Introduction
Agriculturalists are faced with challenges relating to very pressing environmental and health issues, including the need to decrease pesticide use. In many countries, high levels of pesticides have frequently been found in rivers, lakes and groundwater 1 , Reference Schrack, Coquil, Ortar and Benoît 2 . A second pressing environmental issue is the consumption of fossil fuel. Energy use has markedly increased over the past decadeReference Höök, Sivertsson and Aleklett 3 , and some scientists agree that oil availability will decline in the near futureReference Jakobsson, Söderbergh, Höök and Aleklett 4 , leading to a sharp increase in oil pricesReference Shafiee and Topal 5 . In this context, new ways of optimizing or reducing energy use have been proposedReference Mohr and Evans 6 . Global warming is a third challenging environmental issue facing agriculture. About 12% of global greenhouse gas (GHG) emissions emanate from agricultural landsReference Smith, Martino, Cai, Gwary, Janzen, Kumar, McCarl, Ogle, O'Mara, Rice, Scholes, Sirotenko, Metz, Davidson, Bosch, Dave and Meyer 7 , and this proportion is expected to rise in the future, due to increases in the amount of land used for agricultural purposes and the intensification of agricultural practicesReference Galloway, Townsend, Erisman, Bekunda, Cai, Freney, Martinelli, Seitzinger and Sutton 8 . Carbon (C) sequestration in the soil, through the return of crop residues, root deposition and organic amendments, may help to decrease GHG emissionsReference Arrouays, Balesdent, Germon, Jayet, Soussana and Stengel 9 . Sustainable development is another pressing social issue. Sustainable agriculture must satisfy environmental criteriaReference Spiertz 10 . The harmful impact of agriculture on the environment can be lessened by optimizing fertilization (N, P) and increasing crop diversity. Currently pesticide use and energy consumption should also be reduced 1 , Reference Schrack, Coquil, Ortar and Benoît 2 , and soil fertility should be maintained. Since the 1950s, alternative crop management systems have been proposedReference Matson, Parton, Power and Swift 11 and legislation and inspection services have controlled the use of inputs (pesticides, N fertilization). Finally, agricultural production must satisfy the food needs of a soaring world populationReference Spiertz 10 . Global agricultural production currently feeds a population of approximately 7 billion. Current projections suggest that the world population will have reached 9 billion by 2050 12 . The resulting increase in the need for land for housing will reduce the amount of land available for agricultural purposesReference Bouman, Humphreys, Tuong and Barker 13 . The availability of arable land per capita differs greatly between regions (e.g., between China and South America), and major cropping systems must take this scarcity into account. Foley et al.Reference Foley, Ramankutty, Brauman, Cassidy, Gerber, Johnston, Mueller, O'Connell, Ray, West, Balzer, Bennett, Carpenter, Hill, Monfreda, Polasky, Rockström, Sheehan and Siebert 14 have suggested that feeding a population of this size will be possible only if agricultural systems change, along with human eating habits.
Many studies in recent years have focused on the design and assessment of new cropping systems. New crop management strategies have been proposed to decrease pesticide useReference Aubertot, Barbier, Carpentier, Gril, Guichard, Lucas, Savary, Savini and Voltz 15 , to decrease energy consumptionReference Köpke and Nemecek 16 , or to enhance C sequestration through crop management practicesReference Beheydt, Boeckx, Ahmed and Van Cleemput 17 . Energy use and GHG emissions have been calculated and assessed for different systemsReference Van Kessel, Ventera, Six, Arlene, Borde, Linquist and Van Groenigen 18 . At the cropping system level, long-term trials have also been set up to investigate the effects of different technical operations, such as N fertilization, on soil physical and chemical propertiesReference Fu, Tunney and Zhang 19 and on soil biologyReference Heinze, Raupp and Joergensen 20 . Several studies have assessed the differences between organic, integrated and conventional cropping systems in terms of C sequestrationReference Chirinda, Olesen, Porter and Schjønning 21 , energy efficiency and useReference Tuomisto, Hodge, Riordan and Macdonald 22 , profitabilityReference Shadbolt, Kelly, Horne, Harrington, Kemp, Palmer and Thatcher 23 or productivityReference De Ponti, Rijk and Van Ittersum 24 . Some authors have analyzed the impact of different degrees of tillage on productivityReference Simonsen, Posner, Rosemeyer and Baldock 25 or biological activityReference Riley, Pommeresche, Eltun, Hansen and Korsaeth 26 . Others have focused on the effect of cropping systems on biodiversityReference Snapp, Gentry and Harwood 27 , or have used life cycle assessment methods to analyze the sustainability of various farming systemsReference Nemecek, Dubois, Huguenin-Elie and Gaillard 28 . In most of these cases, new cropping systems were designed by modifying a few agricultural practices targeting a single goal (e.g., no chemicals to be used in organic cropping systems; no plowing to increase C sequestration; more legumes in the rotation to reduce energy consumption; and more inputs to enhance profitability), without considering the other dimensions of sustainability. Despite the evidence that the future of agriculture must address a wide range of issues, no study has designed innovative cropping systems with specific and quantitative objectives covering a broad range of issues.
The objective of our project was to design, by prototypingReference Vereijken 29 , innovative cropping systems meeting three quantitative objectives: (1) to satisfy a major environmental constraint, which represents a major break regarding objectives to be reached in current cropping systems (the banning of pesticide use, reducing fossil energy consumption by 50% or reducing gas emissions by 50%); (2) to satisfy a wide range of environmental criteria with specific quantitative targets; and (3) to produce the maximum yield possible given the constraint and the environmental targets. The ultimate aim of this work is to improve arable cropping systems throughout northern Europe. Prototyping was developed to enable agronomists to design, test and improve more sustainable cropping systemsReference Vereijken 29 . With this approach, newly designed cropping systems could satisfy several of the issues mentioned above and contributed to identifying the weaknesses of cropping systems. System prototypes were assessed with tools and a model (ex ante assessment), with discussion of their potential performances, before their assessment in a long-term field experiment (ex post assessment). We set up this long-term field experiment in 2008, and its results will be published in due course. We focus here on the prototyping and assessment of the cropping system prototypes. We discuss our results in terms of innovative design methodology, the innovation of agricultural practices, the availability of suitable tools, models and crop management, and the yields achievable.
Materials and Methods
Design method
The method used for cropping system design was based on the prototyping approachReference Vereijken 29 – Reference Lançon, Wery, Rapidel, Angokaye, Gérardeaux, Gaborelc, Ballo and Fadegnon 32 , which involves four major steps:
-
(i) defining and ranking the constraints and targets;
-
(ii) designing innovative cropping system prototypes on the basis of current knowledge;
-
(iii) assessments of cropping system prototypes with tools and models adapted for the constraints and targets used, with improvement of the cropping systems (in terms of rotation or crop management aspects) by an iterative process, until satisfaction of the constraints or achievement of results considered the best possible; and
-
(iv) assessment of the most promising cropping system candidates in a long-term field trial. This practical assessment is currently being carried out in a long-term field experiment, initiated in 2008.
Constraints and targets for innovative cropping systems
Four different cropping systems with quantified constraints (i.e., conditions that had to be fulfilled), environmental and yield targets, were designed for the agricultural conditions and principal crops of northern France. These constraints and targets were prioritized as follows: an environmental constraint had to be satisfied first; a set of environmental targets then had to be attained, and finally, yield had to be maximized. The quantitative levels of the constraints did not correspond to any regulations and reflected a major break to be reached in current cropping systems. Inclusion of the use of organic fertilizers (manure, compost, etc.), which are currently not readily available to many farmers in large areas of western Europe, was not permitted in the design of the cropping systems.
Productive high environmental performance (PHEP) cropping system
No major environmental constraint was placed on this cropping system, which was designed to reach environmental targets. Eleven environmental indicators, according to the INDIGO® toolReference Bockstaller, Guichard, Makowski, Aveline, Girardin and Plantureux 33 , were used to assess the effects of the cropping system on groundwater pollution (nitrate and pesticides), crop diversity, energy use and soil quality (organic matter content and phosphorus concentration). To reach environmental goals, all these environmental indicators, calculated over an entire crop rotation sequence, had to have values higher than 7 (graduated scale from 1 to 10)Reference Bockstaller, Guichard, Makowski, Aveline, Girardin and Plantureux 33 . This system was used as the reference system for comparisons with the other three systems.
No-pesticide (No-Pest) cropping system
This cropping system was subject to a specific pesticide constraint: no pesticide use was tolerated, even using substances (e.g., acetic acid) at levels usually considered acceptable in organic cropping systems. However, inorganic chemical fertilizers were allowed (these fertilizers are not permitted in organic farming systems). This system had to achieve the same environmental targets as the PHEP cropping system.
Low-energy (L-EN) cropping system
This cropping system was subjected to a specific energy constraint: it had to have fossil fuel consumption levels no greater than half those of the PHEP cropping system. It had to reach the same environmental targets as the PHEP cropping system.
Low-GHG (L-GHG) emission cropping system
This cropping system was subject to a specific constraint concerning GHG emissions: its GHG emissions had to be no more than half those of the PHEP cropping system by increasing C sequestration in the soil and decreasing nitrous oxide (N2O) emissions. It had to meet the same environmental targets as the PHEP cropping system.
For each cropping system, once the constraint had been satisfied and environmental targets had been reached, the combination of agricultural practices giving the highest yields was retained.
Design of the four innovative cropping systems
The innovative cropping systems were designed from published knowledge, quantitative data from field experiments and individual or group expertise provided by scientists, extension service staff and farmers. For each cropping system, one prototype, consisting of the species in the rotation and the combination of agricultural practices used, was designed. If the constraints were not satisfied, the candidate was modified iteratively (changes to the crops in the rotation or agronomic practices) until they were. At the beginning of the process, a modification of a crop led to a multitude of changes; at the end, changes were only one at a time. When the constraints were satisfied, environmental targets were optimized by an iterative procedure until improvement was observed. Maximum achievable yields were then determined by expert knowledge or from trial results, for the various cropping systems. The candidate cropping systems selected for further assessment in a field experiment were those with the best performances in terms of constraints, environmental targets and achievable yields.
We carefully selected agronomic strategies from previous publications, to satisfy the given constraints. Examples of such strategiesReference Smith, Martino, Cai, Gwary, Janzen, Kumar, McCarl, Ogle, O'Mara, Rice, Scholes, Sirotenko, Metz, Davidson, Bosch, Dave and Meyer 7 , Reference Arrouays, Balesdent, Germon, Jayet, Soussana and Stengel 9 , Reference Aubertot, Barbier, Carpentier, Gril, Guichard, Lucas, Savary, Savini and Voltz 15 , Reference Beheydt, Boeckx, Ahmed and Van Cleemput 17 , Reference Munier-Jolain, Faloya, Davaine, Biju-Duval, Meunier, Martin and Charles 34 – Reference Pelster, Larouche, Rochette, Chantigny, Allaire and Angers 45 are presented in Table 1. Current knowledge, based on conventional cropping systems, had to be adapted for innovative cropping systems, and it was necessary to combine strategies. Agronomic strategies were translated into decision rules (as described by DebaekeReference Debaeke, Munier-Jolain, Bertrand, Guichard, Nolot, Faloya and Saulas 46 ) to meet the requirements of future cropping systems and to cope with the variability of weather and agronomic conditions.
Table 1. Examples of published agronomic strategies and associated practices for achieving specific goals.

Assessment with tools and a model and fine-tuning of innovative cropping system prototypes
During the design process, the cropping system prototypes were assessed with various tools and a model, to determine the best ways to satisfy the set of constraints and targets imposed. Direct and indirect non-renewable energy consumption were assessed with the INDIGO® tool (v. 1.9). Direct energy consumption concerned the fuel, lubricants and electricity used to power farm machinery and tractors. Indirect energy consumption concerned the energy used in the manufacture, formulation, packaging and maintenance of inputs, such as machinery, fertilizer or pesticides. The energy outputs of the cropping systems were calculated as the gross energy content of the harvested produce. Energy consumption was calculated on a per hectare basis, per ton of crop product and per calorie produced, over a complete crop sequence.
C sequestration in the soil was assessed with (1) the Roth C 26.3 modelReference Coleman and Jenkinson 47 and (2) the SIMEOS® tool (v. 2010) based on the AMG modelReference Andriulo, Mary and Guerif 48 . We used climatic data (i.e., monthly mean air temperature, monthly precipitation and monthly open pan evaporation) from a meteorological station located in Grignon (Ile-de-France region, 30 km west of Paris). The soil characteristics (plow layer, 0–30 cm) used to drive simulations were as follows: clay content 20.6%, bulk density 1.4, initial C content 8 g kg−1 dry matter. The expected annual yields were estimated from experimental data obtained under the same conditions (i.e., Ile-de-France region) and adjusted by expert knowledge. These values were used to estimate the expected annual dry matter production of roots and stubble, as described by Van Groenigen et al.Reference Van Groenigen, Hastings, Forristal, Jones and Smith 49 . Direct and indirect GHG emissions were estimated with the GES'TIM database 50 . We focused on two main GHGs: N2O and carbon dioxide (CO2). Direct emissions included N2O emissions from N fertilizers, calculated with Intergovernmental Panel on Climate Change coefficients 51 , and the CO2 produced by the combustion of fossil fuels by farm machinery; CO2 respired by soil organisms was not taken into account in calculations. Indirect emissions corresponded to the use of fossil energy in the manufacture and maintenance of farm inputs. GHG balances (C sequestration plus GHG emissions) were determined over periods of 25 and 50 years, in accordance with Intergovernmental Panel on Climate Change proposals 51 and current knowledge of C sequestration kinetics in the soil. In this investigation, any GHG entering the system is counted negatively whereas GHG leaving the system is counted positively. Therefore, the overall balance is a positive value if more GHGs are emitted than sequestered in the system.
Environmental indicators, such as in the INDIGO® tool (v. 1.9), were used to assess the environmental effects of cropping system prototypes. Three indicators of nitrogen effects provided information about ammonia volatilization, N2O emissions into the air, and nitrate leaching into the groundwater. Four pesticide indicators were studied: three providing information about pesticide volatilization, pesticide runoff and pesticide leaching into groundwater and one taking the global effect of pesticides into account. The final four indicators provided information about crop diversity, energy consumption, organic matter in the soil and phosphorus management. Each indicator takes a value between 1 (worst) and 10 (best). For rotations of more than five crops, the crop diversity indicator was calculated from the coefficients of LeteinturierReference Leteinturier, Herman, de Longueville, Quintin and Oger 52 . For example, values 0.5, 4.1 and 7.6, respectively, correspond to a wheat monoculture, a wheat–maize rotation and a wheat–sunflower–spring barley–maize rotation.
All these tools and the model were chosen on the basis of their relevance for assessing compliance with constraints and environmental targets. In a comparison of the performance of nine soil organic C models, using different datasets from long-term experiments from different parts of the world, Smith et al.Reference Smith, Smith, Powlson, McGill, Arah, Chertov, Coleman, Frnko, Frolking, Jenkinson, Jensen, Kelly, Klein-Gunnewiek, Komarov, Li, Molina, Mueller, Parton, Thornley and Whitmore 53 found that the Roth C model was among those that performed best. Bockstaller et al.Reference Bockstaller, Guichard, Keichinger, Girardin, Galan and Gaillard 54 analyzed four methods for assessing the sustainability of agricultural systems. They found that the INDIGO® tool was the most relevant for conditions corresponding to those used here. These tools and the model have been regularly used in different countries. For example, Roth C has been used by Liu et al.Reference Liu, Chan, Conyers, Li and Poile 55 and Cerri et al.Reference Cerri, Easter, Paustian, Killian, Coleman, Bernoux, Falloon, Powlson, Batjes, Milne and Cerri 56 , and INDIGO® has been used by Bockstaller et al.Reference Bockstaller, Guichard, Keichinger, Girardin, Galan and Gaillard 54 .
Current cropping system in the Ile-de-France region
The current system in the Ile-de-France region was defined on the basis of data collected in 2006 (Agreste 57 ), the most recent data available at the initiation of this program. We defined the current cropping system in terms of agronomic practices and crop descriptions. This system was validated by various experts (farmers, extension service staff) with few adjustments in terms of types and numbers of crops in the rotation. This system was used as a reference for further comparisons.
Results
Design methodology step
The systems were designed over a 6-month period, by a panel of about 15 experts. For each innovative cropping system, the first candidate characteristics were based on the current cropping system in the Ile-de-France region. The system was then optimized through an iterative process, which produced approximately 70 prototypes, to find the four most promising candidates. These candidates corresponded to the prototypes satisfying the constraint and environmental targets imposed and yielding the best results in the assessment. For example, for the PHEP system, the value of the crop diversity indicator was gradually increased from 5 to 7 during the fine-tuning of the system, with simultaneous improvement of the values of the other indicators. The first prototype was based on a 3-year rotation (winter oilseed rape, winter barley and winter wheat), currently used in the Ile-de-France region. In the best prototype, a winter legume and spring barley with a mustard catch crop were gradually introduced, leading to the following rotation: winter field beans (Vicia faba), winter wheat, winter oilseed rape, winter wheat and spring barley with a mustard catch crop. In the design process, we began by determining the crop rotation and then defined the crop management practices.
Description of the innovative cropping system prototypes
For each cropping system, we present only the most promising prototype. The systems are first described in terms of the crop rotation, crop management practices and yield targets. We then present the results of the final assessment with respect to constraints (i.e., pesticide use, energy consumption and GHG emissions) and, finally, we evaluate the systems in terms of environmental targets. The crop rotations and targeted yields are presented in Tables 2 and 3.
Table 2. Crop rotations of the innovative cropping systems designed, and a current cropping system, defined by experts on the basis of data from the Ile-de-France region in 2006 (Agreste 57 ).

Table 3. Targeted yields (t ha−1) for different species sown in the innovative cropping systems and comparison with current yields. Cropping systems: PHEP (productive high environmental performance), No-Pest (no-pesticide use), L-EN (low-energy use), L-GHG (low-greenhouse gas emissions) and IdF (current system in Ile-de-France region).

The productive PHEP
The PHEP cropping system was designed with multiple environmental targets in mind and was based on the following agronomic strategies: (1) to reduce pesticide use, we increased crop diversity (four different crops instead of the three currently sown); (2) to reduce the amount of N used and indirect energy consumption, we included at least one legume in the rotation; (3) to decrease nitrogen leaching, a catch crop was always sown before the spring crop and N fertilization was forbidden during autumn and winter; (4) to reduce direct energy consumption, plowing was allowed only once in the rotation, before the spring crop; (5) to reduce pesticide use and crop loss due to insects and diseases, highly resistant varieties were used, together with optimal sowing dates and densities; and (6) to stabilize or/and to enrich the soil organic matter (SOM) content of the soil, crop residues were not removed. As the system had to satisfy environmental targets requiring the use of fewer inputs, the target yields set were similar to those currently achieved with low-input cropping systems in the Ile-de-France region.
The No-Pest cropping system
Pesticide use was prohibited in the ‘No-Pest’ cropping system. Therefore, this cropping system was designed as follows: (1) to break the cycles of some common soil-borne pathogens, we used a long rotation including a range of species (five different crops), with the alternate sowing of host and non-host plants; (2) to reduce weed emergence from year to year, we sowed species with different sowing dates in spring and in winter successively; (3) to decrease pest and disease pressure and damage, we used highly resistant varieties and species mixtures, and excluded crops highly susceptible to some enemies but with few non-chemical solutions, such as oilseed rape or potatoes, from the rotation; (4) to increase the competitiveness of the crop with respect to weeds, we sowed species with rapid shoot growth, such as hemp and triticale; (5) to maximize weed emergence before sowing, we used the stale seed-bed technique; (6) to reduce weed emergence after sowing, plowing was carried out before each spring crop; and (7) we adapted sowing densities to make it possible to use mechanical weeding techniques and to decrease pathogen propagation. We used the following approaches to reach environmental targets: (1) to reduce nitrate leaching, catch crops were always sown before spring crops and the spreading of nitrogen fertilizer was allowed only in the spring; (2) to decrease direct and indirect energy consumption, we decreased the number of plowing events and N fertilization was calculated according to yield objectives; and (3) to stabilize SOM, crop residues were not removed. Yield targets were lower than those for the PHEP cropping system, because no pesticides were used. However, they were higher than those achieved in organic systems because chemical fertilizers were allowed, increasing flexibility in the management of crop nitrogen nutrition. For the integration of these features, in accordance with current knowledge of pest and disease pressures in the Ile-de-France region, experts suggested yield potentials 30% lower than those for the PHEP system for cereals and 25% lower for field beans.
L-EN cropping system
The L-EN cropping system was designed, to have a much lower energy consumption than the PHEP cropping system, as follows: (1) to reduce indirect fuel consumption due to N fertilization, we included as many legumes as possible in the rotation (field beans as a main crop, clover as a catch crop and a white clover-winter wheat mixture), and we used species or varieties with high N use efficiency (e.g., oatsReference Riley, Hoel and Kristoffersen 58 ) and forms of mineral N fertilizers requiring less energy for their manufacture; (2) to decrease direct fuel consumption, we omitted plowing, which is a very resource-intensive operation, and used a direct drilling system; and (3) we decreased the amounts of mineral fertilizer (N, P and K) applied, implying a decrease in target yields. We also designed the L-EN cropping system along the same lines as the PHEP system, to achieve environmental targets for crop diversity, length of rotation, date of nitrogen spreading and catch crop sowing. Target yields were 20% lower than for the PHEP cropping system, except for field beans.
L-GHG emission cropping system
The L-GHG cropping system was designed to decrease GHG emissions by increasing C sequestration in the soil and decreasing N2O emissions.
C sequestration in the soil was increased by: (1) including as many cereals as possible in the rotation, to ensure the production of large amounts of residues (i.e., maize, winter wheat, winter barley or triticale); (2) maintaining continuous soil cover to increase the amounts of organic residues (i.e., cover or catch crops were always sown between main crops, and volunteers were left to grow after harvest); (3) targeting high yields for the main and catch crops, to ensure the production of large amounts of residues; and (4) excluding moldboard plowing, which increases C mineralization.
N2O emissions were reduced by: (1) decreasing the amount of N fertilizer required at rotation scaleReference Jeuffroy, Baranger, Carrouée, de Chezelles, Gosme, Henault, Schneider and Cellier 59 , and consequently direct emissions of N2O, by sowing legumes in the rotation (main and catch crops); (2) improving and optimizing N fertilization practices according to climatic conditions, through the use of appropriate decision rules to prevent applications in conditions favoring N2O emissions; and (3) sowing species with taproots to reduce soil compaction and N2O emissions.
The L-GHG cropping system was also designed according to the same principles as the PHEP system, to reach environmental targets for crop diversity, length of rotation, pesticide use, date of N spreading and catch crop sowing. Target yields were considered to be a compromise between the production of large amounts of C residues (i.e., high yields) and the decrease in N2O emissions (i.e., low N fertilization). Experts thought that potential yields would be similar to those achieved by the PHEP cropping system.
The current cropping system in the Ile-de-France region
This system is based on a cereal crop rotation, with five cereal crops over a 6-year rotation (Table 2). To secure high yields, the agronomic practices were as follows: regular plowings, four times over a 6-year rotation. The amounts of N fertilizer exceed crop requirements, to prevent yield shortfalls in the event of unfavorable climatic conditions or unexpected nitrogen losses. Pesticides and growth regulators were used liberally to prevent diseases, weeds, pests and lodging (three to five pesticides every year).
Cropping System Prototypes Assessments with Tools and a Model
Constraint assessment
Pesticide constraint in the No-Pest cropping system
This constraint was achieved by not applying pesticides in the No-Pest cropping system.
Energy constraint in the L-EN cropping system
Mean total fossil energy consumption (direct and indirect energy), calculated over a single rotation, was 4517 MJ ha−1 yr−1 for the L-EN system and 8826 MJ ha−1 yr−1 for the PHEP system (Fig. 1). Chemicals, including N fertilizers, the largest component, accounted for 1271 MJ ha−1 yr−1 (43% of total indirect energy consumption) in the L-EN system and 4345 MJ ha−1 yr−1 (95% of total indirect energy consumption) in the PHEP system. The use of machinery for tillage, fertilization, harvesting and sowing and crop protection was the only component of direct energy consumption that was nearly halved in the L-EN system (2976 MJ ha−1 yr−1 and 4228 MJ ha−1 yr−1 for the L-EN and the PHEP systems, respectively). The difference between these two systems can be accounted for by the absence of tillage and the use of less N fertilizer in the L-EN cropping system.
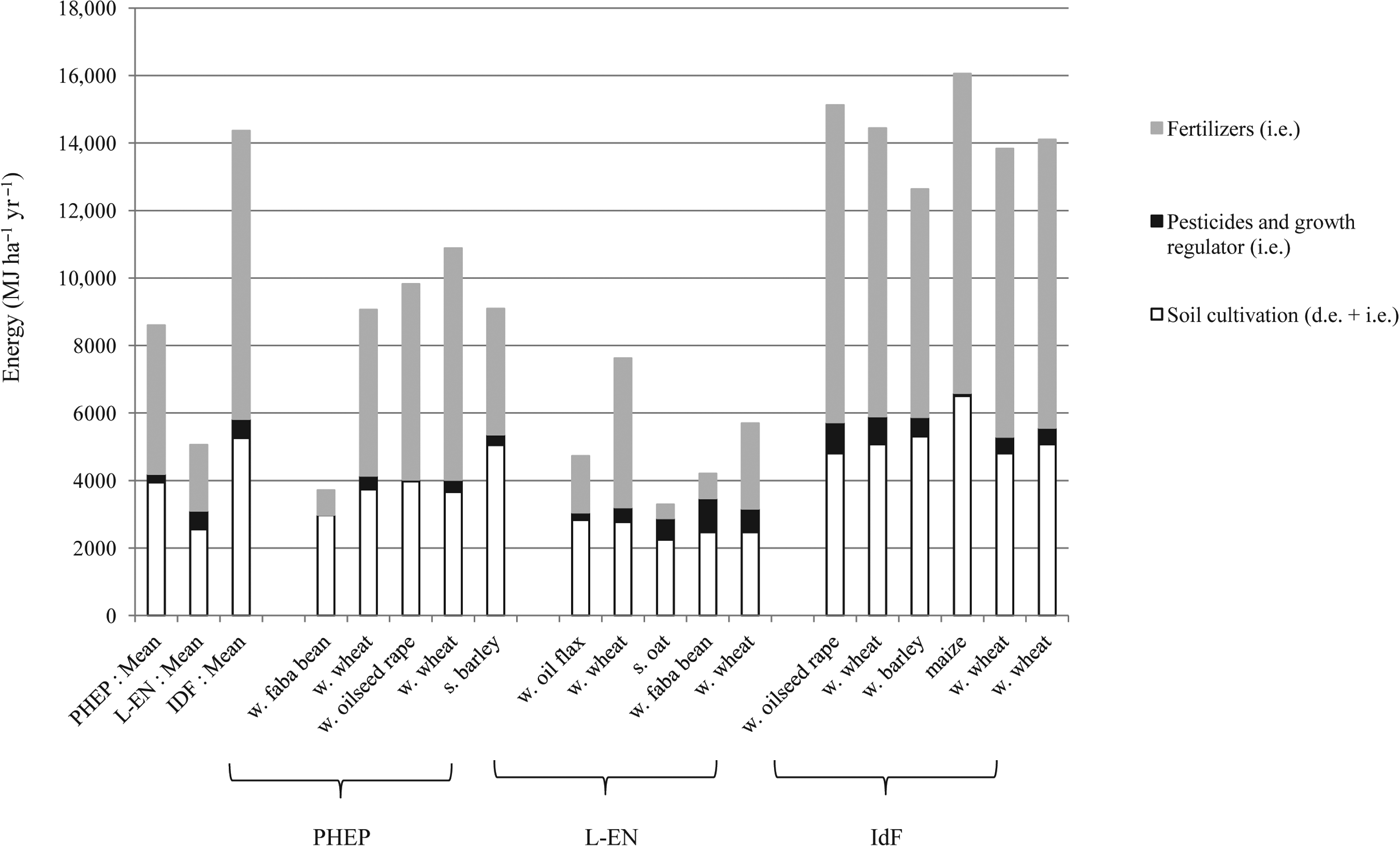
Figure 1. Cropping system prototypes assessment with GES'TIM database: mean total fossil energy consumption (expressed in MJ ha−1 yr−1) calculated over one rotation period for the different cropping systems, and total fossil energy consumption for each crop of the various cropping systems (i.e., indirect energy; d.e., direct energy; w, winter; s, spring). Cropping systems: PHEP (productive high environmental performance), L-EN (low-energy use) and IdF (current system in Ile-de-France region).
When expressed in MJ ha−1, the total fossil energy in the L-EN system is 49% lower than that in the PHEP system (Table 4). However, if expressed in MJ t−1, the energy performance of the L-EN system is lower (i.e., difference between the PHEP and the L-EN systems of only 24% in term of total fossil energy per ton of produce), because the target yield is about 20% lower. A similar reduction in energy use (about 29%) was observed for the calculation in kJ kcal−1.
Table 4. Comparisons of total fossil energy consumption, expressed in MJ ha−1, MJ t−1 and kJ kcal−1 (INDIGO® tool, v.1.9), between cropping systems. Cropping systems: PHEP (productive high environmental performance), L-EN (low-energy use) and IdF (current system in Ile-de-France region).

The GHG constraint in the L-GHG cropping system
C sequestration was assessed for the optimized prototypes of the PHEP and the L-GHG systems, for the mean SOM content in the Ile-de-France region (1.6%). Both the Roth C model and the SIMEOS® tool predicted that C would be sequestered throughout the study period, from the start, in both cropping systems. The highest values were obtained with the L-GHG system over a 50-year period, for both assessment tools (Table 5). For both systems, total C sequestration was higher during the first 25-year period than during the second 25-year period. When expressed in t CO2-eq ha−1, C sequestration values were systematically higher with the Roth C model than with the SIMEOS® tool. Nevertheless, after 25 and 50 years, the differences between the L-GHG and the PHEP systems calculated with the Roth C model and the SIMEOS® tool were similar if the results were expressed in relative values.
Table 5. C sequestration (t CO2-eq ha−1) simulated over 25-year and 50-year periods for the different cropping systems with the ROTH C model (V.26-3) and the SIMEOS® tool (2010), for mean SOM content in the Ile-de-France region (1.6%. SOM=123.3 t CO2-eq ha−1). Cropping systems: PHEP (productive high environmental performance), L-GHG (low greenhouse gas emissions) and IdF (current system in Ile-de-France region).

Direct and indirect GHG emissions were calculated with the GES'TIM database, over one rotation period, for the L-GHG and the PHEP cropping systems (Fig. 2). Mean total GHG emissions were 1104 kg CO2-eq ha−1 yr−1 and 1273 kg CO2-eq ha−1 yr−1 for the L-GHG and the PHEP cropping systems, respectively. Direct and indirect GHG emissions accounted for similar proportions of total emissions: 48 and 52% for direct GHG emissions for the L-GHG and PHEP systems, respectively (Table 6). Chemical fertilizers caused both direct and indirect GHG emissions. They represented 76 and 73% of total GHG emissions for the L-GHG and the PHEP systems, respectively. Soil cultivation, accounting for 19 and 23% of total GHG emissions for the L-GHG and the PHEP systems, respectively, was the second most important component of these emissions. When results were expressed per ha, total GHG emissions were 13% lower in the L-GHG system than in the PHEP system. When expressed per ton of produce, the larger decrease (22%) may be accounted for by the higher yields, calculated at rotation scale, of the L-GHG system than of the PHEP system.

Figure 2. Cropping system prototypes assessment with GES'TIM database: Mean total greenhouse gas emissions (expressed in kg CO2-eq ha−1 yr−1) calculated over one rotation period for the different cropping systems, and total greenhouse gas emissions for each crop of the various cropping systems (i.ghg, indirect greenhouse gas; d.ghg, direct greenhouse gas; w, winter; s, spring). Cropping systems: PHEP (productive high environmental performance), L-GHG (low greenhouse gas emissions) and IdF (current system in Ile-de-France region).
Table 6. Comparison of total simulated GHG emissions, expressed in t CO2−eq ha−1 and t CO2−eq t−1, between cropping systems. Cropping systems: PHEP (productive high environmental performance), L-GHG (low greenhouse gas emissions) and IdF (current system in Ile-de-France region).

In terms of the overall balance of GHG emissions (Tables 7 and 8), GHG values were negative for the L-GHG system, except for the 50-year period with the SIMEOS® tool. All GHG balance values were lower for the L-GHG system than for the PHEP system. The difference in GHG balance between the two systems increased over time, and was greater with the Roth C model, which gave decreases in GHG emission of 51% for the 25-year period and 76% for the 50-year period.
Table 7. Greenhouse gas balances (t CO2-eq ha−1), for different cropping systems, determined with the SIMEOS® tool (2010) and the ROTH C model (V.26-3), for a current soil in the Ile-de-France region (SOM=1.6%) and the GES'TIMReference Van Groenigen, Hastings, Forristal, Jones and Smith 49 database, for 25-year and 50-year periods. The GHG balance is positive when the amount of C emitted in greenhouse gases exceeds that sequestered. Cropping systems: PHEP (productive high environmental performance), L-GHG (low-greenhouse gas emissions) and IdF (current system in Ile-de-France region).

Table 8. Greenhouse gas balance ratio of the various cropping systems, expressed in t CO2-eq ha−1 , simulated with the ROTH C (V.26-3) model over two different periods (25 and 50 years). Cropping systems: PHEP (productive high environmental performance), L-GHG (low greenhouse gas emissions) and IdF (current system in Ile-de-France region).

Assessment of environmental targets
The results of assessments of environmental targets with the INDIGO® tool are shown in Figure 3. For all optimized cropping systems, all 11 indicators had values of at least 7 (i.e., environmental criteria were satisfied), except for the organic matter indicator (OMI) for the No-Pest cropping system (OMI=5.7). The large number of species (more than three in each rotation) and the small quantities of pesticides sprayed on crops (0–2 pesticides used per crop), the systematic restitution of residues and the optimization of tillage and fertilization management (i.e., the small number of plowing operations, optimizing P and N fertilization in terms of both the amounts applied and the timing of applications), resulted in high values for the indicators for crop diversity, pesticide use, SOM, phosphorus, nitrogen and fossil fuel, respectively.

Figure 3. Environmental criteria assessment of the different cropping system prototypes with the INDIGO® tool (v.1.9). Cropping systems: PHEP (productive high environmental performance), L-GHG (low greenhouse gas emissions), L-EN (low-energy use), No-Pest (No pesticides) and IdF (current system in Ile-de-France region). Minimum values to satisfy environmental targets.
The low value of the OMI for the No-Pest cropping system (5.7) could be accounted for by both the large number of plowing operations (alternate years), encouraging mineralization and the lower yields, resulting in smaller amounts of C residues.
Discussion
Design and assessment of innovative cropping systems
The main challenge of this study was to design innovative cropping systems. Our approach is original in the multiplicity of purposes assigned to these systems (i.e., association of one major constraint with environmental and yield targets). In most previous studies, these issues have been analyzed separately. For example, Zentner et al.Reference Zentner, Lafond, Derksen, Nagyd, Wall and May 60 and Gelfand et al.Reference Gelfand, Snapp and Robertson 40 studied energy efficiency, whereas Nemecek et al.Reference Nemecek, Dubois, Huguenin-Elie and Gaillard 28 used life cycle assessment methods to evaluate environmental criteria, NowackiReference Nowacki 61 studied profitability, and Chikowo et al.Reference Chikowo, Faloya, Petit and Munier-Jolain 35 studied new cropping systems with a lower reliance on pesticides. However, several other studies are currently investigating system sustainability including assessments of several different criteriaReference Blazy, Ozier-Lafontaine, Doré, Thomas and Wery 62 , or numerous environmental parametersReference Tuomisto, Hodge, Riordan and Macdonald 22 . In our project, we combined one major constraint with environmental and yield targets, reflecting the multifunctionality of agriculture. Furthermore, there was a clear, particular hierarchy throughout the design process. In most published experiments, environmental consequences are assessed only during the assessment of technical innovations in the trials, or environmental goals exist but are not quantified at the start of the study. In our work, satisfying the major constraints and the precise environmental targets were major aims, which became the conditions determining yield, with target yields set as high as possible under the conditions concerned. In addition, the clear definition of the constraint (i.e., reducing energy consumption or GHG emissions by 50%) and the environmental targets (i.e., having a value of at least 7 for all INDIGO environmental indicators) was also original. The quantitative levels of the constraints did not correspond to any regulations (i.e., these constraints reflected a major break with the regulations). However, these innovative cropping systems were considered as research tools which enabled identification of the most relevant agronomic practice combinations which could be used in more restrictive legislative contexts. The level quantifications of the constraints and environmental targets were very useful during the design process which required calculations.
Before the assessment of the prototypes in a long-term field trial, candidate systems were assessed and improved in an iterative process until the constraints were satisfied and environmental performance with respect to targets was optimized. This theoretical process of improvement has rarely been reported in previous studies. Cropping systems are usually assessed or compared in systems defined on the basis of the main standardized characteristics, essentially relating to one major aspect: e.g., organic versus conventional systemsReference De Ponti, Rijk and Van Ittersum 24 , Reference Coulter, Sheaffer, Wyse, Haar, Porter, Quiring and Klossner 63 , Reference Moreno, Lacasta, Meco and Moreno 64 , no-tillage versus conventional tillageReference Moreno, Lacasta, Meco and Moreno 64 , Reference Feiza, Feiziene, Auskalnis and Kadziene 65 or integrated versus conventional systemsReference Nowacki 61 , Reference Baturo 66 . Quantitative data for environmental criteriaReference Riley, Pommeresche, Eltun, Hansen and Korsaeth 26 , yieldReference Klimekova and Lehocka 67 or economic performanceReference Liebman, Gibson, Sundberg, Heggenstaller, Westerman, Chase, Hartzler, Menalled, Davis and Dixon 68 , Reference Cavigelli, Hima, Hanson, Teasdale, Conklin and Lu 69 were therefore recorded in experiments. The results of these comparisons can be used to compare the impact of different systems, but not to identify all solutions for their improvement. Even though inductive reasoning can bring about some conclusions with regard to general principles, another round of conception and evaluation is required, to strengthen cropping systems. Our prototyping approach is totally different. Innovative systems were assessed by modeling until they satisfied specific constraints and were optimized in terms of specific environmental targets. A field trial was then set up to determine whether each of the selected prototypes could satisfy its multiple constraints and targets. In this case, the various environmental targets were included in the agricultural strategies from the start of the design process, facilitating identification of the weaknesses of the system and making it possible to propose solutions for improvement before undertaking field trials. After the assessment of these innovative cropping systems in a field trial, their costs and economic performances will be calculated in different economic scenarios, to determine the likelihood of their being adopted by farmers.
Our approach required a large panel of experts (scientists, farmers and extension service staff) to design and to support prototypes throughout the design process (i.e., from the first to the last candidates). This was necessary because (1) the best crop management system may not correspond simply to the sum of individual agricultural practices, but may instead involve a set of agronomic strategies and their interactions; and (2) a breadth of agro-ecological knowledge is required to identify sets of agricultural rules likely to be responsive to such strict constraints and environmental targets. Moreover, this approach provided a more realistic view of cropping systems, making the adoption of the proposed innovations more likelyReference Le Gal, Dugué, Faure and Novak 70 . However, the field trial assessment step is still absolutely necessary because some innovative agronomic practices, not currently used in cropping systems, have never been evaluated by experts.
During the design process, about 15 experts attended individual sessions or group meetings, to provide knowledge unavailable from published work. The definition of crop rotations and agronomic practices took about 6 months, and a further 18 months were required for the writing of the decision rules. Published studies involving design processes have differed considerably in the number of experts involved and the time spent by individual experts, depending on the availability of the experts and the difficulties encountered in achieving the goals assigned to systemsReference Blazy, Ozier-Lafontaine, Doré, Thomas and Wery 62 , Reference Stoorvogel, Bouma and Orlich 71 .
Achievement of multiple constraints and targets
For all innovative systems, the constraints were satisfied with no consequences for other environmental components, except for the OMI of the No-Pest system. In this case, regular tillage combined with the restitution of only small amounts of organic matter had an adverse effect on soil environmental characteristics (indicator value for SOM of 5.7, according to the INDIGO® tool). Within this system, it did not appear to be possible to satisfy both the constraint and this environmental target with the available non-chemical techniques for pest control. Moreover, this was only possible with the available techniques by reducing yield targets with respect to those of current regional systems (Agreste 57 ). Nevertheless, progress in integrated pest management is being made, and new techniques may make it possible to improve environmental and yield performances. In the design of the L-EN system, we managed various agricultural processes, decreasing both direct energy consumption (e.g., due to tillage) and indirect energy consumption (due to the use of mineral fertilizers). We halved fossil energy consumption by greatly decreasing N fertilizer inputs, which was associated with a 20% yield loss. However, the energy performance of the L-EN system was expressed relative to that of the PHEP system, which also had a relatively low level of fossil fuel consumption with respect to current practices in Ile-de-France. The total energy consumption of the L-EN system was about 35% that of the current system in Ile-de-France (Agreste 57 ), when energy was expressed in MJ ha−1 (Table 4). For both the L-GHG and the PHEP systems, decreases in pesticide were taken into account by considering the maximum achievable yields to be similar to those of current low-input systems in Ile-de-France (Agreste 57 ), and much lower than those of conventional systems in the region (Agreste 57 ). Achievable yields for the L-GHG and the PHEP systems were considered to be 13 and 21% lower, respectively, than those of the current system in Ile-de-France. However, considering all the innovative systems together, it would appear to be possible to satisfy such ambitious constraints and environmental targets at the expense of only relatively small yield losses.
Available knowledge and current techniques suggested that it would not be possible to overcome all constraints in a single cropping system, because the agronomic practices used in one innovative system were incompatible with the constraints imposed on others. Plowing, one of the most effective practices against weeds used in the No-Pest system, is incompatible with large decrease in fossil energy consumption and the increase in C sequestration achieved with the L-EN and L-GHG systems, respectively. The large decrease in N fertilizer levels of the L-EN system is not compatible with the achievable yields defined for the PHEP and the L-GHG systems. Winter wheat sowing was delayed to avoid pest pressure in the No-Pest system, whereas it was brought forward in the direct drilling conditions of the L-EN and the L-GHG systems. This pattern was already evident during the design of the L-GHG system (i.e., GHG emission processes were managed in hierarchical order). Consequently, the development of a system without pesticides, with ambitious constraints in terms of GHG emissions and fossil fuel use, and with other environmental and yield targets, will require further progress in agronomic knowledge. For example, a better understanding of the interactions between cash and cover crops in terms of cooperative and competitive effects might allow the introduction of a living cover crop during cash crop growth in the L-GHG systemReference Shili-Touzi, de Tourdonnet, Nciri, Le Floch, Saulas and Doré 72 . The field trial assessment again proves essential to gain a better understanding of these interactions.
Improving the design process
In the design of the L-GHG system, we had to rank the secondary objectives (C sequestration had to be enhanced first, and then N2O emissions had to be reduced) to satisfy the GHG constraint. In this case, several practices had effects on both processes involved: no-tillage increased C sequestration in the soil but increased N2O emissions; ample N fertilizer applications were required to obtain high yields and, thus, abundant C residues, but this also generated more N2O emissions. We decided to promote C sequestration, because N2O emission assessments were highly uncertain due to the lack of published data about N2O emissions, for field bean residues for example (IPPC 51 ), and the variability of results due to differences in soil and climatic conditionsReference Rochette and Janzen 44 . Nevertheless, knowledge about the effects of cropping systems on N2O emissions is increasing, and it should be possible to improve the adjustment of cropping systems in the future.
The cropping systems assessment required tools and models adapted to the set of objectives and convenient to use during the iterative optimization process. Some approximations were used, due to the lack of data. In the L-GHG system, the coefficient defined by the Intergovernmental Panel on Climate Change (Tier 1) was used to calculate N2O release from the amount of N applied, rather than using different values for different soil and climatic conditionsReference Rochette and Janzen 44 . There were also uncertainties in the assessment of C sequestration. The two simulations provided similar ranks for the PHEP and the L-GHG systems, but the SIMEOS® tool and the Roth C model gave different quantitative results. One difference between these tools relates to tillage. In the SIMEOS® tool, mineralization mechanisms in the tilled layer are modified by tillageReference Andriulo, Mary and Guerif 48 . In the Roth C model, tillage is taken into account only indirectly, through the C inputs to the soil from crop biomass, which are affected by tillage practicesReference Liu, Chan, Conyers, Li and Poile 55 . Further uncertainties arise from the lack of root biomass data. Furthermore, these tools partially take into account changes in SOM content, which greatly influence N transformation mechanisms in the soil. Anyway, the difference in the values generated by these models suggested that neither may accurately predict actual performances of these contrasting cropping systems. If cropping systems assessment is to be considered relevant, it must make use of tools that are regularly updated, including: (1) ‘new’ machinery, such as harrows, hoes or direct-sowing drills with adjustable parameters in terms of energy consumption and GHG emissions; and (2) new more forms of pesticides or fertilizers. It may also be useful to improve systems assessments with crop models, but many parameters are unavailable for marginal crops (such as hemp or flax), for crop mixtures (e.g., cereal and legume combinations), or species mixtures used as catch crops (e.g., the association of spring oats, mustard and clover).
Several economic and social aspects were not taken into account during this design process, which focused on the cropping system rather than the farm scale. Nevertheless, we excluded some crops that are not grown by farmers in Ile-de-France due to the lack of a market (i.e., with low economic performances), but we included others for which the market is poorly developed in this area (e.g., lucerne and hemp). We did not take the organization of farm work into account either, although the identification and quantification of pest pressures in the field, to decrease pesticide use, is known to be time-consuming. From a technical point of view, we assumed that all farms owned the specific machinery used in the innovative systems, such as direct-sowing drills or mechanical weeding tools. For all these reasons, further investigations of the most relevant innovative cropping systems identified in a field trial assessment are needed, to forecast their possible application in an area.
Toward field assessment of the innovative cropping system prototypes
The innovative systems prototypes designed here were based on different hypotheses about soil and climate effects, because the true impact on crops and soil are unknown. For example, we assumed that agricultural practices and climatic conditions would not affect crop emergence. In our conditions, crops are not affected by direct drilling because this technique is widely used in France (http://agriculture-de-conservation.com/). However, conservation tillage and mulch tillage practices remain largely empiricReference Alletto, Coquet and Roger-Estrade 73 , and additional knowledge is required for the optimization of these techniques, to make it possible to achieve good results in terms of sowing management and target yields. We also assumed that rainfall would provide enough water throughout the year in northern France to allow catch crop and main crop emergence, but this may no longer be the case if the climate changes radically. We assumed that new equilibria would appear with certain practices, allowing inputs to be reduced. In the L-GHG system, the practice of leaving crop residues on the soil should decrease weed emergence, making it possible to decrease the amount of herbicide used, and the absence of plowing should lead to the maintenance or increase in size of ground beetle populations, making molluscicide use unnecessary. The achievable yield was determined on the basis of several assumptions. In the L-GHG system, we promoted C sequestration, which is highly dependent on cereal yields and difficult to estimate in the case of no-tillage systems, because the presence of excessive amounts of crop residues may decrease emergence. The chemical properties of the soil associated with different tillage practices are also poorly characterizedReference Alletto, Coquet and Roger-Estrade 73 . In this system, the effects on water availability of sowing cover crops every year also should be analyzed. In the L-EN system, the mixture of winter wheat and white clover might also decrease cereal yields. In the No-Pest system, yields were defined by approximation to organic systems, in which no mineral fertilizers are permitted. In this system, late winter cereal sowing might lead to higher levels of damping-off and plant death during winter, decreasing yields to a greater extent than anticipated. These are just a few of the uncertainties remaining about the real performance of the systems we have devised. For these reasons, the field assessment of these system prototypes, which is currently being carried out (the experiment started in 2008), is absolutely necessary.
Finally, it should be stressed that approaches of this type could be used in many agronomic situations, with a diversity of challenges, provided that sufficient knowledge is available for the development of innovative strategies. In our opinion, this approach meets the need expressed by Foley et al.Reference Foley, Ramankutty, Brauman, Cassidy, Gerber, Johnston, Mueller, O'Connell, Ray, West, Balzer, Bennett, Carpenter, Hill, Monfreda, Polasky, Rockström, Sheehan and Siebert 14 to ‘search for practical solutions’ for a cultivated planet.
Acknowledgements
We thank the experts who took part in the design process, Marie-Hélène Jeuffroy, Jane Jonhson, Laurence Guichard, Marie Gosme and David Makowski for their useful comments on a previous version of the paper, Suzette Tanis-Plant for linguistic help and Alain Bone for assistance with bibliographic research.