Introduction
Earth and Mars probably resembled each other in the environmental conditions early in their history (Strasdeit, Reference Strasdeit2010). This makes it conceivable that life may have evolved on both planets. Although today the surface of Mars is a hostile place (McKay, Reference McKay2010), the climate was warmer and wetter during the late Noachian and early Hesperian periods, as indicated by the mineral composition of the crust and the presence of certain geological structures (Carr, Reference Carr2012; Ehlmann and Edwards, Reference Ehlmann and Edwards2014). Later, gradual cooling and aridification of Mars, related to decreasing volcanic activity, drastically reduced the surface habitability (Jakosky and Phillips, Reference Jakosky and Phillips2001; Solomon et al., Reference Solomon, Aharonson, Aurnou, Banerdt, Carr, Dombard, Frey, Golombek, Hauck, Head, Jakosky, Johnson, McGovern, Neumann, Phillips, Smith and Zuber2005; Kite, Reference Kite2019). Consequently, potential life either had to escape the harsh surface conditions, for example by migration into the subsurface, became dormant or went extinct (Schulze-Makuch et al., Reference Schulze-Makuch, Fairén and Davila2008; Westall et al., Reference Westall, Foucher, Bost, Bertrand, Loizeau, Vago, Kminek, Gaboyer, Campbell, Bréhéret, Gautret and Cockell2015).
The search for life on Mars means searching for biosignatures such as intact microorganisms, metabolites, cell residues, biomolecules and their degradation products, physical structures (e.g. microfossils) and biominerals (Westall and Cavalazzi, Reference Westall, Cavalazzi, Reitner and Thiel2011). Thermal processes together with minerals in the surrounding soil or rock matrix may have transformed chemical biosignatures into various relatively stable organic and inorganic compounds. These compounds may give hints about the existence of present or former life. Eruption events were an important source of thermal energy on Mars. Indeed, Mars' early history up to the end of the Hesperian period was characterized by volcanic activity resulting in lava flows and basin-filling lava flood events (Greeley and Spudis, Reference Greeley and Spudis1981; Xiao et al., Reference Xiao, Huang, Christensen, Greeley, Williams, Zhao and He2012). Martian volcanism, including lava flows, extended to geologically recent times (until a few tens of millions of years ago) (Hauber et al., Reference Hauber, Brož, Jagert, Jodłowski and Platz2011; Voigt and Hamilton, Reference Voigt and Hamilton2018).
Thermal decomposition products of biomolecules may still be detectable in Martian soil and rocks, particularly in the subsurface. Therefore, the rover of the ESA/Roscosmos' ExoMars mission will carry a drill to collect soil samples from a maximum depth of 2 m and a suite of analytical instruments to investigate the samples (Vago et al., Reference Vago and Westall2017). A visible and near-infrared spectrometer (Ma_MISS) for in situ mineralogical characterization of the bore hole is integrated in the drill tool (De Sanctis et al., Reference De Sanctis, Altieri, Ammannito, Biondi, De Angelis, Meini, Mondello, Novi, Paolinetti, Soldani, Mugnuolo, Pirrotta and Vago2017). The most interesting drill core samples will be investigated by MOMA (a gas chromatograph‒mass spectrometer), MicrOmega (a visible near-infrared hyperspectral microscope) and RLS (a Raman laser spectrometer) to identify potential biosignatures (Bibring et al., Reference Bibring, Hamm, Pilorget and Vago2017; Goesmann et al., Reference Goesmann, Brinckerhoff, Raulin, Goetz, Danell, Getty, Siljeström, Mißbach, Steininger, Arevalo, Buch, Freissinet, Grubisic, Meierhenrich, Pinnick, Stalport, Szopa, Vago, Lindner, Schulte, Brucato, Glavin, Grand, Li and van Amerom2017; Rull et al., Reference Rull, Maurice, Hutchinson, Moral, Perez, Diaz, Colombo, Belenguer, Lopez-Reyes, Sansano, Forni, Parot, Striebig, Woodward, Howe, Tarcea, Rodriguez, Seoane, Santiago, Rodriguez-Prieto, Medina, Gallego, Canchal, Santamaría, Ramos and Vago2017). Similarly, the Perseverance rover of NASA's Mars 2020 mission is equipped with a set of instruments capable of detecting chemical biosignatures (Farley et al., Reference Farley, Williford, Stack, Bhartia, Chen, de la Torre, Hand, Goreva, Herd, Hueso, Liu, Maki, Martinez, Moeller, Nelessen, Newman, Nunes, Ponce, Spanovich, Willis, Beegle, Bell, Brown, Hamran, Hurowitz, Maurice, Paige, Rodriguez-Manfredi, Schulte and Wiens2020).
Some classes of biomolecules are found in all known organisms. Examples are porphyrin-type compounds, proteins and phospholipids. For this work we chose haemin (also called chlorohaemin or α-chlorohaemin), cytochrome c and lecithin, which is a mixture of phospholipids, as representatives of these three classes of biomolecules. Haemin (Fig. 1(a)) is a natural oxidation product of the haem prosthetic group, to which it is closely related structurally (Umbreit, Reference Umbreit2007). The identification of alteration products of haem proteins is relevant to such diverse fields as geochemistry (Callot and Ocampo, Reference Callot, Ocampo, Kadish, Smith and Guilard2000), forensic science (see e.g. Doty et al., Reference Doty, Muro and Lednev2017), archaeology (see e.g. Dinegar, Reference Dinegar1982; Petrone et al., Reference Petrone, Pucci, Vergara, Amoresano, Birolo, Pane, Sirano, Niola, Buccelli and Graziano2018), paleontology (see e.g. Schweitzer et al., Reference Schweitzer, Marshall, Carron, Bohle, Busse, Arnold, Barnard, Horner and Starkey1997; Greenwalt et al., Reference Greenwalt, Goreva, Siljeström, Rose and Harbach2013) and astrobiology (Suo et al., Reference Suo, Avci, Schweitzer and Deliorman2007). Cytochrome c is an evolutionary ancient haem protein which occurs in all three domains of life and is typically involved in electron transfer reactions (Fitch, Reference Fitch1976; Bertini et al., Reference Bertini, Cavallaro and Rosato2006). Phospholipids are basic components of biological membranes (Van Meer et al., Reference Van Meer, Voelker and Feigenson2008).
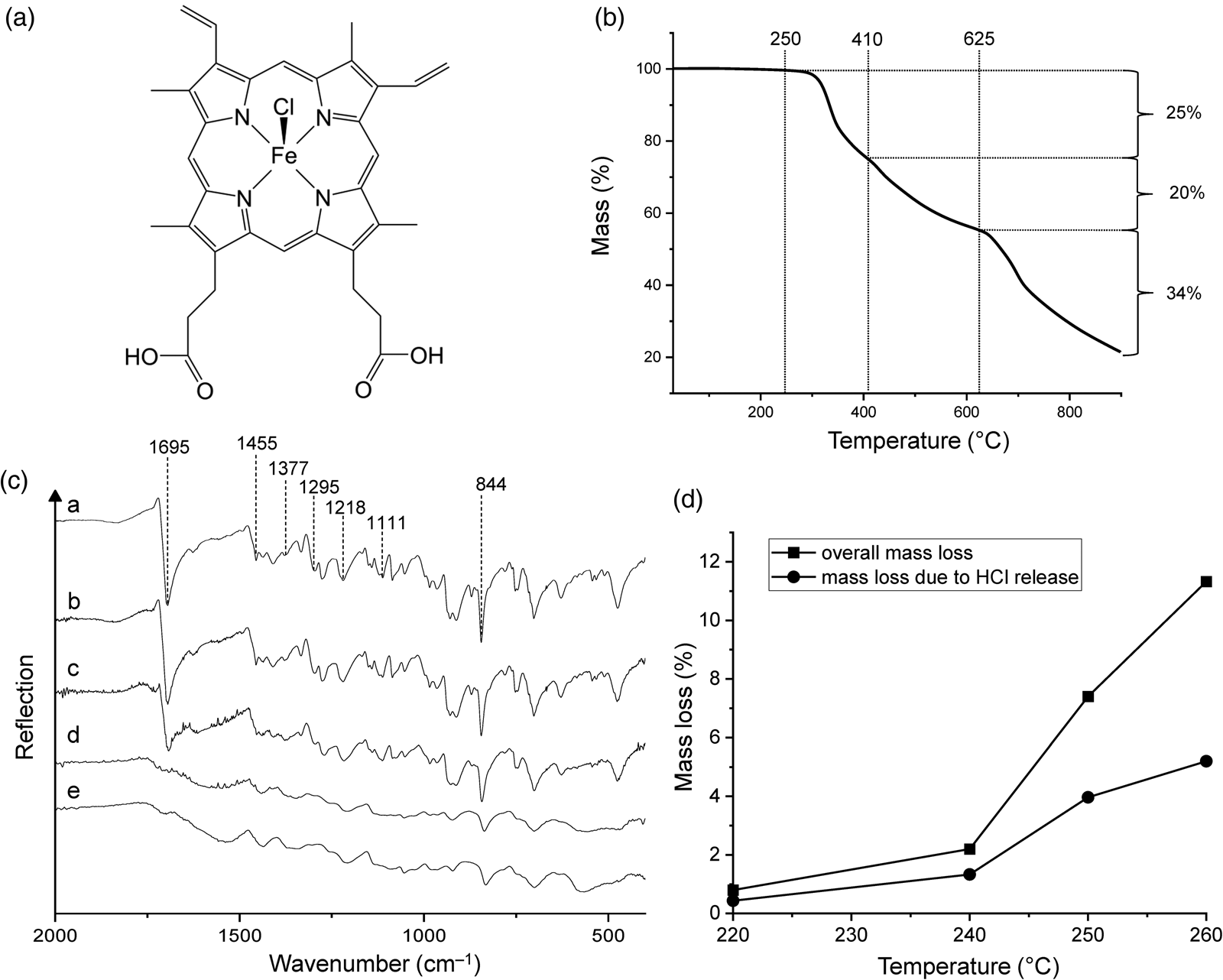
Figure 1. (a) Chemical structure of haemin. (b) Thermogravimetric analysis of haemin under nitrogen gas at a heating rate of 5 K min‒1. (c) ATR infrared spectra of (a) haemin and of the products obtained from heating haemin at (b) 220°C, (c) 240°C, (d) 250°C and (e) 260°C. (d) Overall mass loss of haemin and mass loss due to HCl release after 48 h at different temperatures.
In what follows we describe the thermal decomposition of haemin, cytochrome c and lecithin and the properties of the residues formed. As minerals are known to influence the thermal behaviour of biomolecules (see e.g. Dalai et al., Reference Dalai, Pleyer, Strasdeit and Fox2017; Fox et al., Reference Fox, Pleyer and Strasdeit2019), we not only investigated the neat compounds, but also performed thermolysis in mineral matrices. The matrix materials used were sodium chloride (NaCl), gypsum (CaSO4 ⋅ 2H2O), the clay mineral Ca-montmorillonite STx-1b and the Martian regolith simulant JSC Mars-1A. Chlorides (possibly halite, NaCl), gypsum and montmorillonite are present at the Martian surface (Ehlmann and Edwards, Reference Ehlmann and Edwards2014). Clay minerals are alteration products of volcanic rocks and were detected in the oldest Martian terrains, including Oxia Planum, the landing site for the ExoMars rover (Mandon et al., Reference Mandon, Parkes Bowen, Quantin-Nataf, Bridges, Carter, Pan, Beck, Dehouck, Volat, Thomas, Cremonese, Tornabene and Thollot2021). The clay mineral STx-1b is particularly well characterized (Castellini et al., Reference Castellini, Malferrari, Bernini, Brigatti, Castro, Medici, Mucci and Borsari2017). The regolith simulant JSC Mars-1A used in this study consists of altered volcanic ash (palagonite with relict plagioclase and clinopyroxene; Allen et al., Reference Allen, Jager, Morris, Lindstrom, Lindstrom and Lockwood1998; Cloutis et al., Reference Cloutis, Mann, Izawa, Applin, Samson, Kruzelecky, Glotch, Mertzman, Mertzman, Haltigin and Fry2015).
The aim of this work was to determine whether thermal alteration of biomolecules (including chemical interaction with minerals) forms organic and inorganic materials that can be useful as biosignatures. Such information may be helpful in interpreting data from current and future Mars missions.
Materials and methods
Chemicals and minerals
The following materials were used as received: haemin (Roth, ≥ 98%), cytochrome c (Sigma-Aldrich, ≥ 95%, oxidized form [ferricytochrome] with a reduced cytochrome c content ≤ 5%), soybean lecithin (Roth, ≥ 97% phospholipids), sodium chloride (VWR, analytical reagent), calcium sulphate dihydrate (gypsum, Sigma-Aldrich, Taufkirchen, Germany, ≥ 99%), STx-1b (The Clay Minerals Society, Chantilly, Virginia, USA) and JSC Mars-1A (Orbital Technologies Corporation [ORBITEC], Madison, Wisconsin, USA).
Pellet preparation
The biomolecules were used both as neat powders and as mixtures with the mineral matrices. The mixtures were studied as compact samples (pellets) which were prepared as follows. The organic and the mineral component were dry-mixed and ground together in an agate mortar and pestle for ~2 min. The resulting average grain size was less than 10 μm, except for some haemin crystals which were up to 50 μm long. Then 200 mg of the resulting powder were pressed into a pellet using a hydraulic press. The pellets had a diameter of 13 mm and were ~1 mm thick. The mixtures with CaSO4 ⋅ 2H2O, STx-1b or JSC Mars-1A contained 30% of the organic component. When NaCl was used, 10% of the organic component were usually sufficient because there was less analytical interference from the matrix material. An exception was the haemin‒NaCl sample used for thermolysis at 800°C and subsequent X-ray powder diffraction (XRD) analysis, which was prepared with 30% of haemin.
Tube furnace experiments
The thermolysis apparatus used was essentially the one described by Fox and Strasdeit (Reference Fox and Strasdeit2013). It consisted of a quartz tube positioned in a horizontal tube furnace. The tube was capped with two end pieces carrying the gas inlet and outlet. The open sample container was also made of thermally and chemically resistant quartz glass. After the container with the sample had been placed into the tube, the whole system was purged with nitrogen for at least 24 h to remove any oxygen. The sample was placed either (i) in the heating zone of the furnace before the temperature was raised or (ii) in the part of the tube outside the furnace and pushed into the heating zone when the furnace had reached its final temperature. In the first case the temperature was raised with a heating rate of 5 K min‒1. The final temperature was between 150 and 900°C. Depending on the experiment, this temperature was held constant for 12 or 48 h. All experiments were performed under a slow stream of nitrogen. In some experiments, cold traps or gas washing bottles were inserted between the quartz tube and the gas outlet to collect volatile products.
Analytical instrumentation
Thermogravimetric (TG) analysis was performed with a TA Instruments TGA 550 in a nitrogen atmosphere (≥99.999%, ≤3 vol ppm O2 content). Samples of 1–8 mg were heated in platinum pans. The heating rate was 5 K min‒1 and the temperature range was from room temperature to 900°C.
Transmission and ATR infrared spectra were obtained with a Nicolet 5700 FT-IR spectrometer (Thermo Fisher Scientific). For measurements in transmission mode, samples were embedded in NaCl (VWR, p.a.) or KBr (Roth, p.a.) pellets. The spectral range extended from 400 to 4000 cm–1, corresponding to 2.5‒25 μm. Each spectrum was the average of 600 scans with a spectral resolution of 2 cm–1.
XRD data were collected on a Bruker D8 Focus diffractometer using CuKα radiation. Diffracted radiation was detected by a Sol-X energy dispersive detector except for the 800°C sample of haemin‒NaCl (Fig. S2(c)), for which we used an SSD 160 detector (1D Si strip, nickel filter). Data were recorded in the 2Θ range 5‒90° with a step size of 0.02° and counting times of 1 s per step (Sol-X) and 20 s per step (SSD 160). Reference diffractograms for haematite (RRUFF ID R040024), magnetite (RRUFF ID R060656), cementite (cohenite, RRUFF ID R100076) and sodalite (RRUFF ID R040141) were obtained from the RRUFF database (https://rruff.info/; Lafuente et al., Reference Lafuente, Downs, Yang, Stone, Armbruster and Danisi2015). Reference diffraction data for α-iron (database code 0011214) are available in the American Mineralogist Crystal Structure Database (http://rruff.geo.arizona.edu/AMS/; Downs and Hall-Wallace, Reference Downs and Hall-Wallace2003).
Gas chromatography–mass spectrometry was performed with an Agilent 6890N/5973 GC/MSD system equipped with a DB-5MS column. Helium was used as carrier gas. Volatile products that were condensed in cold traps during thermolysis experiments were extracted with dichloromethane and then transferred to the GC-MS.
Chemical analyses
In order to quantify the evolution of HCl from haemin in moderate-temperature experiments (220–260°C), two gas washing bottles connected in series were inserted between the quartz tube and the gas outlet of the thermolysis apparatus. The bottles were filled with a known amount of NaOH in aqueous solution. The gas flow was passed through the solutions by using fritted gas dispersion tubes. At the end of the thermolysis experiment, the amount of HCl absorbed was determined by the Mohr titration method: Chloride was precipitated as AgCl by titration with a AgNO3 solution. K2CrO4 was used as indicator, and the end-point of the titration was reached when the formation of red-brown Ag2CrO4 started.
In order to quantify the loss of CO2 from haemin at 260°C, two gas washing bottles filled with Ba(OH)2 solution were inserted between the quartz tube and the gas outlet of the thermolysis apparatus. The CO2 in the gas flow reacted with the Ba(OH)2 to form solid BaCO3. The amount of CO2 released was then determined by back titration of excess Ba(OH)2 with oxalic acid solution, with phenolphthalein as indicator. Ba(OH)2 also reacted with the HCl evolved during the experiment, but we were able to take this into account because HCl was quantified independently (see above).
Elemental analyses were performed by Mikroanalytisches Labor Pascher, Remagen, Germany. The thermolysis residues of haemin had the following elemental compositions (in %): 260°C (means from two independent experiments): C 63.93, H 4.60, N 9.85, Cl 0.21, Fe 9.40, O (calculated by difference) 12.01; 500°C: C 70.56, H 1.61, N 11.1, Fe 11.5. These residues were hygroscopic. Therefore, the samples were dried at 60°C before analysis whereby the 260°C samples on average lost 5.5% of their mass. The total water content was ~12% (determined by drying at 150°C for 24 h). Thus, the 260°C samples used for elemental analysis contained ~6.5% ( = 12 ‒ 5.5%) water.
The residue from heating lecithin at 500°C was dried in vacuo at 60°C before analysis. Its elemental analysis gave the following results: C 50.57, H 1.62, N 2.11, P 14.5, O (calculated by difference) 31.2.
Results and discussion
Neat biomolecules
Haemin
Haemin is an iron(III) complex of protoporphyrin IX and chloride which contains iron in a square-pyramidal ClN4 environment. Its structural formula is shown in Fig. 1(a). Iron porphyrins such as haemin are attractive biosignatures in the search for extraterrestrial life (Pleyer et al., Reference Pleyer, Moeller, Fujimori, Fox and Strasdeit2022). In crystals of haemin the molecules are linked pairwise by hydrogen bonds between propionic acid side chains (‒CH2CH2COOH) (Koenig, Reference Koenig1965; Kaduk et al., Reference Kaduk, Wong-Ng, Cook, Chakraborty, Lapidus, Ribaud and Brewer2016). One of the carboxyl groups is in the vicinity of the chlorido ligand of a neighbouring molecule. Even though this is not a hydrogen-bonding interaction, it may play a role in the thermal decomposition pathway of solid haemin (see below).
TG analysis gave an initial picture of the overall thermal behaviour of haemin (Fig. 1(b); see Fig. S1(a) for the first derivative curve). The decomposition onset temperature was determined to be ~250°C. The TG curve showed three major mass loss steps at 250‒410, 410‒625 and 625‒900°C. The latter is caused by at least two thermal processes and was still incomplete at the end of the measurement. The corresponding mass losses were 25, 20 and 34%, and thus the total mass loss at 900°C was 79%. Wang et al. (Reference Wang, Zhou, Chen, Lin, Ke, Xu and Sun2010) reported a smaller total mass loss under similar conditions (45% at 888°C), but the general TG curve shape was the same as in our experiments.
Figure 1(c) shows the infrared spectra of haemin and its 220, 240, 250 and 260°C thermolysis products. Diagnostic spectral features of untreated haemin include the strong C = O stretching band (1695 cm–1), C–C (1455 cm–1) and C–N stretching bands (1377 and 1111 cm–1) and CH2 and CH3 deformation bands (1295, 1218 and 844 cm–1; for band assignments see Dörr et al., Reference Dörr, Schade and Hellwig2008). After heating haemin at 240°C, the spectrum remained unchanged, demonstrating that the compound was essentially stable up to this temperature. However, when haemin was heated at 250°C, the bands were broadened, and most importantly, the C = O band was no longer present, indicating chemical transformation of the COOH groups. Concomitantly, a new, very broad band appeared at ~1560 cm–1, which slightly shifted to ~1540 cm–1 in the spectrum of the 260°C residue. We tentatively assign this band to the asymmetric stretching mode of COO– groups (see below).
The mass loss of haemin in constant temperature experiments at 220, 240, 250 and 260°C was 0.8, 2.2, 7.4 and 11.3%, respectively (Fig. 1(d)). Each experiment was conducted for 48 h. It should be kept in mind that the duration of the experiment is a key parameter that influences the mass loss at a given temperature. Our 48 h data indicate that the decomposition started slowly between 220 and 240°C, but it was too small to be detected by infrared spectroscopy. It should be noted that mass losses in constant temperature experiments occur at lower temperatures than in TG experiments (compare Fig. 1(b) and (d)). In other words, thermal processes appear delayed in TG curves, which is a well-known effect.
We identified two main processes that are involved in the initial decomposition of haemin, namely the protonation of the chlorido ligand (release of HCl) and the decarboxylation of one of the propionic acid side chains (release of CO2 and formation of an ethyl group). If these processes were complete, they would result in a theoretical mass loss of 12.34% (5.59% from HCl and 6.75% from CO2). This may be compared with the experimental value of 11.3% at 260°C, to which HCl contributed 5.2% (Fig. 1(d)) and CO2 6.3%. Hence, the ratio between the experimental and the theoretical mass loss is 0.92. The 260°C samples used for elemental analysis had a water content of ~6.5% (see Materials and methods section). If we therefore assume (i) 92% complete decarboxylation of one of the two propionic acid groups, (ii) 92% complete reaction of the other propionic acid group according to equation (1) and (iii) a water content of 6.5%, then we calculate the following composition (experimental values in parentheses): C 64.28 (63.93), H 5.80 (4.60), N 9.06 (9.85), Cl 0.46 (0.21), Fe 9.04 (9.40), O 11.36 (12.01). It can be seen that there is reasonable agreement between the theoretical and experimental values.
HCl was formed by proton transfer from a carboxyl group to a chlorido ligand according to the following equation:


It is expected from chemical reasoning that the carboxylate group takes the place of the chlorido ligand and a Fe‒OOC group is formed. The dimer in equation (1) only serves to illustrate the principle, and it is unknown whether it is an actual intermediate. Condensation products such as this one will react further by release of CO2 and as proton donors to Cl‒ until all carboxyl groups have been transformed. This explains the absence of the C = O stretching band in the infrared spectra of the 250 and 260°C thermolysis products. The products were sparingly soluble in common organic solvents, indicating a polymeric structure. Their idealized formula is Fe{PP(H)(COO)} where H stands for the ethyl group formed by decarboxylation. The presence of carboxylate groups is supported by a band at ~1540 cm–1 in the infrared spectrum which can be assigned to the COO– asymmetric stretching mode (Deacon and Phillips, Reference Deacon and Phillips1980). There are also candidates for the symmetric stretching band, but the assignment is ambiguous. Reaction 1 is probably facilitated by the relatively short distance of 4.11 Å between the chlorido ligand and a carboxyl O atom of an adjacent molecule in haemin crystals (Koenig, Reference Koenig1965).
The compound β-haematin (haematin anhydride, haemozoin, malaria pigment) is worth mentioning here. It consists of cyclic [Fe{PP(COOH)(COO)}]2 dimers in which the monomers are connected by two Fe‒OOC bonds (see e.g. Bohle et al., Reference Bohle, Dodd and Stephens2012; Straasø et al., Reference Straasø, Marom, Solomonov, Barfod, Burghammer, Feidenhans'l, Als-Nielsen and Leiserowitz2014). β-Haematin can be prepared by HCl abstraction from haemin in solution, a process analogous to reaction 1. Note that in β-haematin half of the carboxyl groups are still intact, in contrast to our thermolysis products.
The iron porphyrin core was still present after treatment at 260°C, but this potential biosignature was progressively lost with increasing temperature. In 500 and 900°C experiments, the formation of carbonaceous material (amorphous carbon, graphite), iron oxides (magnetite Fe3O4, haematite Fe2O3), cementite (Fe3C) and elemental iron (α-Fe) was observed (Fig. 2). In these experiments, haemin was first heated at 500°C for 48 h. Then half of the sample was removed for X-ray diffraction analysis. The diffractograms revealed the presence of amorphous carbon and magnetite (see e.g. Mochidzuki et al., Reference Mochidzuki, Soutric, Tadokoro, Antal, Tóth, Zelei and Várhegyi2003; Shi et al., Reference Shi, Che, Liang, Xia and Zhang2015). The other half of the sample was further heated at 900°C for another 48 h. The diffractogram of the 900°C residue was dominated by graphite and α-iron peaks with smaller peaks of magnetite and cementite (Fig. 2(a)). Obviously, the iron oxides and amorphous carbon which were present at 500°C reacted at higher temperatures to form metallic iron. This process is reminiscent of the reduction of FeO to Fe by carbon-bearing sediments in a basaltic melt (Klöck et al., Reference Klöck, Palme and Tobschall1986).
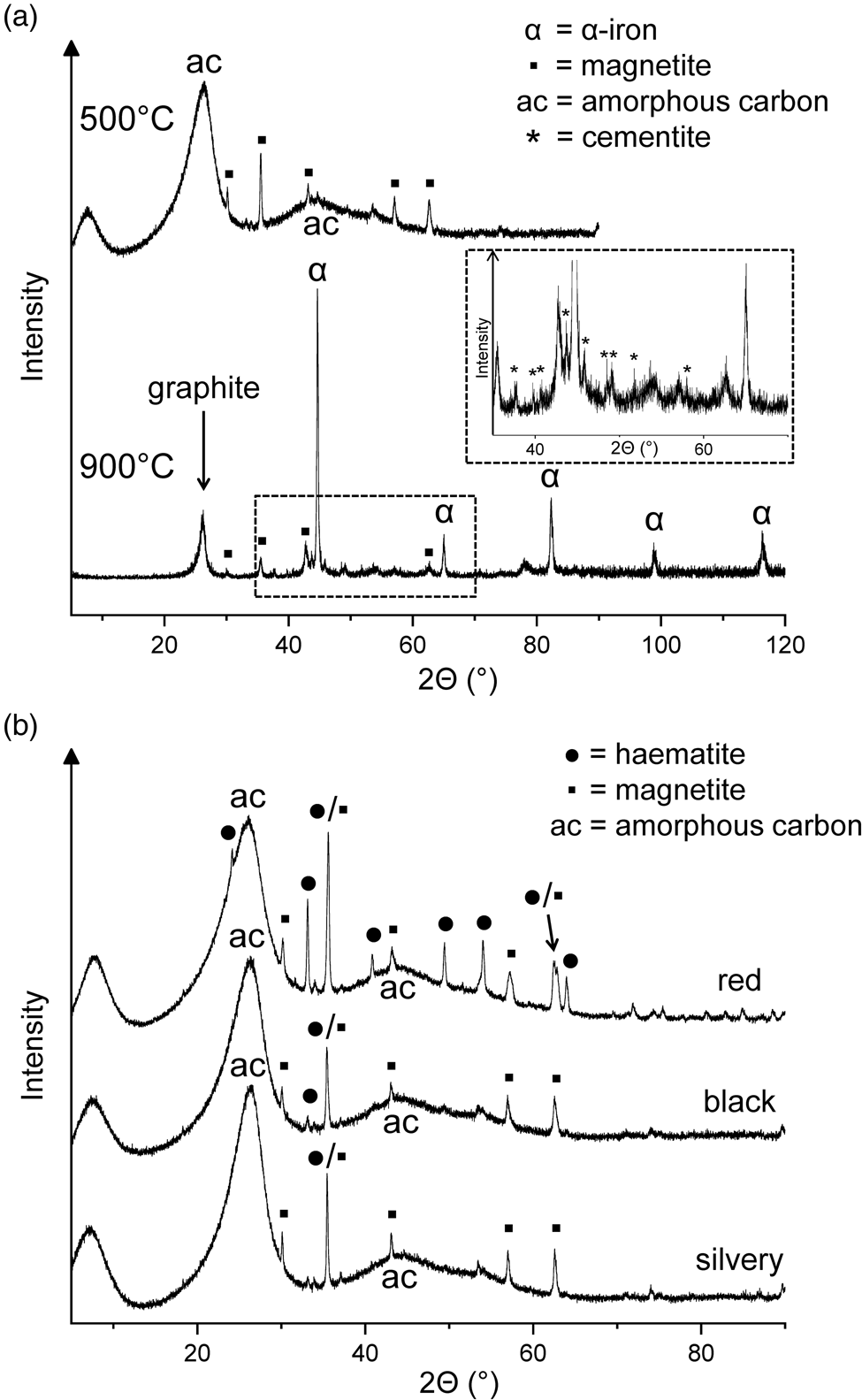
Figure 2. (a) X-ray diffractograms of the residues obtained by heating haemin at 500°C for 48 h and at 900°C for another 48 h. (b) X-ray diffractograms of three differently coloured fractions of a residue obtained at 500°C from haemin.
One of the 500°C samples contained three differently coloured fractions (red, black and silvery), which could be manually separated. In addition to two prominent peaks of amorphous carbon, the X-ray diffractograms showed iron oxide peaks with haematite dominating in the red fraction and magnetite in the other two fractions (Fig. 2(b)). The iron oxides probably resulted from decomposition of the initially formed Fe‒OOC groups (see above). Among the volatile products collected during the 500°C treatment of haemin, 2,3,4,5-tetramethylpyrrole and one of the isomers of ethyltrimethylpyrrole were identified by gas chromatography–mass spectrometry. However, the overall loss of volatile nitrogen compounds was small, judging from the N/Fe atom ratio of 3.85 in the solid residue, which was not significantly different from the value of 4.00 for haemin.
The question arises whether the high-temperature residues obtained from haemin at 500 and 900°C can be useful as biosignatures. Their formation is restricted to environments where the O2 partial pressure is sufficiently low, as for example in the Martian atmosphere (Krasnopolsky, Reference Krasnopolsky2011). Otherwise burning would produce iron oxides as the only solid residues. The N/Fe atom ratio of ~ 4 present in the 500°C residue may be used as an indication that the product originated from an iron porphyrin, but in organisms iron porphyrins are embedded in proteins which would also contribute nitrogen. The close association of amorphous carbon with iron oxides and graphite with metallic iron is due to the special nature of haem as an iron-containing organic compound. However, it is unclear whether these specific constellations of products can serve as biosignatures. Additional methods such as stable isotope ratio measurements may provide further indications of possible biogenicity. But certainly the high-temperature residues are not ‘strong’ chemical biosignatures in the sense discussed by Fox and Strasdeit (Reference Fox and Strasdeit2017).
Cytochrome c
Figure 3(a) shows the TG curve of cytochrome c. The corresponding first derivative curve can be found in Fig. S1(b). Evaporation of weakly bound water caused a mass loss of 3% during nitrogen purging at room temperature (hence the curve starts at 97%) and another 3% up to 130°C. This was followed by a major mass loss of 59% centred at 317°C and ending at ~470°C. The TG curve appears symmetrical in this temperature range. However, given that cytochrome c is a complex molecule and the mass loss is large, this step must consist of multiple chemical processes. Two further steps decreased the mass by an additional 30%, leading to a residual mass of 5% at 900°C. Anhydrous cytochrome c has an iron content of 0.45%. Therefore, the theoretical iron content of the 900°C residue was ~9%, under the reasonable assumption that no iron was lost on heating. Our TG results on cytochrome c essentially match those of Campos et al. (Reference Campos, Nantes, Rodrigues and Brochsztain2004). Notable differences are that the literature curve is less structured and that the major mass loss step was observed at a higher temperature (~395°C compared to 317°C) with a larger mass loss (~76% compared to 59%). These deviations can be attributed to differences in experimental conditions, mainly to the considerably higher heating rate used in the literature study (20 K min‒1).
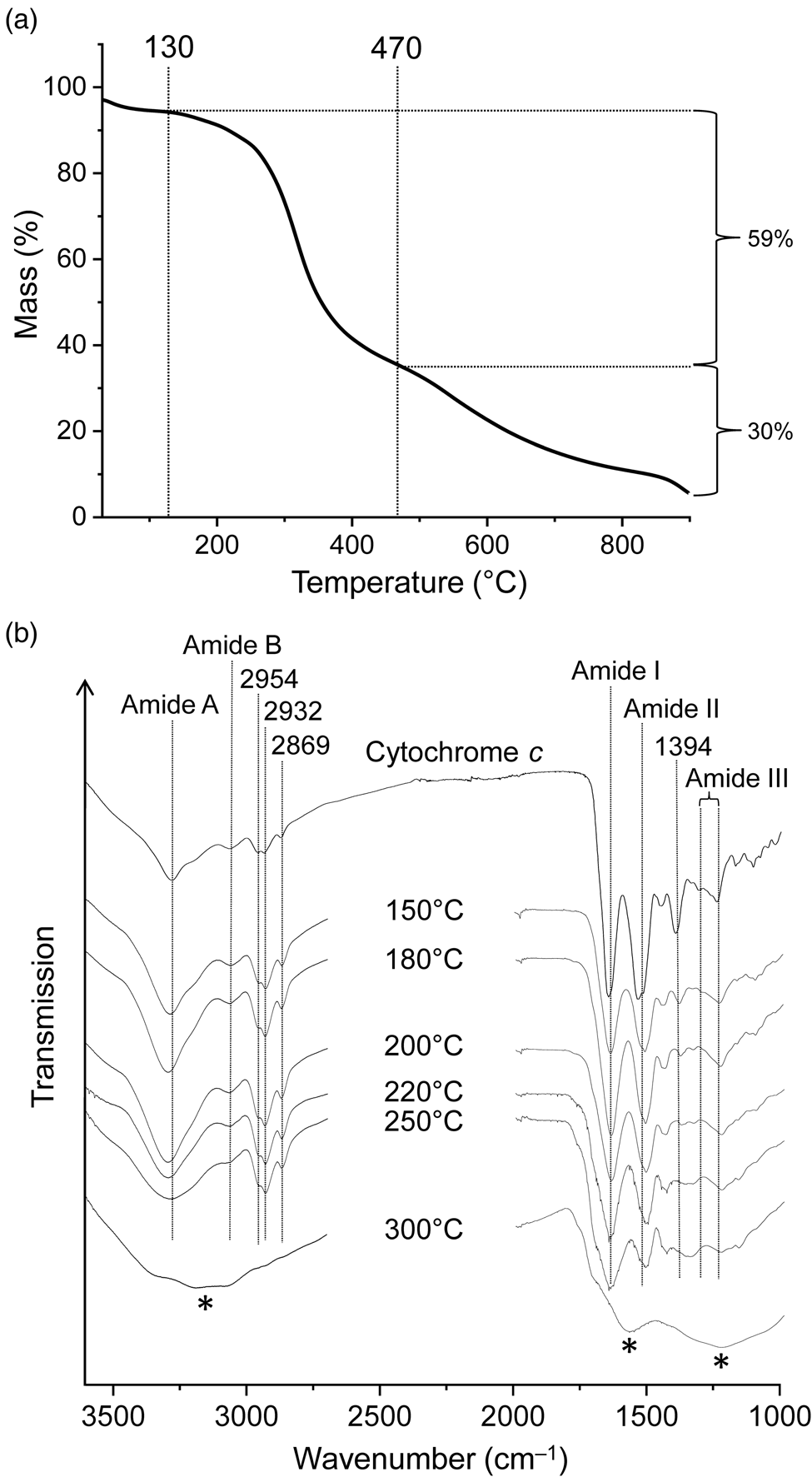
Figure 3. (a) Thermogravimetric analysis of cytochrome c under nitrogen gas at a heating rate of 5 K min‒1. (b) Infrared spectra of cytochrome c and its thermal residues. New bands in the 300°C spectrum are marked by asterisks. The samples were measured in transmission mode in KBr pellets.
Infrared spectra of proteins show characteristic absorptions of the backbone amide groups. In the infrared spectrum of cytochrome c (Fig. 3(b)), such absorptions were found at 3275 and 3062 cm–1 (amide A and B band respectively, NH stretching), 1645 (amide I band, mainly C = O stretching), 1524 (amide II band, maximum at 1533 cm–1, mainly NH bending and CN stretching) and 1240–1308 cm–1 (amide III region). The bands were assigned in accordance with the literature (Smith and Franzen, Reference Smith and Franzen2002; Barth, Reference Barth2007). Absorptions at 2954, 2932 and 2869 cm–1 are caused by CH stretching vibrations. The 1394 cm–1 band is tentatively assigned to side chain vibrations; in particular, the symmetric COO– stretching modes of aspartate and glutamate residues are known to occur in this spectral region (Barth, Reference Barth2007; Parikh et al., Reference Parikh, Kubicki, Jonsson, Jonsson, Hazen, Sverjensky and Sparks2011).
Exposure of cytochrome c to 150°C resulted in small but significant spectral changes (Fig. 3(b)). For example, the relative intensities of absorptions contributing to the amide II band changed (shifting the band maximum from 1533 to 1514 cm–1) and the 1394 cm–1 band decreased in intensity and shifted to 1386 cm−1. The infrared spectrum progressively changed with increasing temperature. While the 250°C spectrum is still recognizable as that of peptides or at least amides, the 300°C spectrum is completely different and shows none of the characteristic amide bands and also no CH stretching bands. Clearly the peptide structure was completely destroyed at temperatures between 250 and 300°C, and the residue was no longer an obvious biosignature. The 300°C spectrum is almost unstructured with broad bands around 3150, 1575 and 1230 cm–1 (Fig. 3(b)). It is reminiscent of the spectra observed for some carbonaceous materials with low H/C atomic ratios (see e.g. Russo et al., Reference Russo, Stanzione, Tregrossi and Ciajolo2014).
Lecithin
Soybean lecithin, which was used in this study, has been reported to contain the phosphodiester L-α-phosphatidylcholine (Fig. 4(a)) as a major component (Scholfield, Reference Scholfield1981). In the TG curve of lecithin (Fig. 4(b); see Fig. S1(c) for the first derivative curve), three mass loss steps were observed between 145 and ~510°C, in good agreement with the results of Nirmala et al. (Reference Nirmala, Park, Navamathavan, Kang, El-Newehy and Kim2011). The associated mass losses were 5, 70 and 8%. Slow mass loss continued beyond 510°C, and the total mass loss reached 88% at 900°C. The curve shape suggests that the second and largest decomposition step consists of different chemical processes, which is reasonable since lecithin is a mixture of many compounds. The temperature range of this step (190‒385°C) encompasses the range in which characteristic infrared bands disappear and the utility of lecithin as a biosignature decreases strongly (see below).
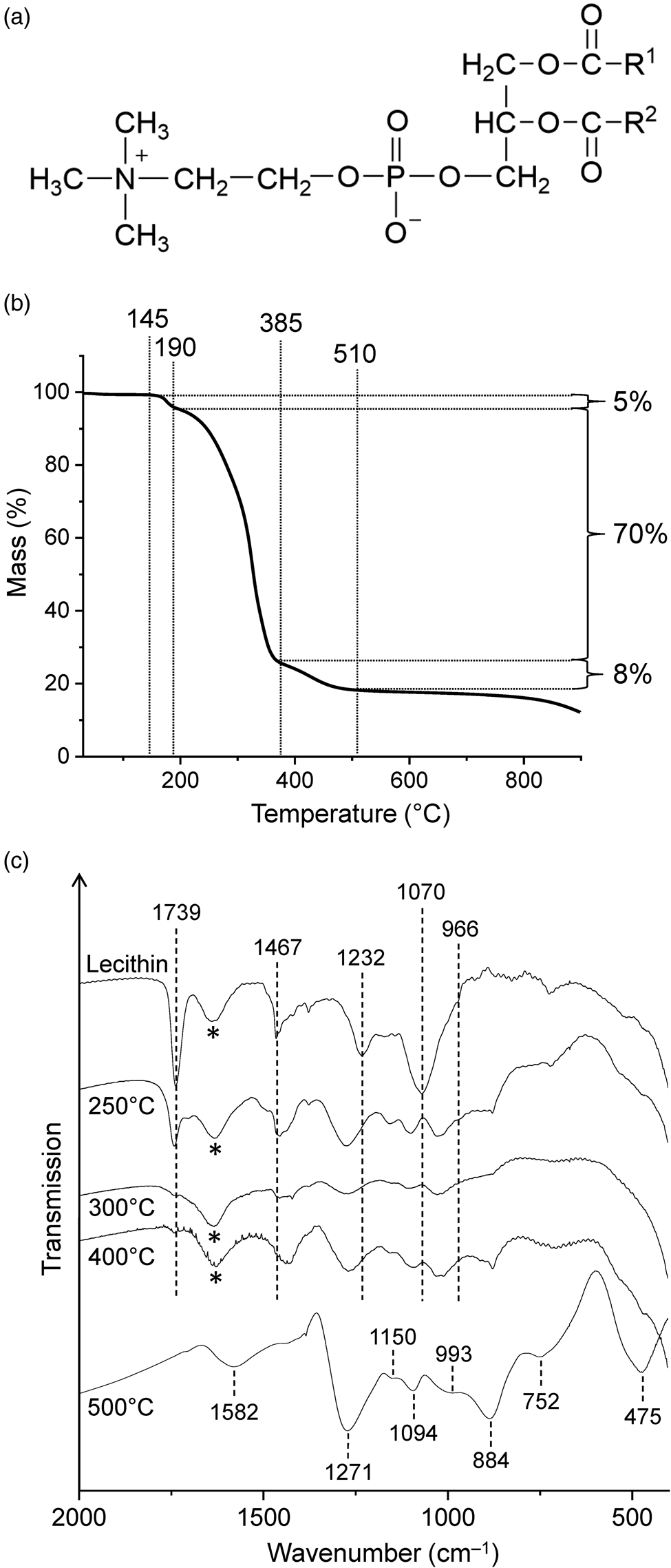
Figure 4. (a) Chemical structure of L-α-phosphatidylcholine, a major component of lecithin (R1, R2 = fatty acid chain). (b) Thermogravimetric analysis of lecithin under nitrogen gas at a heating rate of 5 K min‒1. (c) Infrared spectra of lecithin and its thermal residues. The samples were measured in transmission mode in NaCl pellets except the 500°C residue which was measured in KBr. Asterisks mark the water bending band.
The infrared spectrum of lecithin (Fig. 4(c)) showed a sharp, strong absorption at 1739 cm–1 due to the ester C = O stretching vibrations and three other prominent bands in the fingerprint region at 1467 (CH2 scissoring), 1232 (asymmetric PO2– stretching) and 1070 cm–1 (symmetric PO2– stretching). The band assignments were made according to Tantipolphan et al. (Reference Tantipolphan, Rades, McQuillan and Medlicott2007) and Kudo and Nakashima (Reference Kudo and Nakashima2020). The shoulder at 966 cm–1 was assigned to the asymmetric N–(CH3)3 stretching vibration of the choline group (Popova and Hincha, Reference Popova and Hincha2003). In addition, four bands at 3010, 2956, 2925 and 2853 cm–1 were observed in the CH stretching region (not shown in Fig. 4(c)).
After treatment at 250°C, most infrared absorptions of lecithin were still clearly evident, even though the maxima were shifted (Fig. 4(c)). The intensities also changed. For example, the C = O band was much weaker than in the spectrum of untreated lecithin, indicating that substantial decomposition occurred at 250°C. This finding is consistent with the TG data, which show that the onset temperature of the major mass loss step was 190°C (Fig. 4(b)). In contrast to the 250°C spectrum, lecithin was no longer recognizable in the spectrum of the 300°C residue. Thus in this respect lecithin behaved similarly to cytochrome c (see above).
New bands appearing at 1271, 1150, 1094, 993, 884, 752 and 475 cm‒1 in the 500°C spectrum (Fig. 4(c)) strongly suggest the presence of linear long-chain polyphosphates (Corbridge and Lowe, Reference Corbridge and Lowe1954; Bues and Gehrke, Reference Bues and Gehrke1957; Rao et al., Reference Rao, Sobha and Kumar2001). Such polyphosphates are known as thermal condensation products of dihydrogen phosphate (Greenwood and Earnshaw, Reference Greenwood and Earnshaw1997). They have the general formula (PnO3n+1)(n+2)‒ or approximately (PO3‒)n for large values of n. The O/P atom ratio in the 500°C residue was 4.2 (see Materials and methods section) and thus more than sufficient for polyphosphates.
The presence of polyphosphates raises the question as to where the counter charge is located. We hypothesize that the carbonaceous matrix includes positively charged oxygen and nitrogen atoms, perhaps similar to those in pyrylium and quinolizinium cations, respectively (see e.g. Eicher et al., Reference Eicher, Hauptmann and Speicher2012). A very weak broad CH stretching band is observed at 3035 cm‒1 which is indicative of aromatic structures. There are no spectral features that can be attributed to specific cationic groups, but there is a conspicuous band at 1582 cm‒1, which lies in the region where hydrogen-poor carbonaceous materials have a characteristic absorption band (see e.g. Russo et al., Reference Russo, Stanzione, Tregrossi and Ciajolo2014). The spectra of these materials also show an extremely broad, weakly structured absorption extending from ~1000 to ~1450 cm–1, which may be obscured by polyphosphate bands in the spectrum of the 500°C residue of lecithin. The black appearance and the high carbon (50.57%) and low hydrogen content (1.62%) of the residue are also consistent with the presence of carbonaceous material.
Interestingly, after heating lecithin at 900°C, no solid residue was observed but the quartz sample container showed clear signs of corrosion. Indeed, condensed phosphates (‘metaphosphates’) are known to attack quartz glass at high temperatures (Barz et al., Reference Barz, Haase, Meyer and Stachel1996).
Biomolecules in mineral matrices
On rocky planets such as Mars non-volatile organic materials will usually be in long-term contact with surrounding minerals. This prompted us to investigate the thermal behaviour of biomolecule‒mineral mixtures. We limited our experiments to haemin and lecithin and the mineral matrices sodium chloride (NaCl, halite), Ca-montmorillonite STx-1b and Martian regolith simulant JSC Mars-1A. In the case of lecithin, also gypsum (CaSO4 ⋅ 2H2O) and a 1:1 mixture of NaCl and JSC Mars-1A were used as matrices. It is worth mentioning that gypsum loses most of its crystal water well below 200°C (Strydom et al., Reference Strydom, Hudson-Lamb, Potgieter and Dagg1995).
Figure S2 shows the X-ray diffractograms of residues obtained by heating haemin at 800°C in three different mineral matrices (STx-1b, JSC Mars-1A and NaCl). Graphite, α-iron and cementite were found in all cases. The diffractogram of the haemin‒NaCl residue also contained magnetite peaks. Thus, the same products were observed as in the 900°C experiment with neat haemin (see above).
In some cases, the thermal treatment of lecithin in a mineral matrix gave products not observed from lecithin alone. In sodium chloride, for example, the phosphate esters decomposed to form the diphosphate anion (pyrophosphate, P2O74‒), as is evident from the infrared spectra of the residues obtained between 400 and 700°C (Fig. 5(a)). The characteristic bands of diphosphate (Fig. S3; see e.g. Cornilsen and Condrate, Reference Cornilsen and Condrate1978) are most clearly seen in the 500°C spectrum. If diphosphate was also formed from neat lecithin, the amount was too small to be detected by infrared spectroscopy; indeed, it appeared that long-chain polyphosphates predominated (see above).
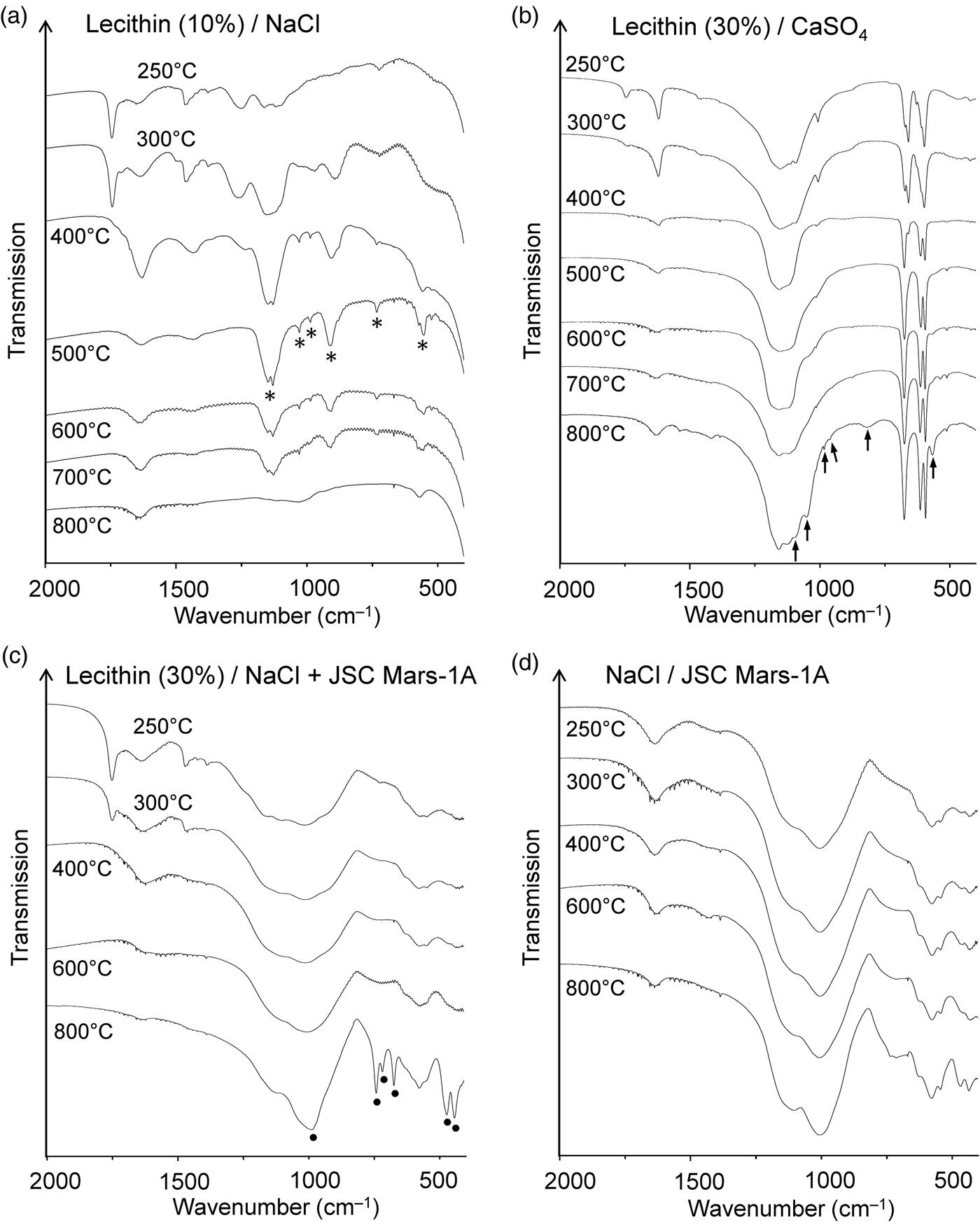
Figure 5. Infrared spectra of the residues obtained from heating lecithin in (a) NaCl, (b) gypsum and (c) a 1:1 mixture of NaCl and JSC Mars-1A. Marked bands are assigned to diphosphate (*), phosphate-related anions (↑) and sodalite (●). (d) Spectra of the thermally treated mixture of NaCl and JSC Mars-1A for comparison. The samples were measured in transmission mode in NaCl pellets.
After heating lecithin‒gypsum mixtures the lecithin C = O stretching band at ~1739 cm–1 was still visible at 250°C but had virtually disappeared at 300°C (Fig. 5(b)). This behaviour was very similar to that of neat lecithin (Fig. 4(c)). The infrared spectrum of the residue from the thermolysis of lecithin‒gypsum at 800°C showed several new bands. At this temperature gypsum is completely dehydrated. Accordingly, some of the bands could be unambiguously assigned to anhydrous CaSO4 (Makreski et al., Reference Makreski, Jovanovski and Dimitrovska2005). However, six weak to very weak bands at 566, 819, 963, 983, 1052 and 1095 cm–1 could not be attributed to CaSO4 (Fig. 5(b)). They lie in the spectral regions where the characteristic infrared absorptions of phosphates (PO43‒) occur (Jastrzębski et al., Reference Jastrzębski, Sitarz, Rokita and Bułat2011; Salama et al., Reference Salama, Neumann, Günter and Taubert2014).
The Martian regolith simulant JSC Mars-1A and the clay mineral STx-1b appeared to have no significant influence on the thermal decomposition of lecithin. The diagnostic ester band in the infrared spectrum (~1739 cm–1) disappeared above 300°C (JSC Mars-1A) and 250°C (STx-1b), respectively (Figs S4 and S5). Complete decomposition of the ester groups in this temperature range was also observed for neat lecithin and lecithin in NaCl and CaSO4. The infrared spectra of the residues formed from lecithin‒JSC Mars-1A and lecithin‒STx-1b between 250 and 800°C showed no bands that could be attributed to phosphate-related anions. If such anions had formed, their bands were too weak or broad to be observed against the matrix background.
When a 1:1 mixture of NaCl and JSC Mars-1A was used as the matrix for lecithin, the infrared spectra of the 400 and 600°C residues were virtually identical to those obtained without lecithin (Fig. 5(c) and (d)). However, after heating the lecithin‒NaCl‒JSC Mars-1A mixture at 800°C, new bands appeared that could be assigned to the aluminosilicate sodalite (Na8[AlSiO4]6Cl2) (Fig. 5(c)). A reference spectrum of sodalite is shown in Fig. S6. Sodalite also occurs naturally as a mineral (Deer et al., Reference Deer, Howie and Zussman2013; Hudson Institute of Mineralogy, 2022a). Interestingly, in the absence of lecithin, no new bands were observed after heating to 800°C (Fig. 5(d)). These results were confirmed by X-ray diffraction (Fig. 6). In addition, neither lecithin‒NaCl (Fig. 5(a)) nor lecithin‒JSC Mars-1A (Fig. S4) produced sodalite at 800°C. Thus, all three components together are essential for sodalite formation.
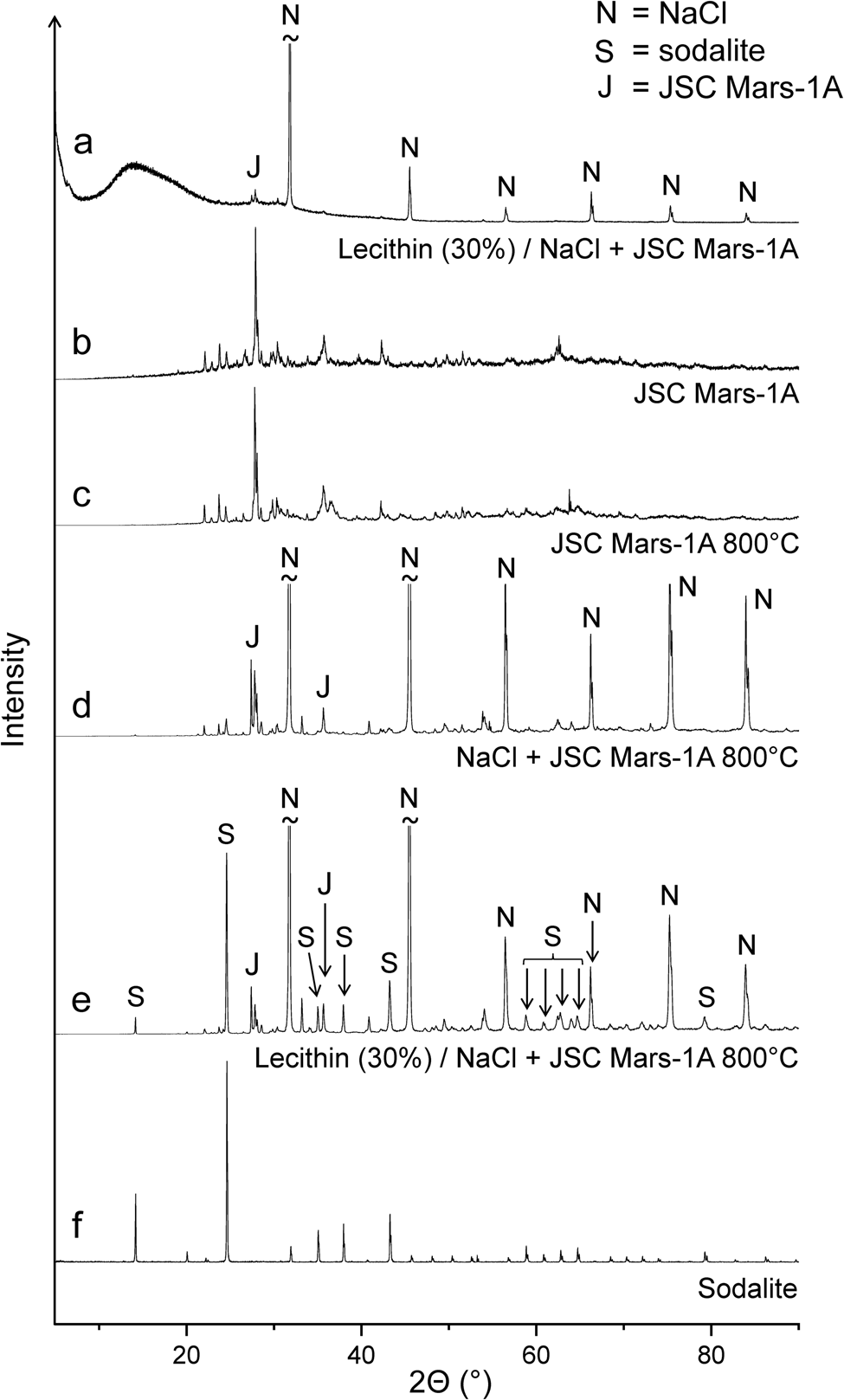
Figure 6. X-ray diffractograms of (a) the lecithin‒NaCl‒JSC Mars-1A mixture, (b) the original JSC Mars-1A, (c) JSC Mars-1A after treatment at 800°C, (d) the NaCl‒JSC Mars-1A mixture after treatment at 800°C, (e) the lecithin‒NaCl‒JSC Mars-1A mixture after treatment at 800°C and (f) sodalite (obtained from the RRUFF database).
Synthetic sodalite can be prepared, for example, by high-temperature solid-state synthesis from the clay mineral kaolinite (Al2Si2O5(OH)4), sodium chloride and sodium hydroxide (Bardez et al., Reference Bardez, Campayo, Rigaud, Chartier and Calvet2008). In our reaction system, JSC Mars-1A provides the aluminosilicate, namely calcium-rich feldspar with the end-member formula CaAl2Si2O8 (Allen et al., Reference Allen, Jager, Morris, Lindstrom, Lindstrom and Lockwood1998), and thus assumes the role of kaolinite. We believe that the strongly basic products that form from lecithin at high temperatures take the place of sodium hydroxide. These products were also responsible for the corrosion of the quartz sample containers at 900°C (see above).
Summary and conclusions
In this study we investigated the effects of temperature on haemin, cytochrome c and lecithin as biosignatures. All experiments were performed under an inert atmosphere of nitrogen. Our main results are as follows:
(1) The characteristic infrared absorptions of the neat samples were still present after heating at 240‒250°C. Thus, the biomolecules were recognisable as such up to this temperature. At higher temperatures the characteristic absorptions disappeared completely.
(2) Haemin showed well-defined decomposition behaviour between ~240 and 260°C where two processes occurred, namely decarboxylation and formation of HCl. The iron porphyrin core, which may serve as an important biosignature (Pleyer et al., Reference Pleyer, Moeller, Fujimori, Fox and Strasdeit2022), remained intact at 260°C. However, it decomposed at higher temperatures.
(3) Solid inorganic products occurred at temperatures of 500°C and above: (i) Haemin formed amorphous carbon and iron oxides at 500°C, and graphite, metallic iron and cementite (Fe3C) at 900°C. It is not clear whether these product mixtures can be used as biosignatures. But it may be noted that biological material can contain more than trace amounts of iron. Dried human red blood cells, for example, are composed of 95‒98% haemoglobin (Kaza et al., Reference Kaza, Ojaghi and Robles2021), corresponding to an iron content of 0.33%. Moreover, loss of volatile products during thermal decomposition will increase the percentage of iron in the residues. (ii) Heating of lecithin at 500°C produced linear long-chain polyphosphates, as indicated by the infrared spectrum of the residue. These anions do not occur in minerals (Pasek, Reference Pasek2020). Therefore, the presence of linear polyphosphates with more than three phosphorus atoms may tentatively be interpreted as a biosignature.
(4) In most cases, minerals did not greatly affect the thermal behaviour of the organic molecules. However, there were two remarkable exceptions for lecithin: (i) Diphosphate (pyrophosphate) was observed as a thermolysis product in sodium chloride between 400 and 700°C. This differs from the behaviour of neat lecithin, where long-chain polyphosphates formed. Whereas numerous phosphate minerals are known, there are only a few rare diphosphate minerals, at least on Earth (see e.g. Hudson Institute of Mineralogy, 2022b). Hence, diphosphate may be considered a potential biosignature. (ii) When a mixture of lecithin, NaCl and JSC Mars-1A was heated at 800°C, NaCl and the feldspar component of the Martian regolith simulant reacted to form the mineral sodalite. This finding is particularly interesting because it demonstrates the formation of a mineral under the influence of (the decomposition products of) biomolecules. Sodalite occurs on Earth in various geological settings, including volcanic ejecta (Hudson Institute of Mineralogy, 2022a). It is therefore probably of limited use as a (secondary) biosignature.
The thermal stability limit of the biomolecules studied here is about 240°C. This temperature may be compared with the surface temperatures that occur near lava flows. For example, on Kilauea volcano, Hawai'i, <175°C were measured at the upper surface of lava levees and 300‒650°C at the inner walls (Pinkerton et al., Reference Pinkerton, James and Jones2002). Clearly, the biomolecules would not survive the high temperatures that occur in close proximity to lava flows. Nevertheless, some of their inorganic decomposition products such as graphite, metallic iron and diphosphate are relatively thermally stable and may serve as potential secondary biosignatures.
We would like to add a few cautionary notes regarding conclusions from studies of the thermal behaviour of chemical biosignatures:
(1) The biomolecules used are selected on the basis of terrestrial biochemistry. However, even when they are involved in basic biochemical functions in all known organisms, these molecules will not necessarily be present in extraterrestrial life. The same biochemical function can often be performed by different molecules. One-electron transfer, for example, is carried out by iron-porphyrin proteins, such as cytochrome c, but also by iron−sulphur and copper proteins (see e.g. Kaim et al., Reference Kaim, Schwederski and Klein2013). It is quite conceivable that potential extraterrestrial biochemistries utilize completely different molecules – not only for one-electron transfer (see e.g. Bains, Reference Bains2004).
(2) Heating experiments have several variables that may influence the outcome, especially when the thermal decomposition processes are complex. These variables include but are not limited to the heating rate, final temperature, annealing time and gas environment (static or flowing, composition). It is virtually impossible to study all combinations of factors that could be relevant. Therefore, the transferability of the results to hot natural environments is limited.
(3) In principle, organic molecules that are formed by thermal decomposition of biomolecules may themselves serve as biosignatures. However, if such molecules are found, for example, on Mars, it may be difficult or even impossible to prove their biogenicity because they could have resulted from prebiotic chemistry in the planet's past, or from meteoritic or cometary delivery. This problem often occurs with potential chemical biosignatures (Fox and Strasdeit, Reference Fox and Strasdeit2017).
Supplementary material
The supplementary material for this article can be found at https://doi.org/10.1017/S1473550423000022
Acknowledgements
We thank Denise Arnold, Miriam Kuzman, Hanna Müller and Juliane Russ for assistance in the laboratory. This work was partly funded by the German Federal Ministry for Education and Research (01PL16003, ‘Humboldt Reloaded’ at the University of Hohenheim).
Conflict of interest
None.