zones include gravity and water pressure. With slope, the uphill column of snow (under natural conditions) is thicker than the downhill column of snow, which creates a pressure gradient. Unless frictional and/or buoyancy/capillary forces within the microcracks are great enough to counterbalance the forces produced by gravity and meltwater, the net trend will be for that grain to move downward and downhill. Consequently, glacial surface topography is an important factor that must be considered in surface and upper profile (firn) studies.
It is possible that downhill concave undulations or surface pools collect uphill pollen as the surface snow melts and washes pollen onto lower sections of the glacier. Theoretically, these concave pools may contain higher pollen concentrations conducive to cryopalynological studies. However, these signals would be composed of transported/mixed pollen from different times, seasons and years, which might lead to misleading results. On the other hand, ridges or convex surface features may promote enhanced downhill transport and thus contain less pollen; however, it would be less likely to be mixed or distorted. Therefore, seasonal/annual resolution might be possible only in these areas.
Ice/tilted stratum
Overall, the IT coolers (Fig. 7) exhibited major spore loss due to meltwater runoff. The idea of pollen being flushed off the ice surface during melting was first proposed by Reference GodwinGodwin (1949), but has since remained untested. Reference Nakazawa and SuzukiNakazawa and Suzuki (2008) also noted a stark decrease in peak pollen concentrations immediately prior to total surface melting, but did not collect meltwater runoff to determine whether it contained pollen. These findings corroborate Reference GodwinGodwin’s (1949) reservations and help explain the dropoff in pollen concentrations documented by Reference Nakazawa and SuzukiNakazawa and Suzuki (2008).
Loss and variability
The high rate of spore loss during the experiment presented here was most likely due to a combination of several factors, such as residual spores sticking to the mortar and pestle, wind loss and loss during the snow transfer into Nalgene bottles, but the most likely source was loss during chemical processing (e.g. container transfers and decanting). Nevertheless, the minimal variability in the results between coolers within the same stratum is an indication of good sampling and processing techniques. As mentioned above, the novel approach to calculating spore concentrations and percentages at each individual depth was a potential source of error because loss during chemical processing (i.e. container transfers, decanting) was heretofore unaccounted. However, it is very unlikely that preferential biases due to losses at individual depths could be repeated three times, thus making the conclusions drawn from these results much more reliable.
Conclusions
The results of this experiment indicate that meltwater is capable of transporting spores and pollen downward from the surface. Lycopodium spores are similar in size (25–40 μm) to many pollen types found in glacial ice, and exhibited significant transport during this experiment. Contrary to the findings of Reference Nakazawa and SuzukiNakazawa and Suzuki (2008), pollen may not concentrate at the melting surface, but instead be transported downward/downhill and even lost completely within meltwater runoff.
Slope angle also appears to aid in the downhill or horizontal transfer of pollen and, upon severe melting, may completely wash away the pollen signal with the meltwater runoff. Reference GodwinGodwin’s (1949) original ideas regarding horizontal pollen relocation at the surface are corroborated by these findings and may also help to explain the sharp decreases in pollen concentrations observed by Reference Nakazawa and SuzukiNakazawa and Suzuki (2008). These phenomena collectively have the potential to contaminate seasonal pollen stratigraphies in snow and firn zones and, ultimately, in glacial ice.
Ice and snow records that have not been subjected to significant meltwater percolation may contain accurate seasonal pollen stratigraphy. However, our results indicate that the presumed seasonality observed in the surface snow, firn and ice of alpine valley glaciers (Reference NakazawaNakazawa and others, 2004, Reference Nakazawa2005, Reference Nakazawa2011, Reference Nakazawa, Konya, Kadota and Ohata2012; Reference UetakeUetake and others, 2006) may simply be a product of meltwater transfer and subsequent deposition rather than a representation of the local or regional phenology. Therefore, using these pollen bands for highresolution analyses is questionable without full consideration of the effects of postdepositional processes such as meltwater percolation.
Acknowledgements
This work was supported by the US National Science Foundation (NSF) under grant Nos ARC1023449 and ARC1023509. Any opinions, findings, conclusions or recommendations expressed in this paper are those of the authors and do not necessarily reflect the views of the NSF.