Article contents
Diversification and ecosystem services for conservation agriculture: Outcomes from pastures and integrated crop–livestock systems
Published online by Cambridge University Press: 11 March 2013
Abstract
Conservation agricultural systems rely on three principles to enhance ecosystem services: (1) minimizing soil disturbance, (2) maximizing soil surface cover and (3) stimulating biological activity. In this paper, we explore the concept of diversity and its role in maximizing ecosystem services from managed grasslands and integrated agricultural systems (i.e., integrated crop–livestock–forage systems) at the field and farm level. We also examine trade-offs that may be involved in realizing greater ecosystem services. Previous research on livestock production systems, particularly in pastureland, has shown improvements in herbage productivity and reduced weed invasion with increased forage diversity but little response in terms of animal production. Managing forage diversity in pastureland requires new tools to guide the selection and placement of plant mixtures across a farm according to site suitability and the goals of the producer. Integrated agricultural systems embrace the concept of dynamic cropping systems, which incorporates a long-term strategy of annual crop sequencing that optimizes crop and soil use options to attain production, economic and resource conservation goals by using sound ecological management principles. Integrating dynamic cropping systems with livestock production increases the complexity of management, but also creates synergies among system components that may improve resilience and sustainability while fulfilling multiple ecosystem functions. Diversified conservation agricultural systems can sustain crop and livestock production and provide additional ecosystem services such as soil C storage, efficient nutrient cycling and conservation of biodiversity.
Keywords
- Type
- Commentary
- Information
- Creative Commons
- This is a work of the U.S. Government and is not subject to copyright protection in the United States.
- Copyright
- Copyright © Cambridge University Press 2013
References
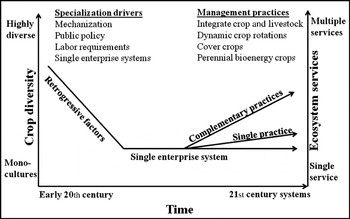
Figure 1. Schematic depicting crop diversity on US farms during the 20th century, the factors associated with the changes and proposed integrated management practices to increase crop diversity and deliver more ecosystem services.
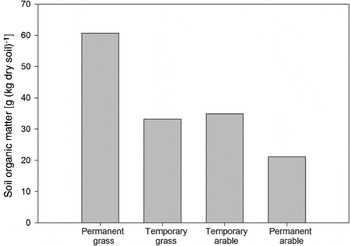
Figure 2. Soil organic matter levels (to a 10-cm depth) in four cropping systems implemented for 36 years in the Netherlands. Permanent grass was perennial ryegrass–white clover mixture. Temporary grassland alternated between 3 years of grass for grazing with 3 years of cropped forage for mechanical harvest. The temporary arable system alternated between 3 years of grass with 3 years of maize. The permanent arable system was in maize. Adapted from van Eekeren et al.31
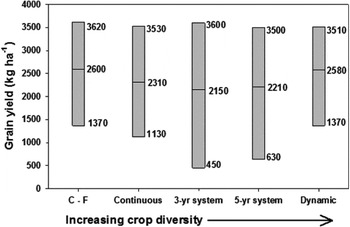
Figure 3. Minimum, maximum and mean spring wheat yield within five cropping systems differing in diversity near Mandan, North Dakota. C-F, Spring wheat–fallow; Continuous, continuous spring wheat; 3-yr system, three-year crop rotation; 5-yr system, five-year crop rotation; Dynamic, dynamic cropping system.
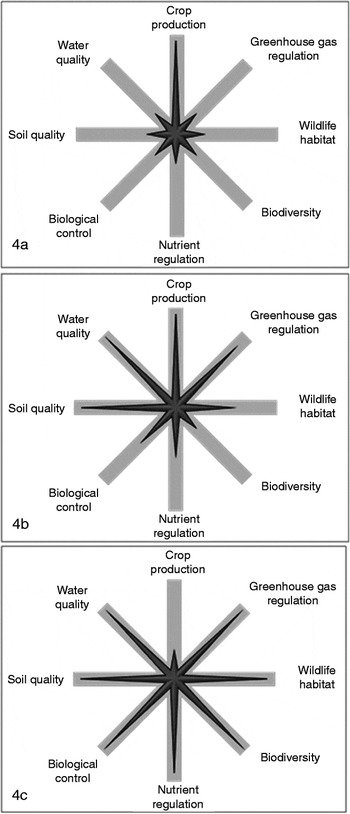
Figure 4. (a) Star diagram showing degree of ecosystem services associated with maize grain ethanol system. The length of each spoke shows the relative magnitude of each ecosystem service provided by the system relative to the highest provision of that service across all systems. (b) Star diagram showing degree of ecosystem services associated with switchgrass bioenergy system. (c) Star diagram showing degree of ecosystem services associated with low-input high-diversity bioenergy system. Source of information based on interpretation of information in selected references72–77.
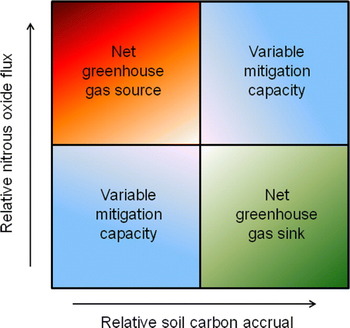
Figure 5. Conceptual diagram documenting potential climate mitigation outcomes associated with soil C accrual and nitrous oxide flux.
- 116
- Cited by
Overview
Greater demands for agriculture to provide food, feed, fiber and fuel have placed increased pressure on farmers to intensify their production practices. Increased use of external inputs and converting marginal land for cropping can compromise ecosystem services obtained from agriculture, especially natural resource conservation, soil health and biodiversity. An alternative would be to develop conservation agricultural systems that exploit synergies of crop diversity to improve ecosystem services. Ecosystem services include the ‘benefits humans derive, directly or indirectly, from ecosystem functions’Reference Costanza, d'Arge, de Groot, Farber, Grasso, Hannon, Limburg, Naeem, O'Neill, Paruelo, Raskin, Sutton and van den Belt1 and frequently are grouped into four broad categories of provisioning (e.g., food, feed and fiber), supporting (e.g., nutrient and water cycling), regulating (e.g., water purification) and cultural services (e.g., aesthetic experiences)2.
Agricultural systems became highly specialized during the 20th centuryReference Dimitri, Effland and Conklin3 with an accompanying decrease in diversity (Fig. 1). Government programs, reliance on petroleum-based products and improved technology are some of the reasons for increased specialization. This specialization decoupled crop and livestock production systemsReference Brummer4. One management alternative to the prevalence of specialized agriculture is integrated agricultural systems at a farm scale. Integrated systems conduct multiple enterprises on the same farm and products from one enterprise are often used by another enterpriseReference Hendrickson, Hanson, Tanaka and Sassenrath5, Reference Hillmire6. Integrated systems also include complementary practices, such as dynamic crop rotations, cover crops and direct utilization of feedstuffs by grazing animals, and can accommodate new enterprises such as perennial bioenergy crops (Fig. 1).
Figure 1. Schematic depicting crop diversity on US farms during the 20th century, the factors associated with the changes and proposed integrated management practices to increase crop diversity and deliver more ecosystem services.
There has been relatively little research on integrated agricultural systems, especially those that include livestock. Integrated crop–livestock research projects are large, not only in land area and livestock requirements but also in financial commitment by research agencies. These projects are often multi-disciplinary in nature and require compromise and cooperation among scientists to ensure that adequate data are collectedReference Tanaka, Karn and Scholljegerdes7.
As outlined by FranzluebbersReference Franzluebers8, conservation agricultural systems rely on three principles to enhance multiple ecosystem services: (1) minimizing soil disturbance, (2) maximizing soil surface cover and (3) stimulating biological activity. Managing for multiple ecosystem services in complex ecosystems (e.g., agroecosystems) requires a high degree of biodiversity in soil organisms, plant communities, and cropping and grazing systems distributed across the landscapeReference Duffy9, Reference Zavaleta, Pasari, Hulvey and Tilman10. We postulate that diverse agricultural systems, especially those that integrate crops and livestock, will conserve natural resources and enhance ecosystem services while maintaining productivity compared with traditional single-enterprise monoculture systems (Fig. 1).
In this paper, we explore the concept of diversity and its role in delivering ecosystem services from managed grasslands and integrated agricultural systems. We begin with managed grasslands and discuss how diversity affects productivity and resistance to weed invasion. Next, we discuss diversified cropping systems including perennial biofuels and then consider the integration of crops and livestock. We then discuss potential outcomes from diversification including the economics of integrated systems along with the trade-offs involved.
Managed Grasslands Diversity and Ecosystem Services
Managed grasslands provide significant provisioning services in the form of food, feed, fiber and fuel. Ecosystem services are enhanced when used as part of conservation approaches such as windbreaks for reducing erosion, vegetative barriers for trapping sediment and reducing water erosion, and buffer strips for protecting riparian zones among other standard practices11. Managed grasslands also serve as key reservoirs of biodiversity and can be used for bioenergy productionReference Sanderson and Adler12.
Plant diversity and grassland productivity
Meta-analyses have pointed to a positive relationship between plant species diversity (most often expressed as species richness) and phytomass productionReference Cardinale, Wright, Cadotte, Carroll, Hector, Srivastava, Loreau and Weis13 or have shown no clear relationshipReference Adler, Seabloom, Borer, Hillebrand, Hautier, Hector, Harpole, O'Halloran, Grace, Anderson, Bakker, Biederman, Brown, Buckley, Calabrese, Chu, Cleland, Collins, Cottingham, Crawley, Damschen, Davies, DeCrappeo, Fay, Firn, Frater, Gasarch, Gruner, Hagenah, Lambers, Humphries, Jin, Kay, Kirkman, Klein, Knops, La Pierre, Lambrinos, Li, MacDougall, McCulley, Melbourne, Mitchell, Moore, Morgan, Mortensen, Orrock, Prober, Pyke, Risch, Schutz, Smith, Stevens, Sullivan, Wang, Wragg, Wright and Yang14. A positive diversity–productivity relationship would have obvious practical application in managed grasslands. In that regard, agricultural scientists have begun to explore the relationship in an agronomic contextReference Wiegelt, Weisser, Buchmann and Scherer-Lorenzen15.
Greater herbage production from diverse mixtures of forage species compared with monocultures or bicultures (grass–legume) has been demonstrated in multiple experiments at different scales in the USReference Tracy and Sanderson16–Reference Picasso, Brummer, Liebman, Dixon and Wilsey19 and EuropeReference Bullock, Pywell and Walker20–Reference Nyfeler, Huguenin-Elie, Suter, Frossard and Lüscher22. Economic analyses have also pointed to greater economic returns and reduced production risk compared with grass monocultures or grass–legume biculturesReference Sanderson, Corson, Rotz and Soder23, Reference Deak, Hall, Sanderson, Corson and Rotz24. In several instances, the productivity benefit of complex mixtures stemmed from the inclusion of key species (e.g., highly productive or drought tolerant species), which supports the concept that functional traits rather than number of species govern outputs of ecosystem servicesReference Mouillot, Villéger, Scherer-Lorenzen and Mason25. The latter point emphasizes the need for more information on functional traits of forages to formulate rational combinations of plants to achieve specific functions in managed grasslandsReference Comas, Goslee, Skinner and Sanderson26.
In pasture-based livestock production systems, greater herbage production and nutritive value are not realized unless the grazing animal efficiently consumes and utilizes the herbage. Grazing trials with livestock have demonstrated variable results for beef cattle gain on forage mixtures versus monoculturesReference Tracy and Faulkner27 and no differences in milk production or herbage intake of dairy cowsReference Soder, Sanderson, Stack and Muller28. Very few grazing trials have been conducted to determine the livestock productivity response to plant diversity in pastures. Greater plant diversity may provide other benefits to grazing livestock including detoxification servicesReference Villalba, Provenza, Clemensen, Larsen and Juhnke29 and enhanced mineral nutritionReference Pirhofer-Walzl, Søegaard, Høgh-Jensen, Eriksen, Sanderson, Rasmussen and Rasmussen30.
Plant diversity and soil carbon
Managed grasslands generally have greater soil C levels than annual cropland (Fig. 2). For example, managed grasslands in Kansas had 43 Mg ha−1 greater soil C in the surface 1 m than annual croplandReference Glover, Culman, Tianna DuPont, Broussard, Young, Mangan, Mai, Crews, DeHaan, Buckley, Ferris, Turner, Reynolds and Wyse32. There are few, if any, studies on plant diversity effects on C sequestration in forage and pastureland. Research on restored native grasslands at the Cedar Creek, MN site indicated greater soil C storage with greater plant diversityReference DeDyn, Shiel, Ostle, McNamara, Oakley, Young, Freeman, Fenner, Quirk and Bardgett33. Grassland restoration practices increased soil C mainly because of the inclusion of the temperate legume red clover (Trifolium pratense L.) and not due to increased plant species richness generated by fertilizer reduction or seed additionReference DeDyn, Shiel, Ostle, McNamara, Oakley, Young, Freeman, Fenner, Quirk and Bardgett33. Inclusion of red clover may have enhanced nutrient cycling through food webs and improved soil structure leading to increased soil CReference van Eekeren, van Liere, de Vries, Rutgers, de Goode and Brussard34. Similarly, adding alfalfa (Medicago sativa L.) to annual cropping sequences for small grains increased soil C through greater belowground C input and soil biological activityReference Sainju and Lenssen35. Thus, the inclusion of species with key functional traits (e.g., N2 fixation through a legume) in managed grasslands may be more important than greater richness or diversity. Carbon sequestration in grasslands depends on maintaining C accumulation rates, avoidance of C loss via disturbance and restoring functionality of degraded grasslandReference Havstad, Peters, Skaggs, Brown, Bestelmeyer, Fredrickson, Herrick and Wright36.
Figure 2. Soil organic matter levels (to a 10-cm depth) in four cropping systems implemented for 36 years in the Netherlands. Permanent grass was perennial ryegrass–white clover mixture. Temporary grassland alternated between 3 years of grass for grazing with 3 years of cropped forage for mechanical harvest. The temporary arable system alternated between 3 years of grass with 3 years of maize. The permanent arable system was in maize. Adapted from van Eekeren et al.Reference van Eekeren, Bommele, Bloem, Schouten, Rutgers, de Goode, Rehaul and Brussard31
Invasion resistance
Invasion resistance of managed grasslands could be improved by formulating unique functional combinations of forage speciesReference Drewnovsky and James37. Research conducted at several scales in a variety of managed grasslands has indicated that species-rich forage mixtures can reduce weed invasionReference Sanderson, Soder, Muller, Klement, Skinner and Goslee17–Reference Picasso, Brummer, Liebman, Dixon and Wilsey19, Reference Kirwan, Luscher, Sebastia, Finn, Collins, Porqueddu, Helgadottir, Baadshaug, Brophy, Coran, Dalmannsdottir, Delgado, Elgersma, Fothergill, Frankow-Lindberg, Golinski, Grieu, Gustavsson, Hoglind, Huguenin-Elie, Iliadis, Jorgensen, Kadziuliene, Karyotis, Lunnan, Malengier, Maltoni, Meyer, Nyfeler, Nykenen-Kurki, Parente, Smit, Thumm and Connolly21, Reference Dodd, Barker and Wedderburn38–Reference Frankow-Linberg, Brophy, Collins and Connolly40. Combining forage species into functional mixtures has been successful in reducing the establishment of invasive plants in rangelandsReference Sheley and Carpinelli41. Mixed-species pastures may be more resistant to invasion by weeds because of (i) more competition created by greater use of resources or (ii) the inclusion of a few dominant productive species that preclude invasionReference Kennedy, Naeem, Howe, Knops, Tilman and Reich42.
Weed abundance was inversely related to the evenness (evenness is a measure of the relative distribution or proportion of plant species in a community) of forage species in clipped plots and on-farm surveys of plant species diversity in pasturesReference Tracy and Sanderson16, Reference Tracy and Sanderson43. In research designed to control for species evenness, however, there was no evidence that evenness of grass–legume mixtures affected weed invasionReference Sanderson, Brink and Stout44. Species composition of the mixtures, however, had a strong effect on invasion, indicating that selecting the appropriate species in mixtures to achieve a specific function is more important than evenness of the species in the mixture.
Management tools needed for diverse mixtures
Few, if any studies, have addressed management issues associated with the use of complex mixtures of forages or other crop plants. Tools are needed to aid the selection of specific mixtures for managed grasslands and to guide the spatial distribution or arrangement of species and mixtures across the various site-types on a farm to achieve specific functions. This includes defining the functional traits of plants so that a rational functional diversity approach can be followed, designing agricultural plant communities to achieve specific functions or combinations of functions rather than simply ‘piling on’ species to achieve diversity in numbers onlyReference Cadotte, Carscadden and Mirotchnick45. Management of highly diverse grasslands to deliver multiple ecosystem services (e.g., soil C storage, biodiversity conservation and enhanced nutrient cycling) in addition to herbage production will likely require trade-off among services and well-designed economic incentives for farmer adoptionReference Klimek, Richter gen., Steinmann, Freese and Isselstein46.
Increasing Cropping System Complexity to Improve the Productivity of Integrated Systems
The foundation of cropping system complexity has its basis in how crops are sequenced over time on the same area of land and where crops are planted across variable landscapes within an individual cropping year. Benefits to agronomic productivity and ecosystem services from increased cropping system complexity are derived from inherent physiological and morphological attributes of crops affecting soil nutrient dynamics, water availability, insect pressure, and incidence of weeds and diseasesReference Karlen, Varvel, Bullock and Cruse47. By rotating different crop types over time (e.g., grasses, legumes, oilseeds, brassicas, etc.) producers can exploit synergistic relationships among crops to reduce input requirements (e.g., following an N-fixing crop with a high N use crop). Moreover, placement of crops on landscape positions that best suits their growth can improve production efficienciesReference Karlen, Varvel, Bullock and Cruse47, decrease sediment lossReference Dabney, Wilson, Mcgregor and Vieira48 and support biodiversity conservationReference Concepción, Díaz and Baquero49. Greater attention to synergistic responses within crop rotations, along with increased awareness of crop–landscape complementarity, is crucial to optimize multiple ecosystem functions within integrated systemsReference Tanaka, Liebig, Krupinsky and Merrill50.
Crop selection and sequencing
Crop selection and sequencing can serve as a critical component in conservation of agricultural systems. Among the many options available to select and sequence crops, a fixed-sequence system, whereby crops are sequenced in a consistent, unchanging pattern, is the most simple. Fixed-sequence systems, however, can contribute to the development of pest and disease infestationsReference Krupinsky, Bailey, McMullen, Gossen and Turkington51, Reference Anderson52, be less responsive to external stressors (e.g., weather)Reference Farahani, Peterson and Westfall53 and may limit opportunities to take advantage of market conditions and/or government programsReference Karlen, Varvel, Bullock and Cruse47. Accordingly, fixed sequences can suffer from significant drawbacks that limit their sustainability over the long term, particularly in the context of challenges associated with anticipated climate change54.
To increase cropping system responsiveness to externalities, opportunity/flex crop sequences have been developed to allow for adjustments in cropping intensity and/or diversity using critical information at planting, such as soil water statusReference Farahani, Peterson and Westfall53, Reference Peterson, Westfall, Peairs, Sherrod, Poss, Gangloff, Larson, Thompson, Ahuja, Koch and Walker55. Even greater flexibility in annual crop sequencing may be realized through the application of a dynamic cropping system concept, where crop sequence decisions are made annually to optimize production, economic and resource conservation goalsReference Tanaka, Krupinsky, Liebig, Merrill, Ries, Hendrickson, Johnson and Hanson56. Dynamic cropping systems are location specific, with unique crop portfolios (i.e., adapted crop species) within an ecoregion, and require a thorough understanding of short-term (one to three years) crop sequencing effects on relevant agronomic and environmental response variablesReference Krupinsky, Tanaka, Merrill, Liebig and Hanson57. Successful application of the dynamic cropping system concept can increase adaptability to high-risk conditions, and may therefore be more economically and environmentally sustainable than other crop selection and sequencing approaches.
The application of dynamic cropping systems has increased crop yield and decreased yield variability in the northern Great PlainsReference Tanaka, Krupinsky, Merrill, Liebig and Hanson58. Strategic inclusion of dry pea (Pisum sativum L.) in a dynamic cropping system increased subsequent spring wheat (Triticum aestivum L.) grain yield by 30% compared with monoculture spring wheat because of improvements in precipitation-use efficiencyReference Tanaka, Liebig, Krupinsky and Merrill50. Analysis of long-term data revealed that spring wheat grain yield varied least in a dynamic cropping system compared with other less diverse crop-sequencing approachesReference Tanaka, Liebig, Krupinsky and Merrill50 (Fig. 3). Moreover, grain yield in the dynamic system was 17–20% greater than 3- and 5-year rotations containing spring wheat (Fig. 3). Such outcomes suggest an inherent resilience of dynamic cropping systems to achieve greater production consistently within a variable environment.
Figure 3. Minimum, maximum and mean spring wheat yield within five cropping systems differing in diversity near Mandan, North Dakota. C-F, Spring wheat–fallow; Continuous, continuous spring wheat; 3-yr system, three-year crop rotation; 5-yr system, five-year crop rotation; Dynamic, dynamic cropping system.
Cover crops
Use of cover crops in annual cropping systems is consistent with the principles of conservation agriculture from the standpoint of maximizing soil cover, stimulating biological activity and increasing diversity. Although cover crops are often categorized by type (e.g., grasses, legumes and brassicasReference Clark59), their use in addressing specific agronomic and/or natural resource issues underscores the value of functional categorization, such as increasing surface cover, assimilating plant nutrients, improving soil–water relations, and decreasing pests and diseasesReference Thorup-Kristensen60, Reference Snapp, Swinton, Labarta, Mutch, Black, Leep, Nyiraneza and O'Neil61. In this regard, numerous reviews have documented the functional value of cover crops to improve ecosystem services, environmental quality and farm profitability across a wide range of growing conditionsReference Clark59, Reference Russelle, Hargrove and Follett62–Reference Dabney, Delgado, Meisinger, Schomberg, Liebig, Kasper, Mitchell, Reeves, Delgado and Follett65. Among these outcomes, cover crops can reduce soil erosion by protecting soil from raindrop impact, improving soil structure and increasing infiltrationReference Langdale, Blevins, Karlen, McCool, Nearing, Skidmore, Thomas, Tyler, Williams and Hargrove66, Reference Kaspar, Radke and Laflen67. Cover crops can enhance soil biological abundance and activityReference Six, Frey, Thiet and Batten68, thereby contributing to increased N mineralization rates and N supplying potential of soilReference Grant, Peterson and Campbell69, Reference Wienhold and Halvorson70.
Emerging use of cover crop cocktails (i.e., complex mixtures of functionally diverse cover crops planted concurrently) offers the potential to exploit previously untapped spatial and temporal niches in diverse cropping systems to improve ecosystem services. Unfortunately, generalizations regarding their effectiveness are currently limited by a lack of published findings.
Bioenergy cropping systems
Before the 20th century, significant pasture and hayland areas were required for grazing animals to maintain draft power for rural farms and urban transportationReference McShane, Tarr and Mohl71. The transition from draft power to the internal combustion engine in US agriculture contributed to the decline in land dedicated to perennial systems, increased reliance on fossil fuels to produce food and led to single enterprise systems (Fig. 1). Concerns about energy security and increased greenhouse gas emissions from fossil fuels have boosted efforts to develop sustainable energy and transportation fuels from biomass. A greater reliance on biofuels may improve or worsen the long-term sustainability of arable cropland, biodiversity and human health, depending on the feedstock source and management practices implemented.
Maize (Zea mays L.) grain ethanol is currently the dominant biofuel feedstock in the US and had contributed to annual crop expansion into existing grasslands to meet the demands for food, feed and biofuelsReference Fargione, Cooper, Flaspohler, Hill, Lehman, Tilman, McCoy, McLeod, Nelson and Oberhauser72. Large-scale conversion of grasslands to maize production can have potentially large effects on biodiversity, water quality, wildlife habitat and other ecosystem services (Fig. 4). Agricultural residues, such as maize stover, are expected to provide substantial amounts of biomass for conversion into biofuels, but excessive maize stover removal can lead to increased soil erosion and decreased soil organic CReference Blanco-Canqui and Lal73. Further, grazing of maize stover after harvest is a simple and economical way of integrating crop–livestock systemsReference Klopfenstein, Roth, Rivera and Lewis78.
Figure 4. (a) Star diagram showing degree of ecosystem services associated with maize grain ethanol system. The length of each spoke shows the relative magnitude of each ecosystem service provided by the system relative to the highest provision of that service across all systems. (b) Star diagram showing degree of ecosystem services associated with switchgrass bioenergy system. (c) Star diagram showing degree of ecosystem services associated with low-input high-diversity bioenergy system. Source of information based on interpretation of information in selected referencesReference Fargione, Cooper, Flaspohler, Hill, Lehman, Tilman, McCoy, McLeod, Nelson and Oberhauser72–Reference Anand, Archer, Bergtold, Canales, Braun, Karlen and Johnson77.
If crop residues become a significant source of biomass feedstock, there will be a critical need for timely and accurate data on residue cover to ensure sustainability; however, no government program exists to objectively monitor residue cover across large areasReference Nowak79–Reference Aguilar, Evans and Daughtry81. The Conservation Technology Information Center (CTIC) holds the most extensive and up-to-date information on residue cover of the US Midwest, but these data are based on ‘windshield observation’ estimates82. Coupled with a strong scientific foundation and proven algorithms, remotely sensed data offer objective and fast measurement of crop residue cover over large areas. Moreover, accessibility to some satellite images is becoming easier as key government agencies offer these data to the public at no cost83.
Herbaceous perennial bioenergy crop systems are perceived to be more sustainable and provide more ecosystem services than maize grain ethanol or agricultural residuesReference Jordan, Boody, Broussard, Glover, Keeney, McCown, McIsaac, Mueller, Murray, Neal, Pansing, Turner, Warner and Wyse74 (Fig. 4). Environmental, societal and economic trade-offs need to be considered when transitioning back to the use of perennial feedstocks to meet rural and urban energy demands. Perennial energy crop systems will probably be planted on marginal cropland or conservation grasslandsReference Walsh, De la Torre Ugarte, Shapouri and Slinsky84. Converting annual cropland production to perennial systems will probably reduce soil erosion, increase soil C sequestration, reduce farm-level fossil fuel requirements and increase wildlife habitatReference Farrell, Plevin, Turner, Jones, O'Hare and Kammen75, Reference McLaughlin, De la Torre Ugarte, Garten, Lynd, Sanderson, Tolbert and Wolf76. However, converting idle, pasture, or conservation land to perennial energy crops may lead to wildlife habitat loss, a decline in plant community diversity and a shift in livestock production from pastures to confinement feeding depending on the region, feedstock used and management practices implemented. Perennial bioenergy cropping systems could also be used as a dual-purpose crop, in which a grazing or forage harvest is implemented in addition to a bioenergy harvestReference Guretzky, Biermacher, Cook, Kering and Mosali85. Rotational systems may be developed in specific climates to incorporate grazing livestock and bioenergy harvests. Integrating a livestock component within herbaceous bioenergy cropping systems further adds management complexity but may offer reduced enterprise risk.
Use of low-input high diversity prairie mixtures (up to 16 species) has been proposed as a sustainable way to produce bioenergy on degraded landsReference Tilman, Hill and Lehman86 (Fig. 4). Some research demonstrated greater biomass yields with greater numbers of perennial species in mixturesReference DeHaan, Weisberg, Tilman and Fornara87. In other research, however, two-species grass–legume mixtures or N-fertilized switchgrass monocultures produced similar or greater biomass yields than low-input high-diversity mixturesReference Schmer, Vogel, Mitchell and Perrin88, Reference Mangan, Sheaffer, Wyse, Ehlke and Reich89. Monocultures of warm-season grasses produced more biomass less expensively than polyculturesReference Griffith, Epplin, Fuhlendorf and Gillen90. Observational research on conservation grasslands in the northeastern USA indicated a negative relationship between plant species diversity on biomass production and biochemical composition conversionReference Adler, Sanderson, Weimer and Vogel91. An environmental trade-off exists between maximizing diversity within plant communities grown for bioenergy purposes and having meaningful biomass supplies to meet societal energy demands. However, it should be recognized that native, perennial monocultures or simple polyculture systems provide more ecosystem services such as C sequestration, wildlife habitat, landscape heterogeneity and nutrient regulation than the existing annual cropping systemsReference Camill, McKone, Sturges, Severud, Ellis, Limmer, Martin, Navratil, Purdie, Sandel, Talukder and Trout92.
Integrated Crop–Livestock Systems
Thus far, we have treated grassland livestock systems and cropping systems separately. World-wide, however, integrated crop–livestock systems (i.e., mixed crop–livestock systems) produce about half of the world's foodReference Herrero, Thornton, Notenbaert, Wood, Msangi, Freeman, Bossio, Dixon, Peters, van de Steeg, Lynam, Parthasarathy Rao, Macmillan, Gerard, McDermott, Seré and Rosegrant93. There are many ways that livestock and crop production can be integrated. This discussion will focus on integration in the USA where both cattle and annual crops are produced on the same area of land in the same year with cattle grazing crop residue or crops specifically grown for their consumption. This type of crop–livestock integration has both potential benefits and drawbacks. Benefits include adding value to crop residue with little additional input costs, reducing the cost of feeding livestock, reducing water requirements for crop production and reducing the environmental impact of livestock production.
An integrated crop–livestock system significantly lowered the average daily cost of wintering cows on the northern Great Plains compared with feeding them hayReference Karn, Tanaka, Liebig, Ries, Kronberg and Hanson94. Grazing cattle on cover crops during the winter on cropland used to grow cotton (Gossypium hirsutum L.) or peanuts (Arachis hypogea L.) during the summer increased farm income in the southeastern USAReference Franzluebbers95. Nearly 25% less irrigation water was used in an integrated cotton–forage–beef production system on the Texas High Plains compared with a cotton monoculture. The integrated system also had less soil erosion, required fewer chemical and N fertilizer inputs, and had greater rainfall infiltration than the non-integrated systemReference Allen, Baker, Segarra and Brown96. Pollution of ground water from N leaching and surface water enrichment with phosphorus is a key environmental concern associated with confining large numbers of cattle in feedlots with high animal densityReference Galyean, Ponce and Schutz97. As a consequence of feedlot-based cattle production, large amounts of urine and feces are concentrated on relatively small areas and atmospheric pollutants (ammonia, nitrous oxide, methane, particulates and odoriferous compounds) are emitted from the animals and (or) the feedlot surface. In contrast, integrating cattle with crop production tends to reduce the density of animals on the land and increases the potential for nutrients in feces and urine to be used for beneficial soil and plant developmentReference Tanaka, Karn, Liebig, Kronberg and Hanson98–Reference Liebig, Tanaka, Kronberg, Scholljegerdes and Karn100.
Soil-related outcomes associated with the integration of livestock and annual cropping systems have been variable, though limited work on the topic has been published. Soil organic C and total N have increased or stabilized with the inclusion of cattle within an annually cropped system or systems with a perennial grass phaseReference Drinkwater, Wagoner and Sarrantonio101–Reference Liebig, Tanaka, Kronberg, Scholljegerdes and Karn104. Such increases in soil C and N have been associated with greater aggregate stability, labile organic matter pools and infiltration rates, thereby conferring potential benefits to soil function associated with erosion resistance, nutrient cycling and soil water relationsReference Tracy and Zhang103–Reference Sulc and Tracy106.
Potential drawbacks of integrating crop and cattle production include soil compaction, which reduces crop production, and interference with new crop growth. Interference can occur if crops or crop residues are windrowed to facilitate grazing but are not adequately grazed during fall or winter because they are covered by excessive snow or ice or if they are not as palatable to cattle as expected. Cattle can cause soil compaction if they are allowed to graze corn residue when the soil is wet, so management guidelines encourage residue grazing only when the soil is dry or frozen unless the soil is tilled before seedingReference Sulc and Tracy106. On the cold northern Great Plains, water infiltration rates were not reduced with 3, 6 or 9 years of an integrated cattle–crop production system with late fall and winter grazingReference Liebig, Tanaka, Kronberg, Scholljegerdes and Karn100. This region of the US has distinctive freeze/thaw and wet/dry cycles that may limit soil compaction when cattle graze crop fields during the late fall and winter with occasional warm and thawing weather. Another potential drawback of integrated crop–livestock systems may be poor distribution of nutrients from manure and urine, leading to uneven plant growth.
Outcomes from Diversification of Cropping and Integrated Agricultural Systems
Fixing nitrogen to reduce external inputs
Legumes contribute N to cropping systems through symbiotic N fixation. Accordingly, legumes play an important role in diverse cropping systems through their effects on plant available NReference Grant, Peterson and Campbell69. Agronomic outcomes from the inclusion of legumes in cropping systems, however, depend on their use either as cover or grain crops, as most N in annual legumes is contained in seed. When harvested for seed, N contributions to the soil from annual legumes are negligible or slightly positiveReference Stevenson and van Kessel107, Reference Miller, Gan, McConkey and McDonald108. Conversely, when annual legumes are used as green manure, significant N can be made available to soil for subsequent crop uptakeReference Grant, Peterson and Campbell69, Reference Power109.
Legumes can have additional positive crop sequence effects on subsequent crops. Underlying mechanisms contributing to yield responses are highly complex, and are probably the interaction of legume effects on soil water status, N availability and disruption of pest cyclesReference Anderson52, Reference Miller, McConkey, Clayton, Brandt, Staricka, Johnston, Lafond, Schatz, Baltensperger and Neill110, Reference Merrill, Tanaka, Krupinsky and Ries111. Accordingly, association of N contributions from annual legumes with increased crop yield is not always consistentReference Krupinsky, Bailey, McMullen, Gossen and Turkington51, Reference Miller, McConkey, Clayton, Brandt, Staricka, Johnston, Lafond, Schatz, Baltensperger and Neill110. Conversely, perennial legumes, such as alfalfa, can provide significant short-term (3–5 years) N additions to soilReference Kelner, Vessey and Entz112 and subsequent yield increases in grain crops following forage stand terminationReference Hoyt113, Reference Entz, Baron, Carr, Meyer, Smith and McCaughey114. Such yield benefits from perennial legumes, however, are not reproducible across all management systems. In regions where limited precipitation seriously limits crop productivity, perennial legumes can create a ‘forage-induced drought’, resulting in depressed crop yields following stand terminationReference Entz, Baron, Carr, Meyer, Smith and McCaughey114.
Carbon sequestration
Adoption of complex cropping systems can enhance soil C sequestration and improve soil quality. The magnitude and rate of soil C sequestration depends on crop selection and sequence, site history, and edaphic and climatic factors that directly affect biomass productivity and C retention in soilReference Karlen, Varvel, Bullock and Cruse47, Reference Brady and Weil115. Crop sequences that include perennial phases are most effective at increasing soil C due to below-ground C input from root biomass and rhizodeposition and decreases in soil C loss from erosionReference Zan, Fyles, Girouard and Samson116, Reference Dabney, Shields, Temple and Langendoen117. Crop sequences with frequent inclusion of easily decomposable, low residue producing crops (e.g., annual legumes) are least likely to increase soil CReference Varvel118.
It is important to note that while certain cropping systems can lead to soil C accrual, their effects on global warming potential can be variable based on N2O flux, resulting in either enhanced (e.g., increased soil C and decreased N2O emission) or negated (e.g., increased soil C and increased N2O emission) climate mitigation potential (Fig. 5). Such variable responses emphasize the importance of inclusive greenhouse gas evaluations to clearly determine trade-offs associated with management.
Figure 5. Conceptual diagram documenting potential climate mitigation outcomes associated with soil C accrual and nitrous oxide flux.
Increased soil C in diverse cropping systems has been linked to changes in soil physical, chemical and biological properties that affect ecosystem functions, such as nutrient cycling, filtering and buffering capacity, and regulation of hydrological attributesReference Andrews, Karlen and Cambardella119, Reference Hudson120. Carbon-induced improvements in soil attributes and related functions can positively affect crop yield and environmental qualityReference Wienhold, Pikul, Liebig, Mikha, Varvel, Doran and Andrews121. Accordingly, management decisions leading to increased soil C in diverse cropping systems contribute to increased agroecosystem resilience under anticipated climate changeReference Delgado, Groffman, Nearing, Goddard, Reicosky, Lal, Kitchen, Rice, Towery and Salon122, Reference Lal, Delgado, Groffman, Millar, Dell and Rotz123.
Enhancing soil biology to improve soil function
Arbuscular mycorrhizal (AM) fungi are critical to the formation of economically and environmentally sustainable grasslands and livestock agricultural systems by closing water and nutrient cyclesReference Allen, Swenson, Querejeta, Egerton-Warburton and Treseder124, Reference Smith and Read125. These fungi are obligate root symbiotes of about 90% of all vascular plantsReference Carlile and Watkinson126 and are ubiquitous in most soilsReference Carlile and Watkinson126–Reference Wright and Upadhyaya128. In this relationship, plants allocate photosynthetic C to the fungus to stimulate the acquisition of nutrients from the soil via the fungal hyphaeReference Allen, Swenson, Querejeta, Egerton-Warburton and Treseder124, Reference Wright and Upadhyaya128. Plants also benefit through greater absorption of water; stimulation of growth hormones; protection against pathogens; and soil structural improvement for better porosity, water infiltration and root penetrationReference Allen, Swenson, Querejeta, Egerton-Warburton and Treseder124, Reference Miller and Jastrow129–Reference Purin and Rillig132.
AM fungal hyphae are also responsible for macroaggregate (>0.25 mm) formation by providing the framework upon which organic matter collectsReference Miller and Jastrow129. AM fungi produce glomalin, a glycoprotein found in abundance in both native and agricultural soilsReference Wright and Upadhyaya128, Reference Wright and Upadhyaya130. Glomalin is located in the fungal hyphal cell wall and may protect it from pathogen attack and nutrient and water lossReference Nichols, Wright, Magdoff and Weil131–Reference Borowicz133. As the hyphae decompose, glomalin sloughs off and may ‘glue’ and stabilize soil aggregatesReference Wright and Upadhyaya128, Reference Wright and Upadhyaya130.
In agricultural systems, mycorrhizal fungi are vulnerable to excess fertilization or pesticide useReference Nichols, Wright, Magdoff and Weil131, Reference Rillig and Allen134, Reference Ryan and Graham135, physical destruction by tillageReference Beare, Hu, Coleman and Hendrix136 and absence of host plants, resulting in reductions in C allocation to the rhizosphereReference Rillig and Allen134, Reference Treseder and Turner137 and weakened relationship with newer crop varietiesReference Hamel and Plenchette138, Reference Sawers, Gutjahr and Paszkowski139. Excess fertilization may cause plant roots to reject AM colonization, preventing the fungus from obtaining the C it needs to live and grow. Fungicides may reduce mycorrhizal populations directly or indirectly by affecting soil organisms responsible for breaking down organic matter and minerals into the plant nutrients that AM fungi access in exchange for C. Tillage fragments fungal hyphae, making the fungus more susceptible to decomposition and reducing its ability to survive until the next growing season. Use of non-mycorrhizal crops, or crops with a weak relationship with mycorrhizal fungi, limits the amount of C the fungus receives, therefore affecting growth and activity.
Health of the grassland AM fungi relationship depends on plant diversity and community structure, and the level of plant dependence on AM fungi. As grazing intensity increases, mycorrhizal growth and activity decrease through reduction in living roots and activityReference Klumpp, Fontaine, Attard, Le Roux, Gleixner and Soussana140. Under heavy grazing or no grazing, invasive species tend to dominate, which reduces plant diversity and may reduce mycorrhizal number and diversityReference Callaway, Thelen, Rodriguez and Holben141, Reference Mummey and Rillig142. Warm-season plants are more dependent on mycorrhizal fungi than cool-season plantsReference Bentivenga and Hetrick143, Reference Hetrick, Wilson and Schwab144.
Economics
Diversification in agricultural production has economic benefits related to risk management and economy of scope. Economy of scope refers to situations where the cost of producing multiple outputs is lower for a single-integrated firm than for multiple specialized firmsReference Panzar and Willig145. This can occur with complementarities in production, such as in producing crops and livestock, where grain or forages from crop production are used for animal feed, and livestock manure is used for fertilizer, reducing the cost for both. As another example, growing crops in rotation increases yields or reduces inputs relative to growing individual crops continuously in monoculture. Research has shown that the economy of scope in agriculture can be significant. Crops and livestock were produced at a much lower cost for integrated farms in Wisconsin than in specialized farmsReference Chavas and Aliber146. The cost of joint crop and livestock production for Missouri farmers was on average 14% less than the cost of specialized crop or livestock productionReference Wu and Prato147. Integrated farms, however, were less efficient than specialized farms at adjusting the mix of inputs used in production to minimize costs. As a result, total cost efficiency tended to be lower for integrated farmsReference Wu and Prato147. Diversification can include off-farm income sources as well as different farm enterprises. Off-farm income can be critical for small farms to remain competitive with larger farmsReference Nehring, Fernandez-Cornejo and Banker148. Fernandez-Cornejo et al.Reference Fernandez-Cornejo, Mishra, Nehring, Hendricks, Southern and Gregory149 showed a 24% cost saving for corn and soybean producers with off-farm income compared with those without.
Both the WisconsinReference Chavas and Aliber146 and MissouriReference Wu and Prato147 studies showed that the economy of scope tended to decline with the size of the enterprise, so that larger farms would find it beneficial to specialize. This is also probably related to economy of scale. Economy of scale occurs when cost of production decreases with increasing farm size. In developed countries, research indicates that costs tend to decline for small farms and reach a stable lower level for intermediate to large farm sizesReference Chavas150. Economy of scale is often associated with specialization, because management of larger farms becomes more complex with more enterprises, and management of more specialized systems is easier and improves production control and efficiencyReference Chavas and Barham151, Reference Chavas and Holt152. Specialization in larger farms may also contribute to economy of scale by spreading capital costs over more units of production (although this might not be the case if the same equipment/facilities could be spread over multiple enterprises) and the ability to obtain volume discounts on input purchases. However, the trend toward greater specialization with farm size is not universal. Morrison Paul et al.Reference Morrison Paul, Nehring, Banker and Somwaru153 indicated greater benefits to diversification with larger farm size for US farms. Skolrud et al.Reference Skolrud, O'Donoghue, Shumway and Melhim154 showed that the relationship is industry-specific for Washington farms, with trends toward greater diversification with increasing farm size for wheat, beef and apple farms, but greater specialization with increasing farm size for dairies.
Risk management can be an additional driver for diversification. Farmers are generally risk averse, i.e., they are made worse off by being exposed to risk and may be willing to forgo some income for a reduction in risk. Diversification can reduce risk exposure by including income from a range of enterprises that are influenced in different ways by varying weather and market conditions. Incomes from these different enterprises are typically not perfectly correlated, so the overall variation in income is reduced. While risk management concerns may provide an incentive for agricultural diversification, some have noted that diversification to off-farm investments may be a more effective diversification strategyReference Young and Barry155. Other risk management tools including crop insurance and government programs, and marketing tools such as forward contracting and futures and options markets, may have important interactions with diversification. Availability of crop insurance and government programs has been observed to reduce incentives for diversification at the farm level for a South Dakota case farmReference Archer, Pikul, Riedell, Hanson and Krupinsky156. Others, however, found a general positive relationship between farm diversification and government payments and crop insurance for US farmsReference Mishra, El-Osta and Sandretto157. Other factors may be more important drivers of diversificationReference Pannell, Malcolm and Kingwell158. In addition to risk and economies of scope, other key drivers of diversification include non-uniformity of resource quality (e.g., different soil types might be better suited to different types of production) and resource constraints (e.g., time and equipment constraints), and that the ‘main issue raised by variability of price and production is how to respond tactically and dynamically to unfolding opportunities or threats to generate additional income or to avoid losses.’Reference Pannell, Malcolm and Kingwell158 This indicates the need for development of dynamic agricultural systems and tools for managing these systems. This would build on the dynamic cropping systems approach discussed earlier, and expand the approach to other agricultural systems.
Economy of scope may also play an important role in producers’ willingness to supply non-marketed ecosystem services. From an economic perspective, the willingness of producers to supply ecosystem services depends on whether provision of these services increases profits or reduces risks at the farm level. Many ecosystem services do not have an effect on profits or risks at the farm level, because many effects are driven by processes at a larger spatial scale than the farm level, occur primarily off-farm and (or) may be public goods where markets do not fully capture the value of these servicesReference Randall159–Reference Lant, Kraft, Beaulieu, Bennett, Loftus and Nicklow161. For example, the spatial pattern of land cover across broad geographic regions can have a large effect on the population and distribution of wildlife species. In the absence of a market for wildlife services, the wildlife produces no income for a farm situated within the landscape. Furthermore, even if a market existed for the wildlife services, the provision of these services would depend on coordinating the pattern of land use among the various farms within the landscape. These services, however, are produced jointly with marketed agricultural products. In cases where the non-marketed ecosystem services are complementary with marketed goods (economy of scope between non-marketed ecosystem services and agricultural products) producers may not need additional incentive to achieve the non-marketed servicesReference Wossink and Swinton162. This can occur for ecosystem services that provide productivity or input reduction benefits. Examples include practices that enhance pollinator habitat, soil N fixation, or enhance soil quality through increased soil organic carbon (SOC) storage and reduced erosion, and these enhancements can produce benefits beyond the farm level.
Trade-offs
Not all ecosystem services may be realized at once, hence a critical challenge is to identify and accurately assess trade-offs among desired services. In addition, achieving ecosystem service goals may produce economic and social trade-offs. For example, maintaining acceptable wildlife habitat on grassland farms may compromise the quantity and quality of forage needed for livestock production, and this has economic consequences for the livestock producer. In this instance, trade-offs among wildlife habitat, livestock production and economic return at the farm level must be decided and the trade-off assessment encumbered by practical and ethical considerationsReference Brauman, Daily, Ka'eo and Mooney163. The value of an ecosystem service must be assessed within the context of the service, and includes both social and economic values. A grassland farmer may value grassland for its provisioning services (quantity and quality of forage), whereas a grassland conservationist may value attributes such as appropriate plant diversity and vegetation structure that support bird populations. Identifying trade-offs among the various dimensions of sustainability for alternative production systems provide a transparent mechanism for decision makers to determine an appropriate balance among competing goalsReference Weersink, Jeffrey and Pannell164. Trade-offs can be strongly influenced by spatial variation in resourcesReference Antle and Capalbo165, Reference Antle and Stoorvogel166, as well as dynamics over time.
Increasing bioenergy production from agricultural land may require trade-offs in land use. In the USA, projections of expanded ethanol and biodiesel production suggest large reductions in pasturelandReference De La Torre Ugarte, English and Jensen167. A global analysis of bioenergy production suggested that shifting livestock production from pasture to confinement feeding would reduce land needs for agricultureReference Taheripour, Hertel and Tyner168. If these changes in land use are realized, there may be pressure to intensify inputs and management on remaining hay, forage and pastureland in the future169. The expanding need for biomass production would probably force forage and grazing livestock production on to more marginal lands with potential trade-offs in soil and water quality.
Monitoring changes in land use, especially in grassland, and in cropping diversity presents a significant challenge. The cropland data layer of the National Agricultural Statistics Service is by far the most extensive spatial dataset depicting the major crop-specific land cover on the entire conterminous USA. But even the cropland data layer generalized grassland into broad categories such as pasture/grass and other hays. Broad categorizations of some of these land uses possess inaccuracy in quantifying ecosystem services and mapping essential resourcesReference Naidoo, Balmford, Costanza, Fisher, Green, Lehner, Malcolm and Ricketts170. A more specific baseline data for monitoring grassland and pastureland would pave the way for better resource management, reliable detection and intervention of land use conversion and accurate assessment of ecosystem services.
An alternative for increasing bioenergy production without shifting land use is utilizing crop residues as feedstocks. A field-level case study analysis of the potential for harvesting corn stover for bioenergy production showed soil organic C losses with corn stover harvest using a traditional tillage system. However, by switching from conventional tillage to no-till, soil organic C could be increased while harvesting biomass and increasing farm profitsReference Anand, Archer, Bergtold, Canales, Braun, Karlen and Johnson77.
Similar cases have been identified where economic returns and provision of ecosystem services may be both improved. For example, in irrigated crop production, research showed opportunities to reduce net greenhouse gas emission and increase economic returns by avoiding excess application of N fertilizer and by switching from conventionally tilled continuous corn to a no-till corn–bean rotationReference Archer and Halvorson171. However, achieving further reductions in net greenhouse gas would reduce farm profitability. This is a common observation where market forces lead producers to maximize profitability, and the economic optimum and environmental optimum typically do not coincide. As a result, achieving increases in ecosystem services often comes at a cost to farm profitability.
This trade-off between ecosystem services and economic returns could be reduced if ecosystem services provided an economic benefit to the farmer. This may occur if markets are developed where farmers would be compensated for the value of ecosystem services they provided. Also, as indicated earlier, this may occur when the ecosystem services provide input-reducing or productivity benefits. Diverse integrated agricultural systems, where inputs from one enterprise come from products of another enterprise, and which rely on well-functioning ecosystem services such as disease and pest suppression, and nutrient and water cycling, are likely to realize these input-reducing and productivity benefits. Diverse integrated systems can reduce the trade-offs, and in some cases provide win–win opportunities for simultaneously increasing agricultural profitability and ecosystem services.
Conclusions
In this synthesis, we demonstrated that diversifying agricultural systems via increasing plant species in managed grasslands and combining several crop species into logical sequences and dynamic rotations can enhance crop production, the use of natural resources and in some instances improve economic returns. However, much of the research documenting potential benefits of diversification is based on studies focused on a single ecosystem service. There is a great need for research comparing various systems and measuring multiple ecosystem services in multiple environments. This will enable analyses of potential trade-offs among services and will better inform management and policy guidelines. Owing to the increased management intensity required by integrated systems based on diversification, agriculturalists need to be convinced that these systems are economically and environmentally sustainable to ensure adoption. Research is needed that quantifies the economic and environmental performance of integrated systems, including both short-term and long-term effects. Ideally, such research would actively engage farmers in the research process and result in useful management guidelines and tools to successfully promote and implement integrated systems.
Achieving global food security would seem to argue for agricultural systems focused solely on production to meet the need for human food. To sustain, and not just meet, food production needs, however, requires the conservation of natural resources that support agricultural systems. Conservation agricultural systems that rely on diversity in plants, animals and soil micro-organisms at multiple scales can sustain production and provide additional ecosystem services such as soil C storage, efficient nutrient cycling and conservation of biodiversity.