Dietary tryptophan and the pathways to serotonin function
Serotonin, or 5-hydroxytryptamine (5-HT), is a monoamine neurotransmitter in the central nervous systems (CNS) of the majority of animals, including human beings. Its synthesis depends on the supply of the essential amino acid, l-tryptophan (TRP), which cannot be biosynthesised by human beings and so must be obtained from dietary sources. Moreover, serotonin synthesis rate depends on the availability of the precursor TRP. The scope of this review is to consider recent findings from research involving effects of supplementing TRP supply on behaviour and their interaction with genetic susceptibility, including indirect evidence that TRP supplementation likely alters affective states via effects on central serotonin function.
An important consideration for understanding effects of TRP administration is that only about 5 % of endogenous serotonin is found in the brain; the remainder is in the gut (about 90 %), principally released by enterochromaffin cells, and in peripheral tissue or in the blood, where it is taken up into blood platelets(Reference Fernstrom1–Reference Young and Teff3). Indeed, the name serotonin derives from its discovery in blood 70 years ago and the observation that it caused contraction of vascular smooth muscle(Reference Watts, Morrison and Davis4); thus, one function of serotonin is to regulate local blood flow. This imbalanced distribution between brain and periphery needs to be borne in mind when considering the possible impact of dietary manipulation of central serotonin by TRP, and the potential influence of alternative metabolic pathways as well as probable moderating effects on these metabolic routes. These issues are considered further later; nevertheless, serotonin is a widely distributed and important CNS neurotransmitter, arising from neuronal cell bodies located in the higher and lower raphe nuclei of the brainstem, and acting at multiple receptor subtypes with a range of behavioural effects(Reference Jacobs, Martin-Cora and Fornal5). Serotonin's established importance in affective disorders and appetite, as well as sleep and cognition(Reference Fernstrom, Langham and Marcelino6), make understanding who might benefit most from therapeutic use of TRP an important goal of research.
Metabolic pathways for l-tryptophan
As with other essential amino acids, TRP can contribute to hepatic biosynthesis of proteins; however, TRP is typically incorporated into proteins at only 1–2 % of total amino acids, making it the scarcest of amino acids in dietary proteins(Reference Young and Teff3, Reference Fernstrom and Fernstrom7). Nevertheless, if TRP is acutely deficient, incorporation into protein synthesis will contribute to a substantial lowering of plasma TRP levels(Reference Moja, Restani and Corsini8, Reference van Donkelaar, Blokland and Ferrington9). However, in the absence of TRP deficiency, the majority of consumed TRP is metabolised via other pathways, including for synthesis of 5-HT, melatonin and niacin (vitamin B3). Indeed, it has been estimated that only 1 % of dietary TRP is used for brain 5-HT synthesis(Reference Richard, Dawes and Mathias10). TRP use for synthesis of niacin is via the oxidative kynurenine pathway, which has also been termed the TRYCAT pathway(Reference Maes, Leonard and Myint11). This pathway is becoming increasingly recognised as having important implications for health, including neuropsychiatric conditions such as depression(Reference Maes, Leonard and Myint11, Reference Anderson, Jacob and Bellivier12). A further route for TRP metabolism is via degradation by gut microbiota, which can lead to production of both positive and detrimental active metabolites, including quinolinic acid(Reference Fernstrom1); therefore, individual variation in the gut microbiome may have implications for TRP metabolism and thus brain health and psychological wellbeing(Reference Dinan and Cryan13).
The kynurenine, or TRYCAT, pathway involves an initial rate-limiting metabolism of TRP to kynurenine catalysed by the hepatic enzyme, tryptophan 2,3-dioxygenase (TDO), which can be induced by glucocorticoid hormones(Reference Badawy14). However, under inflammatory conditions, the extrahepatic enzyme, indole 2,3-dioxygenase becomes increasingly important in metabolising TRP to kynurenine, due to induction by pro-inflammatory cytokines(Reference Maes, Leonard and Myint11). These inductive influences on diversion of TRP metabolism away from 5-HT synthesis have been proposed as mechanisms underlying the link between stress, inflammation, deficient 5-HT function and depression(Reference Maes, Leonard and Myint11, Reference Anderson, Jacob and Bellivier12).
The metabolism of TRP for the synthesis of 5-HT is catalysed by the rate-limiting enzyme, TRP hydroxylase (TPH), which converts TRP into 5-hydroxytryptophan. In turn, 5-hydroxytryptophan is decarboxylated to 5-HT by the enzyme aromatic amino acid decarboxylase. The key observation for this pathway is that TPH is not fully saturated by its substrate TRP under normal conditions, so that raising brain TRP levels could increase serotonin synthesis. However, brain TRP levels are buffered from plasma TRP by the blood–brain barrier: to be transported into the brain, TRP has to compete for uptake across the blood–brain barrier against other amino acids, in particular a group known as the large neutral amino acids (LNAA), especially the branched chain amino acids, leucine, isoleucine and valine, but also phenylalanine and tyrosine (the precursors for catecholamine, dopamine, adrenaline, noradrenaline, transmitter synthesis). Thus, the ratio of plasma or serum TRP to LNAA (TRP:LNAA) is recognised as the best peripheral biomarker of uptake of TRP into the brain(Reference Fernstrom and Fernstrom7). Some 90 % of TRP in blood is typically bound to the blood protein albumin, and it is often assumed that the remaining free unbound fraction of TRP should be taken to be the best predictor of TRP entry across the blood–brain barrier. However, it has been shown that TRP binding to albumin is very labile, such that TRP can easily be released in cerebral circulation. Furthermore, TRP can be displaced from or prevented from binding to albumin by NEFA, which also bind readily to albumin(Reference Fernstrom and Fernstrom7, Reference van Donkelaar, Blokland and Ferrington9). Therefore, factors that alter NEFA levels in blood will affect the availability of free TRP for entry into the brain: for example, sympathetic activation by stress or exercise will induce lipolysis, increase plasma NEFA and so release more TRP from albumin. This acute stress-induced increase in availability of TRP for serotonin synthesis might contribute to the observation that even mild stress can increase 5-HT release in rat brain(Reference Gibson, Barnfield and Curzon15). It also suggests that caution is required in interpreting correlations between single measures of plasma-free TRP and personality traits such as anxiety or aggression, as these may interact with the experimental procedure and perceived stressful nature of the study to modify TRP levels. In contrast, food or drink that stimulates insulin release, and so promotes uptake of NEFA into tissue, will tend to reduce the availability of free plasma TRP, but at the same time will remove competing LNAA from plasma into tissue(Reference Fernstrom and Fernstrom7). Thus, measuring both free and total TRP may ensure better prediction of TRP entry into the brain and its behavioural associations(Reference van Donkelaar, Blokland and Ferrington9, Reference Pardridge and Fierer16).
However, 95 % of 5-HT is synthesised and used in the gut, blood and peripheral tissue(Reference Fernstrom1, Reference Badawy14). Although the synthesis of 5-HT from TRP follows a similar biochemical path in brain and periphery, the form of the enzyme TPH, by and large, differs slightly between these regions; these isoforms are known as TPH1 and TPH2 respectively, indicating the order of characterisation(Reference Fukuda17, Reference Walther and Bader18). To be precise, in the brain the principal isoform, TPH2, shown to depend on expression of a different gene form from TPH1(Reference Walther and Bader18), was found to be highly expressed by measuring mRNA specific to the brainstem raphe nuclei, where brain serotonin is primarily synthesised, whereas TPH1 was found to be responsible for 5-HT synthesis, and ultimately melatonin, in the pineal gland(Reference Patel, Pontrello and Burke19) and gut(Reference Walther and Bader18). However, this classification is oversimplified, as TPH1 mRNA has also been shown to be more highly expressed in the amygdala and hypothalamus than TPH2(Reference Zill, Büttner and Eisenmenger20), although its precise role in those sites is uncertain.
Serotonin and behaviour
Serotonin has long been associated with several fundamental aspects of behaviour, including sleep, appetite, cognition, and social and emotional behaviours such as anxiety, depression, empathy and aggression(Reference Gyurak, Haase and Sze21, Reference Steenbergen, Jongkees and Sellaro22). These influences of serotonin on behaviour will be briefly reviewed prior to consideration of the impact of TRP supplementation and its interaction with 5-HT-related genotypes.
Early neurophysiological and lesion work on the function of CNS 5-HT demonstrated a clear role in regulating sleep(Reference Jouvet23), whereas the therapeutic use of monoamine oxidase (MAO) inhibitors (which prevent serotonin, and other monoamine, metabolism by the enzyme MAO), as well as development of tricyclic antidepressants (which inhibit synaptic reuptake of monoamine neurotransmitters), such as imipramine, to treat depression, led to the ‘serotonin hypothesis’ of depression, in which depression is seen primarily to result from a deficit in 5-HT function(Reference Coppen, Shaw and Herzberg24, Reference Cowen and Browning25). The theory expanded to consider a role for CNS 5-HT in associated clinical affective disorders as well as regulation of mood in healthy people(Reference Cowen and Browning25). However, this pharmacotherapeutic evidence was non-specific to serotonin, and ironically, notwithstanding the risk of oversimplifying neural bases to complex disorders, the best evidence for a major role for CNS 5-HT in control of affect has come from studies that manipulate TRP entry to the brain(Reference Fernstrom26). Furthermore, whilst recent studies combining neuroimaging with administration of selective serotonin reuptake inhibitors have also strengthened the evidence for a role for central 5-HT in depression(Reference Godlewska, Norbury and Selvaraj27), other evidence is emerging for the importance of peripheral metabolic pathways for TRP, including roles in inflammatory processes and melatonin synthesis, underlying major depression, seasonal affective disorder and bipolar disorder(Reference Fernstrom1, Reference Maes, Leonard and Myint11, Reference Anderson, Jacob and Bellivier12, Reference Pereira, Pradella Hallinan and Alves28).
Central serotonin is known to be involved in cognitive function, especially memory, attention, decision making and information processing, as well as in the processing of emotionally relevant stimuli(Reference Fernstrom26, Reference Silber and Schmitt29, Reference Young30). However, cognition and emotion, or affect, are not entirely separable, and are often strongly interdependent(Reference Duncan and Barrett31–Reference Young33). Emotions, via their neural substrates, influence memory and attention for example, and depression and anxiety are associated with cognitive impairments and biases that can contribute to the affective disorder and its maintenance(Reference Rolls32, Reference Mathews and MacLeod34).
Effects of acute tryptophan depletion
This review is mainly concerned with genetic susceptibility to effects of forms of TRP administration that may lead to increased serotonin synthesis in the brain; however, by way of comparison, and given the scientific influence, a brief overview is included of findings, and their implications, on deficits in central 5-HT induced by acute TRP depletion (ATD) methods(Reference Young33, Reference Young, Smith and Pihl35). ATD is usually induced by ingestion of amino acid loads devoid of the precursor amino acid TRP to suppress 5-HT synthesis, and can be preceded by a low-TRP diet for a few days(Reference Silber and Schmitt29). This results in a substantial (e.g. >70 %) and rapid drop in plasma TRP, and TRP:LNAA ratio (>80 %) that may last 4–6 h(Reference Young30, Reference Young, Smith and Pihl35); similar effects have been found with a more palatable low-TRP collagen protein mixture(Reference Evers, Tillie and van der Veen36), and more recently a gelatin-derived TRP-free protein/carbohydrate mixture has been used(Reference van Donkelaar, Blokland and Ferrington9). Moreover, the serotonin metabolite, 5-hydroxyindole acetic acid measured in cerebrospinal fluid (CSF) declined by about one-third at 12 h, after which measurements stopped(Reference Williams, Shoaf and Hommer37). ATD methods have provided the most consistent evidence for serotonergic involvement in cognition, including impairment of memory consolidation(Reference McAllister-Williams, Massey and Rugg38, Reference Riedel, Klaassen and Deutz39) and aspects of cognitive flexibility including learning(Reference Park, Coull and McShane40) and decision-making(Reference Rogers, Tunbridge and Bhagwagar41). Moreover, evidence in animal models is persuasive of opposing effects of both ATD and TRP supplementation on brain 5-HT(Reference Fernstrom, Langham and Marcelino6, Reference Crockett, Clark and Roiser42–Reference Hulsken, Martin and Mohajeri44).
In support of a key role for serotonin in affective disorders, ATD also alters emotional processing and regulation(Reference Cools, Calder and Lawrence45–Reference van der Veen, Evers and Deutz47). Reducing TRP access to the brain by ATD tends to mimic the cognitive biases seen in depressed populations, such as impaired memory for, attention to, or recognition of positive v. negative information including facial expressions(Reference Mathews and MacLeod34, Reference Harmer, Rogers and Tunbridge48, Reference Robinson and Sahakian49). However, positive effects of ATD on cognition, for example on decision making and focused attention have also been reported(Reference Riedel, Sobczak and Schmitt50–Reference Booij, Van der Does and Haffmans52), albeit interacting with a history of depression(Reference Sobczak, Honig and Nicolson53). One explanation has been that serotonin may affect ‘hot’ cognitive tasks that include an affective component, but not ‘cold’ cognitive tasks that do not obviously involve emotional stimuli(Reference Robinson and Sahakian49).
Neuroimaging techniques show that activity of brain regions involved in emotion regulation such as the limbic system and prefrontal cortex is sensitive to ATD(Reference Biskup, Gaber and Helmbold46). The evidence is consistent with a normally inhibitory role of serotonin on any tendency for negative emotional bias(Reference Allen, McKnight and Moreno54, Reference Robinson, Overstreet and Allen55). Importantly, family or personal history of depression, sex and at-risk genotypes, have been reported to moderate effects of ATD on brain activity to emotional stimuli(Reference Biskup, Gaber and Helmbold46, Reference van der Veen, Evers and Deutz47, Reference Roiser, Levy and Fromm56).
Despite a history of use of anorexigenic drugs with serotonergic agonist activity such a d-fenfluramine(Reference Curzon, Gibson and Oluyomi57), and reductions in food intake established for high doses of TRP(Reference Hill, Blundell and Huether58), and thus an expectation that ATD might increase appetite, the few studies addressing this directly in human beings suggest little effect of ATD on appetite despite concurrent mood effects(Reference Reilly, McTavish and Young59–Reference Oldman, Walsh and Salkovskis61). Two studies comparing ATD in women with bulimia nervosa v. healthy controls found conflicting results(Reference Kaye, Gendall and Fernstrom60, Reference Weltzin, Fernstrom and Fernstrom62): although both studies found increased negative affect in bulimic women, only one reported increased energy intake in these women(Reference Weltzin, Fernstrom and Fernstrom62), although the other did find an increased desire to binge eat(Reference Kaye, Gendall and Fernstrom60). However, curiously, another study reported a concurrent increase in both nausea and hunger in healthy women(Reference Rieber, Mischler and Schumacher63). These findings also need to be considered in the context of opposing relationships between depression and appetite across patients(Reference Simmons, Burrows and Avery64).
Two other behaviours that appear to be sensitive to serotonin depletion are aggression and impulsivity(Reference Young33, Reference Virkkunen, Goldman and Nielsen65). ATD has resulted in increased aggressive behaviour in the majority of studies where measured(Reference Young33), and aggressive traits have correlated with plasma levels of TRP and CSF indices of serotonin turnover(Reference Virkkunen, Goldman and Nielsen65). However, gene by environment interactions, including stressful life events, and sex differences, are likely to moderate findings(Reference Glick66, Reference Perry, Goldstein-Piekarski and Williams67), and a meta-analysis of associations between 5-HT function and aggression in human beings revealed only a weak negative relationship(Reference Duke, Begue and Bell68). It may be that stronger associations will be found when genetic variants influencing serotonin function, such as in enzymes involved in synthesis and metabolism, or polymorphisms in transporter systems (see later), are taken into account(Reference Antypa, Serretti and Rujescu69, Reference Laas, Kiive and Maestu70). Indeed, a key criticism put forward is the observation that ATD lowers TRP quite universally across participants, and yet the behavioural effects differ considerably depending on a propensity to dysfunction of mood or emotional regulation, or poor stress coping(Reference van Donkelaar, Blokland and Ferrington9).
Effects of l-tryptophan administration and supplementation
In contrast to ATD, which is a research tool to investigate serotoninergic processes in human beings, and for which most effects are not beneficial, administration of TRP (and its first-stage metabolite, 5-hydroxytryptophan) has a long history of being studied for potential clinical benefit in depression, as well as for basic research, as a means to facilitate entry of TRP into the brain and thus elevate 5-HT synthesis and release(Reference Fernstrom26, Reference Young33). The methods can vary from intravenous administration of TRP to oral supplementation of TRP, or use of TRP-rich proteins or peptide preparations, either acutely or chronically(Reference Fernstrom26, Reference Silber and Schmitt29, Reference Schmitt, Wingen and Ramaekers71). It is also possible to increase the TRP:LNAA ratio, and so enhance TRP entry across the blood–brain barrier, by feeding a carbohydrate-rich, very low-protein meal, since the rise in insulin removes more LNAA into surrounding tissue. This dietary method has been shown to benefit cognitive and emotional function, and reduce the cortisol response to stress, in more stress-prone, neurotic participants(Reference Markus, Panhuysen and Tuiten72–Reference Markus, Panhuysen and Jonkman75). This mechanism has also been suggested to underlie dietary effects on mood and performance, such as calming after high-carbohydrate meals v. arousal after protein-rich meals(Reference Gibson and Green76, Reference Hoyland, Lawton and Dye77). Recently, using data from the US National Health and Nutrition Examination Survey for nearly 30 000 adults, dietary intake of TRP was found to be inversely associated with self-reported levels of depression, and positively related to sleep duration (more strongly in women; adjusted for protein intake)(Reference Lieberman, Agarwal and Fulgoni78). Thus, even in complex whole diets, TRP intake appears to provide psychological benefits.
TRP supplementation has been employed as a potential treatment for depression and sleep disturbance since the early 1960s(Reference Coppen, Shaw and Herzberg24, Reference Thomson, Rankin and Ashcroft79), although a Cochrane Review of 108 trials (including for 5-hydroxytryptophan) for antidepressant effects in 2002 found that only two trials were of sufficient quality to be included(Reference Shaw, Turner and Del Mar80). Nevertheless, on that limited evidence, TRP was considered to be better than placebo in alleviating depression, at least in mild to moderately depressed people. Moreover, for more than a decade prior to that review, the US Food and Drug Administration had banned over-the-counter sales of TRP following an outbreak in 1989 of the harmful eosinophilia–myalgia syndrome in users of TRP supplements. The cause was eventually traced to impurities in TRP supplements from one Japanese manufacturer, after which the ban was lifted in 2001(Reference Richard, Dawes and Mathias10, Reference Fernstrom26). Thus, for at least five decades, TRP has been used pharmacologically, i.e. at daily doses sometimes well in excess of ten times the RDA (5 mg/kg) for this essential amino acid. There was early evidence for probable enhancement of brain 5-HT function: after 50 mg/kg TRP (3·5 g per 70 kg subject) was consumed in a milk drink, plasma TRP increased 8-fold, TRP in CSF increased 6-fold after 6–8 h, and the metabolite 5-hydroxyindole acetic acid increased almost 2-fold in CSF by 8 h, suggesting increased turnover of brain 5-HT(Reference Eccleston, Ashcroft and Crawford81). This 2-fold increase in 5-HT turnover was replicated in a later study of CSF 5-hydroxyindole acetic acid changes, using 3 and 6 g TRP, with no further increase at the higher dose, although the level was sustained for longer, i.e. 12 v. 8 h(Reference Young and Gauthier82).
In a review of potential side-effects, Fernstrom(Reference Fernstrom26) concluded that such use of TRP appears to be largely safe from adverse events, although the evidence is limited and not systematic. There are some reports of symptoms such as nausea, tremor or dizziness when high doses are used (although these are also common symptoms reported in placebo-treated subjects). However, the greatest risk of side-effects occurs when TRP is combined with other drugs that enhance 5-HT availability, such as antidepressant selective serotonin reuptake inhibitors or MAO inhibitors: then a toxic ‘serotonin syndrome’ may occur that can include hyperthermia and coma(Reference Fernstrom26). A more common effect of high doses of TRP is fatigue or drowsiness, which has led to TRP being used to aid sleep, in which case sedation is not an unwanted side-effect(Reference Fernstrom26). However, a complication of oral TRP at higher doses is that it increases the release of several hormones, including growth hormone, cortisol and prolactin(Reference Porter, Gallagher and Watson83) (the latter thought to indicate increased central serotonin, and dopamine, activity). A recent study also reported that intragastric administration of 1·56 g TRP increased plasma cholecystokinin and glucagon-like peptide 1, as well as slowing gastric emptying(Reference Meyer-Gerspach, Hafliger and Meili84): although subjective appetite was not affected, it is likely that these mechanisms contribute to reduced food intake reported after higher doses of TRP(Reference Hill, Blundell and Huether58). Even so, food intake might be reduced merely due to TRP-induced drowsiness.
There is also concern that excess metabolism through pathways such as TRYCAT could lead to high levels of neuronally active metabolites such as kynurenic acid and quinolinic acid. However, a recent review did not find evidence for adverse side-effects via these routes, although it was acknowledged that more systematic research is needed(Reference Fernstrom1). Furthermore, it has been argued that the modest antidepressant effect of TRP loading is due to accelerated hepatic degradation of TRP in depressives, probably via stress-related neuroendocrine enhancement of the catabolic hepatic enzyme TDO(Reference Badawy85).
As would be expected in a treatment with antidepressant potential, there is considerable evidence for beneficial effects of TRP on mood and social behaviour, and these findings have recently been reviewed(Reference Steenbergen, Jongkees and Sellaro22, Reference Young33). There is some evidence that TRP can reduce aggression in schizophrenic patients(Reference Young33), and reduce quarrelsomeness while increasing agreeableness in healthy participants with a tendency to irritability or aggression(Reference Steenbergen, Jongkees and Sellaro22). Thus, it has been proposed that serotonin may influence a basic drive to be social, and that modulation of serotonin can alter more complex social behaviours by affecting social behaviour along an agreeable-quarrelsome axis(Reference Young33). For example, there is evidence that TRP supplementation can promote prosocial behaviour in economic decision-making tasks(Reference Steenbergen, Jongkees and Sellaro22). Somewhat counterintuitively, a more recent study, in which 1 g TRP was given three times daily for 14 d to those with a family history of depression, found increased quarrelsomeness and reduced agreeableness (at home), but improved mood, compared with placebo(Reference Hogenelst, Schoevers and Aan Het Rot86). This was interpreted as possibly reflecting the development of more control in social interactions at home.
Effects of l-tryptophan-rich protein preparations
Bearing in mind such concerns about loading with high doses of TRP as the single amino acid, in recent years methods have been developed to enhance TRP availability to the brain by administering TRP-rich dietary proteins: the most published example is the whey protein α-lactalbumin. The effects of this protein are usually compared with responses after ingestion of another protein, typically casein hydrolysate (another milk protein), which has lower levels of TRP but greater amounts of the competing LNAA(Reference Silber and Schmitt29).
Similarly to a high-carbohydrate meal, α-lactalbumin has been shown to enhance (or correct) serotonin function (indexed by prolactin release) and cognition, and to reduce cortisol release, in stress-prone (more anxious) participants(Reference Markus, Olivier and de Haan87, Reference Markus, Olivier and Panhuysen88). α-lactalbumin attenuated deficits in delayed memory in women suffering from premenstrual syndrome(Reference Schmitt, Jorissen and Dye89) and in recovered depressives and healthy subjects(Reference Booij, Merens and Markus90). This TRP-rich protein also improved the perception of emotional faces within women(Reference Attenburrow, Williams and Odontiadis91); however, effects on emotional face processing tend to be weaker than dosing with TRP alone(Reference Scrutton, Carbonnier and Cowen92).
Another TRP-rich protein that has been used for research in this area is a proprietary peptide product, which is an egg-white protein hydrolysate formulation that contains fewer competing LNAA (DSM Nutritional Products Ltd., Basel). This peptide, taken in drink form, has been shown to be more effective in raising plasma TRP:LNAA ratios than either α-lactalbumin or TRP alone(Reference Markus, Firk and Gerhardt93, Reference Mitchell, Slettenaar and Quadt94). Preliminary studies using a 12-g dose (0·66 g TRP) of this TRP-rich protein hydrolysate showed improved mood in all subjects and enhanced psychomotor and vigilance performance in individuals more resilient to stress(Reference Markus, Firk and Gerhardt93, Reference Markus, Verschoor and Firk95). This was supported by a functional MRI study in young women(Reference Kroes, van Wingen and Wittwer96) which found that this dose improved mood acutely as well as increasing activation of brain limbic circuitry, especially medial cingulate gyrus, during a fear induction task. Conversely, during reward anticipation, activation of reward pathways was reduced. Effects on resting state connectivity were in line with modulation of brain regions involved in regulation of mood. Subsequently, lower doses were found to be effective in enhancing mood and positivity in emotional processing acutely (0·13 g TRP)(Reference Gibson, Vargas and Hogan97), and chronically (0·07 g TRP for 19 d) in improving aspects of mood and sleep, as well as modest benefits to cognition, in middle-aged women, relative to a casein control treatment(Reference Mohajeri, Wittwer and Vargas98).
Role of genetics in moderating effects of l-tryptophan supplementation or challenge on serotonin-related behaviours
Gene polymorphisms involved in the metabolism of TRP and regulation of serotonin could have a substantial influence on behavioural effects of manipulations of TRP availability. There is potential for moderation of TRP effects by polymorphisms in each of the key enzymes influencing TRP metabolism and thus serotonin synthesis, i.e. TPH1, TPH2, TDO, indole 2,3-dioxygenase and also by polymorphisms of the MAO-A enzyme that metabolises central serotonin (Fig. 1). These various 5-HT-related polymorphisms may form an interactive system that determines the aetiology and prognosis of various forms of affective disorder(Reference Fukuda17, Reference Oxenkrug99–Reference Popova and Kulikov102). However, the most evidenced serotonergic genetic influence on behaviour is the 5-hydroxytryptamine transporter-linked promoter region (5-HTTLPR) polymorphism of the serotonin transporter gene (SLC6A4)(Reference Shinozaki103–Reference Pergamin-Hight, Bakermans-Kranenburg and van Ijzendoorn104). The recommended classification of 5-HTTLPR genotypes is a functional combination of variable number tandem repeats of short or long length of the gene promoter amplicon and SNP variants, LA and LG, where LG is functionally equivalent to the short, and LA to the long, variable number tandem repeats forms(Reference Shinozaki103–Reference Pergamin-Hight, Bakermans-Kranenburg and van Ijzendoorn104). Effects of 5-HTTLPR genotypes are usually compared between the homozygous alleles that are either high (long variants; L/L′) or low (short variants but including LA; S/S′) expressing of mRNA for the 5-HT transporter receptor.

Fig. 1. This figure illustrates metabolic and other biochemical pathways in gut and blood that moderate the ability of supplementary l-tryptophan (TRP) to enter the brain as the precursor for the synthesis of brain serotonin (5-hydroxytryptamine (5-HT)), and thus to alter behaviour, especially mood, cognition and appetite. Rounded rectangles indicate enzymes involved in the various pathways. Thus, indole 2,3-dioxygenase (IDO) and tryptophan 2,3-dioxygenase (TDO) are involved in the catabolism of TRP via the ‘tryptophan catabolite’ (TRYCAT) pathway, resulting in kynurenine (KYN) and then niacin formation. This could alter the TRP-to-large neutral amino acids (TRP/LNAA) ratio and thus TRP entry into the brain, where the enzyme tryptophan hydroxylase (TPH; present as either TPH1 or TPH2) is the rate-limiting step for conversion of TRP to 5-HT in serotonergic neurones. Action of 5-HT at the synapse can, in turn, be modified by the enzyme monoamine oxidase-A (MAO-A), and by the 5-HT transporter system that has functional genetic variants in the 5-HT transporter-linked promoter region (5-HTTLPR). Abbreviations in bold represent influences that have known functional genetic variants which may vary in their moderating effects; these, in turn, can interact with sex.
Another important genetic factor in predicting serotonergic effects on behaviour is sex. Women are more susceptible to, and have higher heritability for, affective disorders (even allowing for sociocultural effects on presentation), may be more sensitive to stress, and tend to be more responsive to serotonin selective reuptake inhibitors treatment(Reference Perry, Goldstein-Piekarski and Williams67). Brain 5-HT synthesis rates are reportedly 50 % lower in women than men(Reference Nishizawa, Benkelfat and Young105), and ATD causes greater lowering of mood in women than men(Reference Booij, Van der Does and Benkelfat106). In some studies, women also appear to be more sensitive to, or to benefit more from, TRP supplementation; indeed, some researchers chose to study women only for these reasons(Reference Gibson, Vargas and Hogan97, Reference Mohajeri, Wittwer and Vargas98). Furthermore, sex interacts with serotonergic gene polymorphisms in several systems, including 5-HTTLPR, TPH1, TPH2 and MAO-A(Reference Perry, Goldstein-Piekarski and Williams67, Reference Gressier, Calati and Serretti107), and these interactions can be further moderated by stress(Reference Brummett, Muller and Collins108–Reference Sjoberg, Nilsson and Nordquist111). Therefore, the sex of participants needs to be considered when interpreting findings in this area.
Tryptophan administration and 5-hydroxytryptamine transporter-linked promoter region genotypes
Only a few studies have investigated whether these 5-HT- and TRP-related genotypes alter the effects of TRP loading (or challenge or supplementation), and these appear to be limited to a comparison of 5-HTTLPR genotypes: these studies are summarised in Table 1. In the earliest published study(Reference Brummett, Muller and Collins108) to examine moderation of TRP loading by the 5-HTTLPR tri-allelic genotype, forty-one men and thirty-one women were infused intravenously with a high dose of TRP (100 mg/kg), while aspects of mood were assessed (Profile of Mood States). Far from improving mood, this procedure generally increased negative affect, but the effects were moderated by genotype and sex: in men, only those with the high-expressing L/L′ polymorphism showed increased negative mood, whereas in women, only the L/L′ group showed no increase in negative mood. This opposing interaction between sex and 5-HTTLPR genotype is in line with evidence based on the impact of social stressors on negative affect in adolescents(Reference Sjoberg, Nilsson and Nordquist111). However, sample sizes were small, especially in the S/S′ groups (seven men; nine women).
Table 1. Summary of studies investigating interactions between l-tryptophan (TRP) supplementation or challenge and tri-allelic 5-HT transporter-linked promoter region (5-HTTLPR) genotypes on behaviour
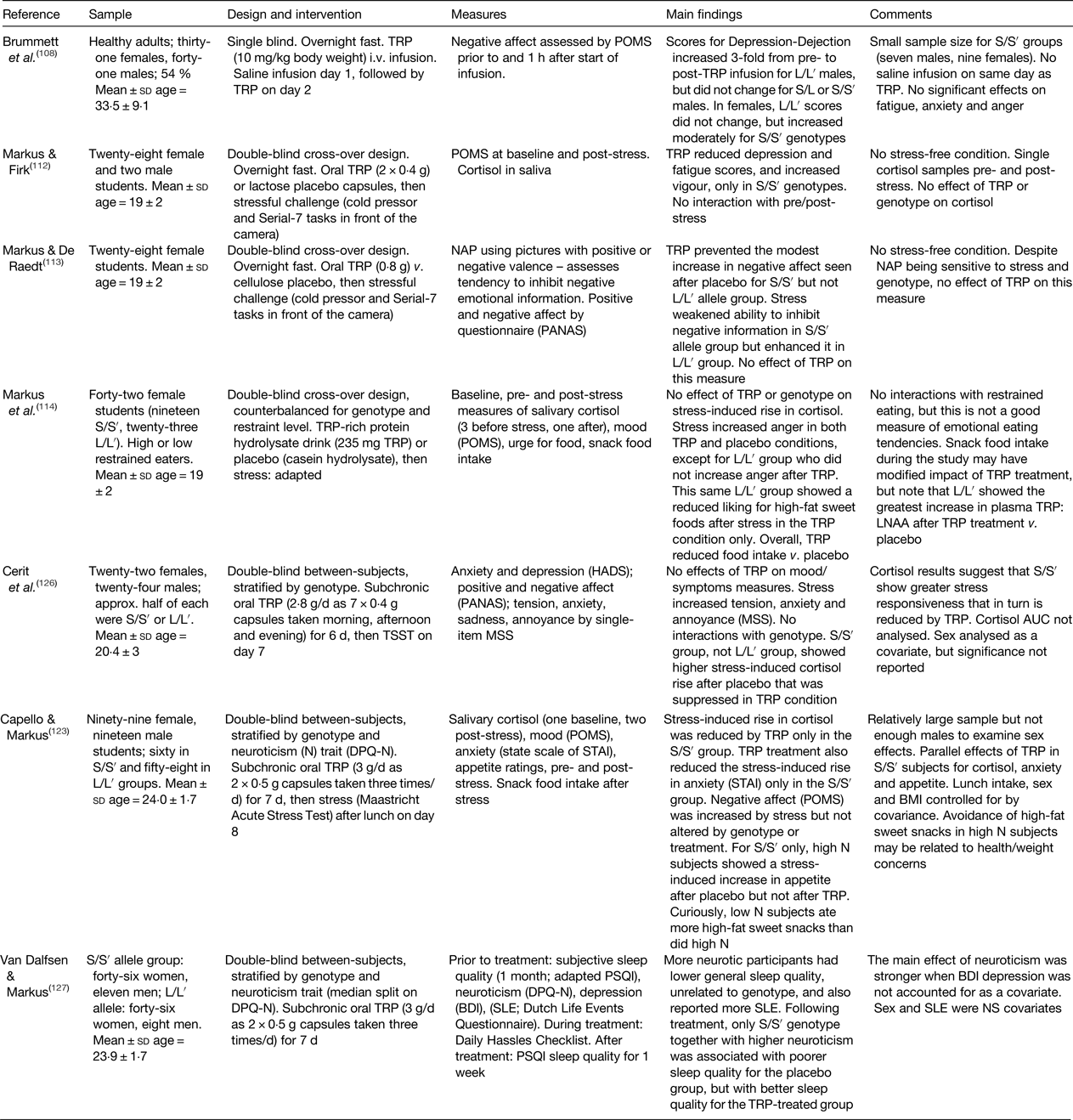
POMS, Profile of Mood States; NAP, Negative affect priming; PANAS, Positive and Negative Affect Schedule; TSST, Trier Social Stress Test; HADS, Hospital Anxiety and Depression Scale; MSS, Mood States Scale; DPQ-N, Dutch Personality Inventory; STAI, State and Train Anxiety Inventory; PSQI, Pittsburg Sleep Quality Index; BDI, Beck Depression Inventory; SLE, Stressful Life Events.
Using a far lower dose, and oral administration, Markus and Firk(Reference Markus and Firk112) examined potential interactions between acute TRP supplementation, stress and 5-HTTLPR genotype on mood, cortisol and cognition. They hypothesised that the TRP challenge would ameliorate the effects of stress on mood and cortisol in subjects homozygous for the tri-allelic S/S′ genotype compared to those with the L/L′ genotype. In a cross-over design, thirty student participants (sixteen S/S′-allele; fourteen L/L′-allele; only one man in each group) received either TRP (2 × 0·4 g) or placebo (lactose), prior to a stressful challenge, with baseline and post-stress measures of mood (Profile of Mood States) and salivary cortisol. The stressor consisted of repeated unpredictable cold pressor stress (hand on a 1·5°C cold plate) interspersed with a Serial-7 subtraction task (repeatedly subtracting 7 from a variable starting number), performed in front of a camera and researcher; errors were recorded. The design did not include a stress-free condition, and only a single baseline measure of cortisol, so interpretation of the observed decline in cortisol after the stress is difficult, as this decline is anyway typical for cortisol during the morning. However, neither TRP treatment nor genotype significantly altered this decline in cortisol. Nevertheless, the stressor caused mood to deteriorate, with increases in feelings of anger, depression and fatigue, but a decrease in vigour. A key finding of this study is that the TRP treatment reduced depression and fatigue, while increasing vigour, specifically in the S/S′ allele group only. However, these effects were pooled across stress condition, so presumably were not significantly altered by stress (data for a pre/post-stress × genotype × treatment interaction were not presented). Genotype also influenced performance on the subtraction task: the S/S′ group performed worse than the L/L′ group after placebo, but after TRP, performance was the same for both allele groups; again, this result was independent of stress. Even so, pre-stress results were not presented, so stress may have contributed somewhat to the findings. For example, the fact that the S/S′ group made more mistakes in the subtraction task under placebo may indicate that subjects with this genotype were not coping as well with the stressful aspect of the task: that this detriment was removed by TRP treatment strongly suggests it reflected suboptimal 5-HT function during a demanding task. It is also important to note that this sample consisted of twenty-eight women and only two men.
A subsequent report from this group(Reference Markus and De Raedt113) used the same stressor and TRP treatment to examine interactions of treatment, stress and 5-HTTLPR genotype on another measure of mood (Positive and Negative Affect Schedule) and attentional bias (inhibitory responses) to negative emotional stimuli. This bias was measured by reaction times to facial expressions varying in emotional valence and primed by previous stimuli of the same or opposite valence (negative affective priming). This study appears to have used the same participants as Markus and Firk(Reference Markus and Firk112) except excluding the two men (i.e. twenty-eight women). In the placebo condition, negative affect increased after stress only for the S/S′ genotype group, and furthermore this rise in negative mood was prevented by TRP treatment. For the negative affective priming task, there was an interaction between stress and genotype, such that S/S′ subjects showed faster responding to congruently than incongruently primed negative expressions after stress, an indicator of reduced inhibition to negative affective stimuli. The L/L′ group showed the opposite response, suggesting that this allele may confer some resilience to effects of stress on emotional processing. However, no effects of TRP treatment were found for this behaviour, although, as the authors point out, the study has a relatively small sample size and may be underpowered to detect three-way interactions of this sort.
Subsequently, Markus et al.(Reference Markus, Verschoor and Smeets114), established a larger student cohort screened for 5-HTTLPR genotype, and studied nineteen female S/S′ and twenty-three female L/L′ homozygous allele groups, with about half of each group selected to be either high or low on restrained eating (Three Factor Eating Questionnaire(Reference Stunkard and Messick115)). This study investigated potential interactions between TRP treatment, 5-HTTLPR genotype, stress, restraint and emotional eating, in a double-blind placebo-controlled crossover design. Stress was elicited using a modified Trier Social Stress Test(Reference Kirschbaum, Pirke and Hellhammer116); TRP challenge was accomplished using an egg-white protein hydrolysate enriched with TRP (4-g dose given as a 200-ml drink, containing 0·24 g TRP; DSM, Delft; see earlier), v. a casein hydrolysate placebo (0·03 g TRP). Blood samples were taken for amino acid analysis 90 min after consuming the drinks, and four salivary samples were taken during the study to assess cortisol levels. Interestingly, there was a significantly greater increase in plasma TRP:LNAA ratio following TRP treatment for the L/L′ group (70 % increase) compared with the S/S′ group (30 % increase). However, although stress resulted in a rise in cortisol, there were no significant effects of either TRP treatment, genotype or restrained eating on cortisol in this study. Mood generally deteriorated from before to after the stress; of particular interest, the increase in anger after stress occurred in all groups except the L/L′ genotype group who had received TRP supplementation, in whom there was no change in anger following stress.
Liking (pleasantness of taste) for a variety of foods of different sensory categories (sweet or savoury, low- or high-fat) was assessed using ratings of images of the foods. Only the high-fat sweet food liking ratings showed significant effects: in the L/L′ allele group, liking for high-fat sweet foods declined following stress only when given the TRP supplement, whereas there were no significant changes to liking ratings for the S/S′ allele group. Actual food intake was assessed by offering several snack foods (mini chocolate bars, pretzels and nuts) both before and after stress. The only significant result was a 38 % reduction in snack intake after TRP treatment (averaged across stress pre/post-measures); no effects of genotype, stress or restrained eating were seen. An overall appetite-suppressant effect of TRP may be expected, given that ATD tends to increase appetite(Reference Rieber, Mischler and Schumacher63), and higher doses of TRP (at least 2 g) have long been known to suppress appetite and reduce food intake by 10–20 %(Reference Hill, Blundell and Huether58); nevertheless, the dose of TRP effective here is considerably smaller (0·24 g), so the size of this effect is remarkable.
There are several intriguing findings in this study, not least the weaker increase in plasma TRP:LNAA ratio in the S/S′ subjects. The authors point out that this difference between genotypes is a unique finding, and speculate that it may be due to increased diversion of peripheral TRP to metabolism via the kynurenine pathway, due to induction of the hepatic TDO and peripheral indole 2,3-dioxygenase enzymes, which are known to be stress-sensitive(Reference Badawy14). However, direct evidence for such a mechanism reducing the TRP:LNAA ratio in S/S′ allele subjects after TRP supplementation is lacking. One study that measured 5-HTTLPR genotypes and administered 50 mg/kg TRP did assess the plasma kynurenine:TRP ratio as an index of TDO activity; however, this was in male patients with alcohol use disorder, and the study did not assess behavioural effects of TRP(Reference Vignau, Soichot and Imbenotte117). Those patients who experienced ‘blacked-out violent impulsive behaviour’ during binge drinking showed a higher kynurenine:TRP ratio than those who did not, suggesting that less TRP would be available to the brain. Nevertheless, no differences were reported for 5-HTTLPR genotype subgroups, although sample sizes may have been too small (nine cases, nine alcohol-dependent controls, received oral TRP) for meaningful statistics in this pilot study, and polymorphisms in the enzymes themselves were not measured. This may be important as there is evidence for example that the TPH1 218AA polymorphism is a risk factor for alcoholism and bipolar disorder(Reference Chen and Miller118). Anyhow, this impaired effect of TRP treatment on the plasma ratio in this S/S′ group(Reference Markus, Verschoor and Smeets114) may explain the lack of behavioural effects seen for this group in this study, in contrast to some effects that were specific to the L/L′ genotype. Conversely, the most likely explanation for a lack of stress-induced, or emotional, eating is the probability that few of the participants had emotional eating tendencies. Participants were selected on the basis of scores on the Three Factor Eating Questionnaire restrained eating scale, which, unlike some items on the disinhibition or hunger scales of this questionnaire, does not explicitly assess emotional eating and is usually orthogonal to it. We have argued previously that cognitive restraint per se is not a good predictor of stress eating tendencies(Reference Gibson119, Reference Oliver, Wardle and Gibson120). Furthermore, in a more recent study from this group, S/S′ allele subjects (both male and female) were shown to be more likely to eat sweet fatty foods after mild stress than L/L′ genotypes, an effect that was reduced by a sucrose preload(Reference Markus, Jonkman and Capello121). However, in that study, there was no manipulation by TRP load. Another study from this group investigated whether examination stress would differentially affect appetite for these two genotype groups(Reference Capello and Markus122): findings confirmed that the S/S′ genotype group was more likely to show stress-induced eating of sweet snacks, although again there was no manipulation of TRP.
Nevertheless, the interaction between genotype, stress, emotional eating and effects of subchronic TRP supplementation was investigated in mainly female participants (ninety-nine women, nineteen men) asked to self-administer 3 g TRP daily for 7 d (or placebo cellulose), before undergoing an acute stress test (repeated cold pressor and Serial-17 subtraction task known as the Maastricht Acute Stress Test)(Reference Capello and Markus123). Changes in appetite ratings, snack intake, mood and cortisol were assessed. Subchronic TRP treatment reduced the cortisol response to stress only in the S/S′ allele group. Similarly, the TRP treatment resulted in a significantly less stress-induced increase in anxiety only in the S/S′ group, but independently of trait neuroticism. Stress increased rated appetite, but interestingly TRP reduced this increase specifically in S/S′ subjects who also scored highly on neuroticism. The parallels across these TRP by genotype interactions are notable. By comparison, the only significant finding reported for post-stress snack intake was a greater intake of sweet fatty snacks by the low neuroticism v. the high neuroticism group, perhaps due to health concerns in the latter group. The interaction of genotype with neuroticism on the stress-induced change in rated appetite is similar to the results of an earlier study in which mainly female participants with low or high trait anxiety were subjected to stress (mental arithmetic during loud noise) and treated acutely with either TRP-rich α-lactalbumin or casein(Reference Verschoor, Finlayson and Blundell124). Food liking and preference was assessed by responses to food images displayed via a computer program(Reference Finlayson, King and Blundell125). While appetite ratings increased for all groups after stress, both liking and preference for sweet foods increased specifically for highly anxious participants, and these increases were prevented by α-lactalbumin treatment, implying that the increased desire for sweet food induced by stress in high-anxious participants was related to impaired 5-HT function. However, in this study, genotypes were not measured. Moreover, in the case of actual eating(Reference Capello and Markus122), it seems that other factors influenced the behaviour, although differences in timing between stress and food intake could be involved, and in this subchronic treatment design, no treatment was given on the test day.
Another group also examined effects of a similar subchronic TRP treatment (2·8 g/d for 6 d) on responses to stress (Trier Social Stress Test) in relation to 5-HTTLPR genotype(Reference Cerit, Jans and Van der Does126). In this study, about half the participants were female (twenty-two women, twenty-four men), although sex was included as a covariate in analyses, rather than reporting interactions with sex. There was a clear interaction between stress, genotype and treatment on salivary cortisol: S/S′ allele subjects on placebo (cellulose) showed the largest rise in cortisol induced by the stress, supporting a stress sensitivity of this genotype, but this effect was substantially reduced by prior TRP treatment (even though no TRP was taken on the test day): the lower cortisol response in L/L′ participants was not further reduced by TRP. However, while mood deteriorated after the stress, this was not differentially influenced either by treatment or genotype, contrary to Capello and Markus(Reference Capello and Markus123).
Subsequently, a recent study investigated whether a similar subchronic treatment with TRP (3 g/d for 7 d) could benefit the quality of sleep, and whether this might depend on 5-HTTLPR genotype(Reference van Dalfsen and Markus127). Thus, this study compared effects between S/S′ allele subjects (forty-six women, eleven men) and L/L′ allele subjects (forty-six women, eight men). Potential effects of neuroticism were investigated using a median split of questionnaire scores into high and low neuroticism groups. General sleep quality was assessed prior to treatment, then sleep quality after the week of treatment was measured for a further week. Higher neurotic participants tended to report lower general sleep quality, unrelated to genotype. However, following treatment, specifically S/S′ genotype together with higher neuroticism was associated with poorer sleep quality for the placebo group, but with better sleep quality for the TRP-treated group.
Finally, there is recent evidence of differential impact of 5-HTTLPR genotypes on mood changes during challenging tasks in the context of two intervention studies that had found beneficial effects of acute(Reference Gibson, Vargas and Hogan97) and chronic(Reference Mohajeri, Wittwer and Vargas98) treatment with a TRP-rich egg-white protein hydrolysate (DSM) on mood, emotional processing and cognition in Caucasian women aged 45–65 years(Reference Gibson, Mohajeri and Wittwer128). Participants were genotyped for the tri-allelic 5-HTTLPR polymorphism, and distributions of genotypes were in accordance with Hardy–Weinberg equilibrium (allele sample sizes; acute study: SS/SLG = 11, SLG/SLGLA = 36, LALA = 13; chronic study: SS/SLG = 13, SLG/SLGLA = 36, LALA = 10).
We planned to compare the two homozygous groups (SS/LG (designated S/S′) v. LALA (designated L/L′)) on behavioural outcomes; however, with several different treatment groups, cell sizes would be too small for meaningful analyses of treatment by genotype effects. Therefore, we examined outcomes on the pre-treatment baseline day, when the participants completed the same set of tests as during treatment, which allowed us to pool the outcome data for all participants within each genotype group. The series of cognitive and behavioural tests lasted for 3·5 h from the baseline (pre-test) mood measure to the final post-test mood measure, with 1 h of rest in between, so represented a challenging and potentially ego-threatening process for the participants. Furthermore, we compared pre-test to post-test changes only in those emotions that had proved responsive to subsequent TRP supplementation treatment. Specifically, these were wellbeing and fatigue in the acute study, and a positive feeling of high energy (stimulated, buzzing, impulsive) in the chronic study (emotions were derived by factor analyses of ratings on twenty-eight items presented on a computer, known as the Mental and Physical Sensations Scale). For the acute study, we found that well-being declined from pre- to post-test in the S/S′ group, but not in the L/L′ group, whereas fatigue increased significantly only for the S/S′ group. For the chronic study, high-energy mood increased from pre- to post-test for the L/L′ group, but did not change for the S/S′ group.
These differences in genotypes for mood changes during challenging and potentially stressful tasks are in line with evidence that the S/S′ genotype would confer greater risk of affective disorders such as anxiety or depression, or conversely a protective effect of the L/L′ allele, in women. Moreover, the known sensitivity of these changes in mood to TRP treatment supports mediation via changes in serotonin function.
Conclusions
The main theme emerging from the literature on TRP supplementation and genotypes is the observations of interactions between TRP and genotypes, sex and stress on changes in mood, cognition, cortisol and appetite. It is particularly important to consider the influence of a key ‘genotype’, sex. For example, in women, the 5-HTTLPR S/S′ genotype predicts sensitivity to improvements in the mood by TRP supplementation, especially during stressful challenges, whereas the L/L′ genotype tends to be protective against stress-induced mood deterioration and rise in cortisol, but may differ in sensitivity to TRP administration. In men, if anything, the L/L′ genotype confers risk of stress-induced increases in negative affect; however, there are insufficient studies with adequate power to detect sex × genotype × stress × TRP in the literature to draw strong conclusions.
Since the 5-HTTLPR genotypes may influence neurodevelopment and/or tonic 5-HT adaptive responsiveness at least as much as acute functioning of the brain serotonin system(Reference Shinozaki103, Reference Outhred, Das and Dobson-Stone129), it would be advantageous to assess extent of early life stress and/or stressful life events, as well as personality traits predictive of affective disorders, in studies of TRP effects on behaviour. However, when measuring multiple influences on behaviour, as well as sex differences, investigators need to ensure sufficiently large sample sizes to increase the likelihood of reliable findings(Reference Gressier, Calati and Serretti107): routinely screening for genetic polymorphisms in suitable populations would be helpful.
There is a need to broaden studies on the potential benefits of TRP supplementation to include a greater range of serotonin-related genotypes, including enzymes involved in key metabolic pathways (Fig. 1). This may eventually lead to clear predictions as to who is likely to benefit most from this relatively simple nutrient-based treatment. Until then, although there is preliminary evidence that individuals with some genotypes, particularly the 5-HTTLPR S/S′ allele in women, may benefit from TRP supplementation as an aid to stress coping and emotional regulation including comfort eating, further research is needed before reliable recommendations can be made on targeted use of TRP treatment, or adjustment of dietary TRP intake, for beneficial behavioural outcomes.
Acknowledgements
The author grateful to the Nutrition Society, UK, and the Royal Society of Medicine, London, for inviting me to present a review of this topic at their Winter Conference on Diet, Nutrition and Mental Health and Wellbeing, December 2016.
Financial Support
The author has received research funding from DSM Nutritional Products Ltd., Basel, Switzerland.
Conflicts of Interest
The sponsors had neither influence on conducting the experiments beyond consultation over design, nor on statistical analyses. The sponsors did not contribute to this review.
Authorship
E. L. G. is solely responsible for authorship of this article.