Introduction
Discussion of crinoid origins must include the fundamental but contentious issue of calyx homologies, especially regarding the complexities of plate architecture. Indeed, this matter continues to be a central point of debate among crinoid workers. The calyx harbors a large proportion of characters used in phylogenetic analyses, which magnifies the importance of this issue. Earlier views of crinoid calyx plate homologies predate the widespread recognition that the first crinoids were very different from more crownward forms (see Ausich, Reference Ausich1996, Reference Ausich, Mooi and Telford1998). Attempts to incorporate data based on study of earliest crinoids led to new interpretations of such homologies (see Guensburg and Sprinkle, Reference Guensburg and Sprinkle2003, and section below on Homologies and terminology—a historical perspective). Ausich (Reference Ausich1996, Reference Ausich, Mooi and Telford1998) accepted long-standing homologies based on the work of Carpenter (Reference Carpenter1879), in the process resolving a clade that included both blastozoans (eocrinoids, rhombiferans, etc.) and crinoids to the exclusion of all other echinoderms (Clausen et al., Reference Clausen, Jell, Legrain and Smith2009; Kammer et al., Reference Kammer, Sumrall, Zamora, Ausich and Deline2013; Ausich et al., Reference Ausich, Kammer, Rhenberg and Wright2015a; Sumrall, Reference Sumrall, Zamora and Rábano2015). Those working with new interpretations of earliest crinoids, as well as embryological data that helped to reshape views of homologies of major body wall regions and the construction of feeding structures, discovered that crinoid origins apart from blastozoans, probably from stem group, pentaradiate echinoderms, fit the available data more parsimoniously (Guensburg and Sprinkle, Reference Guensburg and Sprinkle2009; Guensburg et al., Reference Guensburg, Blake, Sprinkle and Mooi2016, Reference Guensburg, Sprinkle, Mooi and Lefebvre2021).
Earlier, expanded terminology for calyx plate homologies was implemented using the hypothesis that a four-circlet calyx (with the late Tremadocian Aethocrinus as the founding exemplar) was inherited, fully organized, from within the subphylum Blastozoa through a Rhopalocystis-like (“eocrinoid”) ancestor (Ausich et al., Reference Ausich, Kammer, Rhenberg and Wright2015a). In contrast to previous works, including the original description (Ubaghs, Reference Ubaghs1963), plates in Rhopalocystis were treated as homologous with those of crinoid calyces, with the pronouncement of radials, basals, infrabasals, and lintels that were then coded accordingly in a phylogenetic analysis (Ausich et al., Reference Ausich, Kammer, Rhenberg and Wright2015a). These interclass homologies were subsequently challenged (Guensburg et al., Reference Guensburg, Blake, Sprinkle and Mooi2016), but no alternative hypothesis as to how well-ordered crinoid calyces evolved was put forward.
Here, we uncover new evidence for the origin of plate systems in the calyx that eschews comparisons based on superficial similarity and prior interpretations of calycinal patterns that were questioned long before the present work (e.g., Mooi et al., Reference Mooi, David, Marchand, David, Guille, Féral and Roux1994; Simms, Reference Simms1994; Mooi and David, Reference Mooi, David, Waters and Maples1997; Guensburg and Sprinkle, Reference Guensburg and Sprinkle2007; among others). The new data are incorporated in a revised character analysis. This information is used to provide a phylogenetic analysis probing crinoid origin, early history, and the evolution of the calyx itself.
Material and methods
Taphonomy and preparation
When warranted, specific comments regarding preservation issues are provided in the figure captions. Specimens of Camptostroma are preserved as molds. All protocrinoid and other later crinoid specimens were preserved in calcite with varying diagenetic effects. No additional preparation was required, except that specimens were coated with ammonium chloride prior to imaging.
Calcite skeletons were exposed by preparation using an air scribe and finishing with fine needles. Overgrowths imbue the calyx with a much more fully covered appearance than would have appeared in life. Individual plates served as centers of calcite overgrowths that can extend outward beyond original plate boundaries, filling interstices including through-going pores, such as epispires. The latter can be recognized because they are often filled with material of a darker color than neighboring plates. In many instances, epispires are evident in topographic lows produced by weathering and etching caused by differential solubility. Much experimentation was required to obtain the most revealing images: uncoated, thin ammonium chloride coating, and immersion in water all provided best results, depending upon the specimen.
A large-field montage image of the Titanocrinus holotype at the Field Museum, Chicago, PE 52720, was prepared at the Illinois Natural History Survey, Urbana. All other images are multi-focus, stacked montages taken at the Field Museum with a Leica DMS 300 digital microscope and accompanying software.
Repository and institutional abbreviation
All figured specimens are deposited in the Field Museum, Chicago, Illinois (FMNH).
Homologies and terminology—a historical perspective
Earlier workers sought to inform echinoderm phylogeny through recognition of class-level homologies. One such proposal was the “calycinal theory” of Lovén (Reference Lovén1866; see Philip, Reference Philip1965, for discussion). As reference to the calyx suggests, early workers recognized crinoids as most plesiomorphic among extant echinoderms because possession of a calyx was itself considered uniquely plesiomorphic. However, no crinoid precursor morphology was recognized; rather, the data were derived from partial developmental and fossil evidence without advantages of the earlier fossil record or modern embryology. The proposed interclass homologies quickly encountered resistance (Sarasin and Sarasin, Reference Sarasin and Sarasin1888; Neumayr, Reference Neumayr1889), and by the turn of the twentieth century, they were practically abandoned. Mortensen (Reference Mortensen1935) later referred to the calycinal theory as the “crinoid phantom.”
In spite of this, crinoid calyx plate homologies, developed within the calycinal theory, remain in use today by many workers (for example, Ausich et al., Reference Ausich, Kammer, Rhenberg and Wright2015a; Wright, Reference Wright2017). Carpenter (Reference Carpenter1879) himself had posited crinoid calyx plate homologies within that theory, emphasizing linkages among crinoids, echinoids, and asteroids. In short, the Carpenter system presents terminology based strictly on radial-interradial orientation beginning with the radials; that is, terminology is assigned according to whether they align or alternate with ambulacra (Fig. 1). An ineluctable consequence of the application of this convention is that different terms for the calyx base circlet result depending on whether there are one or two circlets aboral to radials. Concomitantly, a dichotomy is automatically invoked at the calyx-stem juncture (the so-called “Law of Wachsmuth and Springer”; see Ubaghs, Reference Ubaghs, Moore and Teichert1978). Under this “Law,” basals are confined to the mid-calyx of dicyclic crinoids but are also posited to form the calyx base of monocyclic forms.
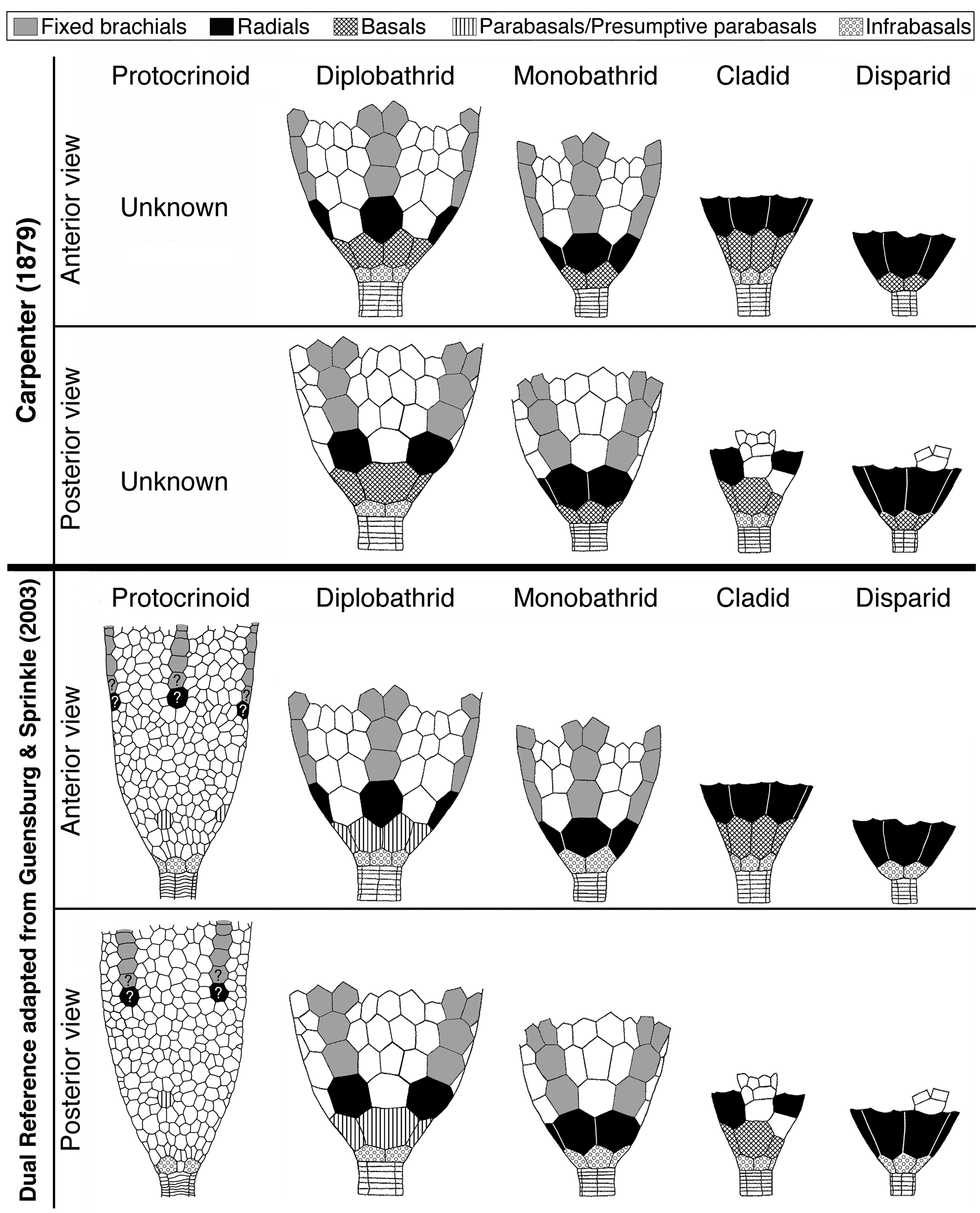
Figure 1. Comparative schematic drawings of five selected groups of crinoids contrasting Carpenter's crinoid calyx plate homologies with those applying the Dual Reference system adopted herein; modified from Guensburg and Sprinkle, Reference Guensburg and Sprinkle2003.
A better understanding of this situation was perhaps unavailable to Carpenter because extant stalked forms and pentameric stems were largely unknown, or at least relatively unstudied, at the time he was working. Monocyclic versus dicyclic constructs were brought into this discussion, but most simply put, circlets below radials (but aligned with them) were termed infrabasals. Accordingly, monocyclic crinoids such as disparids and monobathrid camerates that have a single circlet of plates alternating with radials adoral to them have basals. Diplobathrid camerates and cladid-articulate crinoids have infrabasals, and these align with radials but alternate with basals. However, consistent orientation of the calyx-stem construct points to homology of the calyx base circlet regardless of radial or interradial plate orientation. With newer findings, it has become apparent that newer concepts would also be needed, yet these went largely undeveloped.
A dual reference system first suggested by Guensburg and Sprinkle (Reference Guensburg and Sprinkle2003), and adopted here (Fig. 1), resolves the inconsistencies inherent in the Carpenter system (see section on New information and rationale for a dual reference system, below). The dual reference system redefines existing terminology such as “basals” and “infrabasals,” at the same time seeking to minimize potential confusion in the modified definitions of these terms. Using Guensburg and Sprinkle (Reference Guensburg and Sprinkle2003), “infrabasals” subsume plates that traditionally include both infrabasals and basals (Table 1). Basals include only some of the plates traditionally termed “basals.”
Table 1. Calyx plate homologies under Carpenter's (Reference Carpenter1879) previous concepts, and reformulations of these homologies implemented in this study based on position and development.

*Primordials form in all but a few Paleozoic crinoids but are largely unrecognized in post-Paleozoic forms where they are resorbed very early in development.
In this study, infrabasals are aboralmost in the calyx and always appear oral to the stem, except when secondarily lost through decalcification. They consistently form a border with the stem, whether the calyx is monocyclic or dicyclic. Infrabasals represent the primordial, initial circlet in calyx development, regardless of calyx plate number or arrangement adoral to this system (see section on Character evolution links early radial echinoderms to protocrinoid and later crinoids, below). Although traditional terminology is retained herein, it is important to emphasize just how different this new understanding is from that of Carpenter (Reference Carpenter1879) when it comes to subradial calyx plating, and how these findings paint a fundamentally different picture of the calyx itself.
A differentiated mid-calyx circlet in dicyclic crinoids, termed ‘basals,’ occurs in both diplobathrid camerate and cladid crinoids, but non-congruent evolutionary events among stem crinoids that occur at different relative times in the phylogeny indicate separate origins for these traditionally homologized plates. The protocrinoid Titanocrinus lacks a differentiated mid-calyx circlet (Fig. 2) but does express submerged ambulacra on the tegmen, which is a camerate apomorphy (Guensburg, Reference Guensburg2012; Cole, Reference Cole2017). The submerged condition seen in Titanocrinus and in many other camerate crinoids consists of a tegmen made entirely of extraxial elements, with the axial skeleton hidden entirely beneath. This evidence indicates that a diagnostic feature of the camerate lineage originated prior to more stemward diversification. In contrast, Apektocrinus, a stem taxon coeval with Titanocrinus, possesses basals along with cladid characteristics that include axial morphology, such as exposed hinged cover plates on the tegmen (Guensburg and Sprinkle, Reference Guensburg and Sprinkle2009). These contrasting earliest crinoid anatomies support the conclusion that differentiated mid-calyx circlets of camerate and cladid crinoids are homoplastic. This conclusion is further supported by lack of congruence among other regions in these two groups, particularly in the upper calyx, tegmen, and posterior interray regions. Here, we differentiate the mid-calyx circlets of these two groups; basals for the cladid lineage (includes flexibles and articulates), parabasals for camerates. Terminology applied to crinoid rays follows the findings of Guensburg et al. (Reference Guensburg, Sprinkle, Mooi, Lefebvre, David, Roux and Derstler2020), that of stems follows Ubaghs (Reference Ubaghs, Moore and Teichert1978).
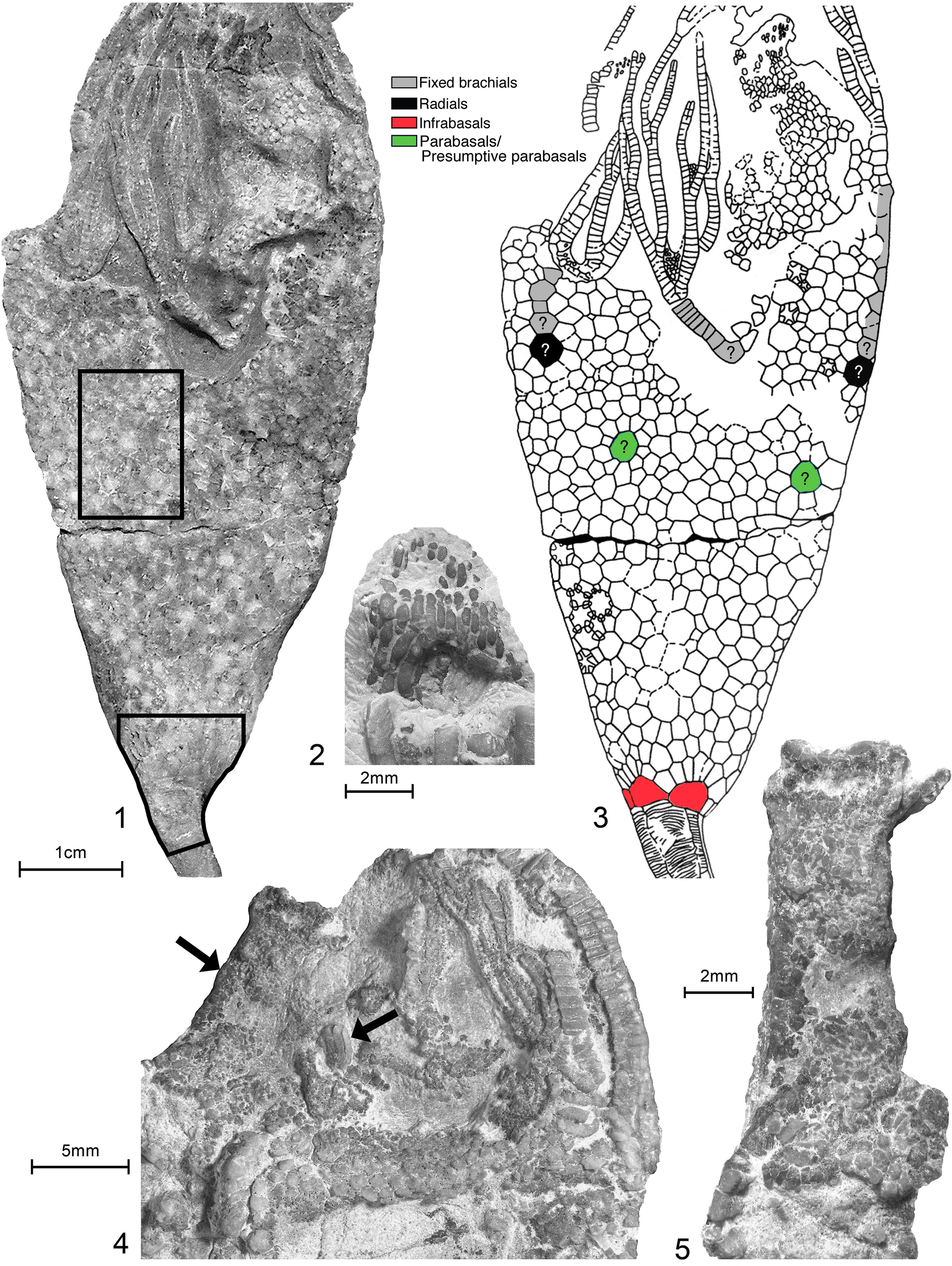
Figure 2. Titanocrinus sumralli Guensburg and Sprinkle, Reference Guensburg and Sprinkle2003. (1, 3) Holotype FMNH PE 52720: (1) D view showing flattened calyx with numerous plates along with proximal stem, tegmen, and partial arms; immersed; enlarged regions in (2) and (3) indicated by enclosures; (2) paratype FMNH PE 52723, uncoated: detail showing stout lath-shaped plates forming anal pyramid, for comparison with plates indicated by right hand arrow in (4) below; (3) line drawing showing plate outlines and plate homologies; (4, 5) paratype FMNH PE 52724, uncoated: (4) tegmen and proximal arm trunks, most of tegmen composed of small stellate plates with central tubercle, 5–6 subcircular respiratory epispires (gaps between plates); tegmen drapes adorally onto arm trunks (primibrachials), where they diminish in size to small, tightly fitted granular ossicles, no indication of cover plates; arrow at left indicates arm trunk, arrow at right points to lath-shaped anal pyramid plates; (5), enlargement of left hand arm trunk, indicated by left arrow in (4), showing many small closely fitted granular plates.
Terminology for blastozoans, first implemented in Guensburg et al. (Reference Guensburg, Sprinkle, Mooi, Lefebvre, David, Roux and Derstler2020), generally follows: Parsley (Reference Parsley and Sprinkle1982) for Eumorphocystis, Paul (Reference Paul1968) for Macrocystella, Ubaghs (Reference Ubaghs and Moore1968) for Rhopalocystis, and Sprinkle (Reference Sprinkle1973) for Cambrian eocrinoids. Following the results of recent phylogenetic and character analyses (Guensburg et al., Reference Guensburg, Blake, Sprinkle and Mooi2016, Reference Guensburg, Sprinkle, Mooi, Lefebvre, David, Roux and Derstler2020, Reference Guensburg, Sprinkle, Mooi and Lefebvre2021), we continue to find strong evidence that specific calycinal plate systems in blastozoans and crinoids are not homologous, leading us to discard terms such as “basals” and “radials” for blastozoans (see Guensburg and Sprinkle, Reference Guensburg and Sprinkle2007; Guensburg et al., Reference Guensburg, Blake, Sprinkle and Mooi2016). Distinctions to this effect are documented in the analysis of characters used in the phylogenetic analysis (Appendix 1).
Character evolution links early radial echinoderms to protocrinoid and later crinoids
Conceptual framework
Understanding the early steps in the evolution of echinoderms, and the deep origins of groups with such distinctive morphologies as crinoids, hinges entirely on decisions regarding ways of conceptualizing morphological characters and, as emphasized in the introduction above, delineating hypotheses of homology. Because different authors have disagreed on their approaches to this complex issue, different possible evolutionary histories have found support (Mooi and David, Reference Mooi, David, Waters and Maples1997; Ausich et al., Reference Ausich, Kammer, Rhenberg and Wright2015a, Wright et al., Reference Wright, Ausich, Cole, Peter and Rhenberg2017; Sheffield and Sumrall, Reference Sheffield and Sumrall2019; Guensburg et al., Reference Guensburg, Sprinkle, Mooi and Lefebvre2021). In describing the application of the extraxial-axial theory (EAT), Mooi and David (Reference Mooi, David, Waters and Maples1997) established relationships among body regions of echinoderm clades. This approach has been suggested to emphasize differences rather than similarities among these taxa (e.g., Ausich, et al., Reference Ausich, Kammer, Rhenberg and Wright2015a; Sheffield and Sumrall, Reference Sheffield and Sumrall2019). Guensburg et al. (Reference Guensburg, Sprinkle, Mooi and Lefebvre2021) responded to this criticism by indicating specific ways in which the EAT provides homology criteria to unify entire regions of echinoderm body structure, resulting in a framework that can unite echinoderm clades despite their disparate body plans (David and Mooi, Reference David and Mooi1996; Mooi and David, Reference Mooi, David, Waters and Maples1997, Reference Mooi and David2008; David et al., Reference David, Lefebvre, Mooi and Parsley2000).
Here, we have attempted to enforce the strongest possible criteria for hypotheses of homology among morphological features; those grounded in classical methodology include conjunction, congruence, and similarity, with relative strengths diminishing in that order. We also recognize that the lack of supporting data other than general similarity can result from homoplasy (Patterson, Reference Patterson1988; Freudenstein, Reference Freudenstein2005). For analyses of extant forms, this is alleviated to a large extent by analyses of development, ontogeny, and gene expression (David and Mooi, Reference David and Mooi1996; Mooi and David, Reference Mooi, David, Waters and Maples1997, Reference Mooi and David2008; Mooi et al., Reference Mooi, David and Wray2005). These concepts are seldom considered in classic treatments of plate systems among fossil forms. Difficulties with superficial similarity are particularly evident for early stemmed echinoderms in which like terminology spills over from group to group even though specialists have long recognized the problematic nature of flawed application of such nomenclature (Mooi and David, Reference Mooi, David, Waters and Maples1997; Guensburg and Sprinkle, Reference Guensburg and Sprinkle2007; Guensburg et al., Reference Guensburg, Blake, Sprinkle and Mooi2016).
Protocrinoid calyces as the plesiomorphic condition of the crinoid calyx
Titanocrinus sumralli Guensburg and Sprinkle, Reference Guensburg and Sprinkle2003, and Glenocrinus globularis Guensburg and Sprinkle, Reference Guensburg and Sprinkle2003 (hereafter Titanocrinus and Glenocrinus, because each is monospecific and can be referred to collectively as protocrinoids) are also among the earliest-known crinoids. These were originally described as stem Crinoidea (Guensburg and Sprinkle, Reference Guensburg and Sprinkle2003) and later, stem Camerata (Guensburg, Reference Guensburg2012; Cole, Reference Cole2017). The principal criterion used in these phylogenetic findings is presence of submerged ambulacra, arguably a camerate apomorphy also present in protocrinoids (Guensburg, Reference Guensburg2012) (Fig. 2). A detailed reanalysis of the protocrinoid calyx, expanded from the original and emended descriptions (Guensburg and Sprinkle, Reference Guensburg and Sprinkle2003, Reference Guensburg and Sprinkle2016), follows.
Protocrinoid calycinal morphologies are unique among crinoids: large, thick, primary plates are surrounded by small, irregular secondary plates, generally with long axes arrayed in rosette patterns throughout the calyx (Figs. 3.2, 3.4, 4.1–4.3, 5.2), all set in otherwise disorganized fields (Sprinkle and Guensburg, Reference Sprinkle, Guensburg and Barker2001; Guensburg and Sprinkle, Reference Guensburg and Sprinkle2003; Ausich et al., Reference Ausich, Kammer, Rhenberg and Wright2015a). Rosettes increase in size aborally. Secondaries possess ovate respiratory pores (epispires). Other regions of the calyx lack any apparent ordered pattern and still others, more adorally in the calyx, suggest organization in poorly organized columns (Fig. 4.4). Up to 19 smaller plates per primary plate occur in Titanocrinus. Mid-calyx primaries are subcircular in outline (Fig. 3.2, 3.4). In general, secondaries abutting primaries are the smallest of their type. Viewed from the internal body wall, each secondary expresses a main ridge or keel running longitudinally with a crossing brace articulating to juxtaposed secondaries; deep notches extending toward the outer thecal wall are present between ridges (Fig. 3.3).
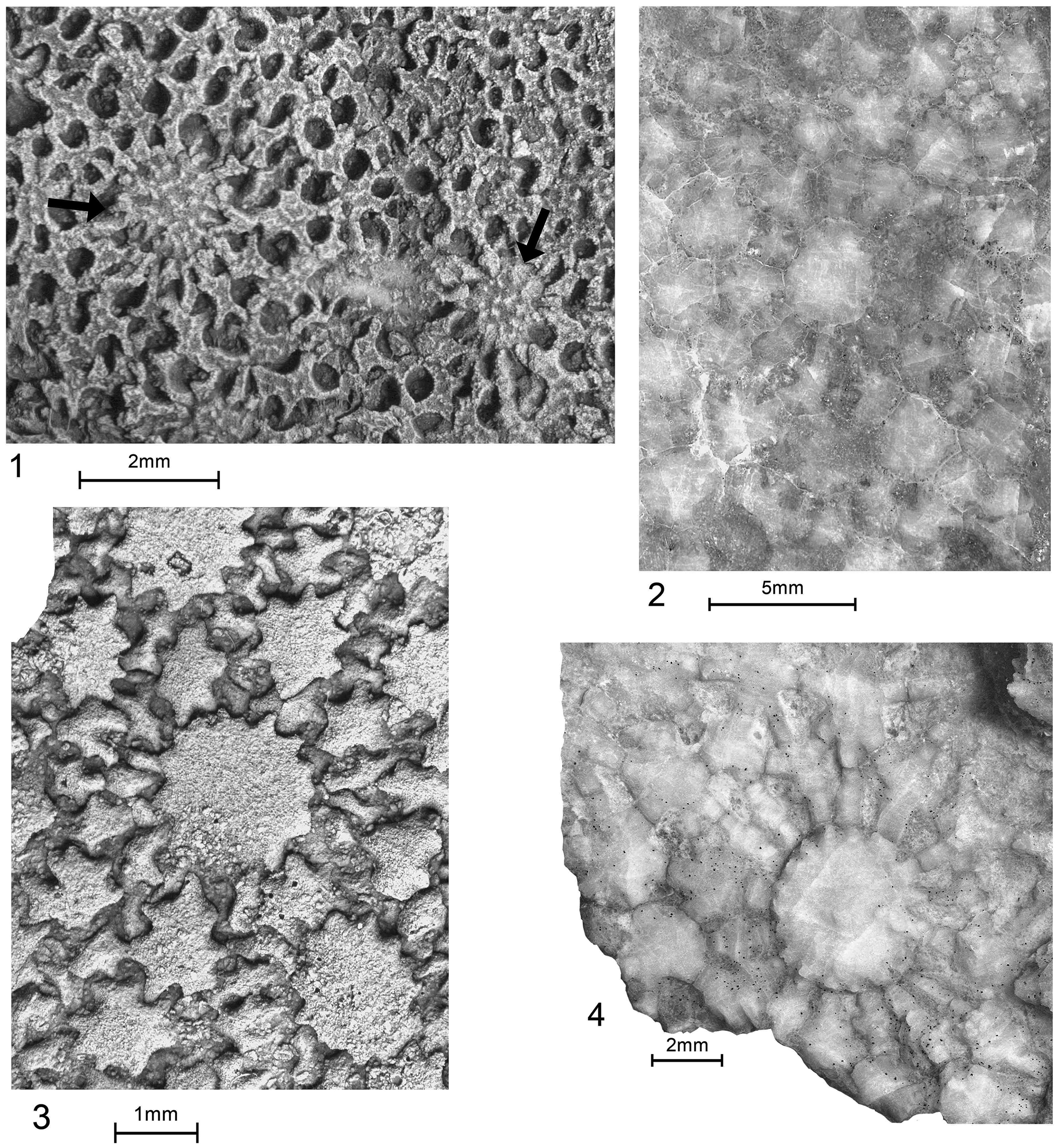
Figure 3. Comparative extraxial plating of Camptostroma roddyi Ruedemann, Reference Ruedemann1933, and Titanocrinus sumralli Guensburg and Sprinkle, Reference Guensburg and Sprinkle2003. (1, 3) Camptostroma roddyi, topotypes FMNH PE 93326 and FMNH PE 93327, respectively; both coated latex casts; (1) middle CD interambulacrum, exterior with two large primaries indicated by arrows, each surrounded by field of secondaries forming reticulate network pierced by many large, ovate epispires; (3) distal interambulacrum, interior with stellate primary at center contacting small secondaries, larger secondaries beyond; (2, 4) Titanocrinus sumralli, holotype FMNH PE 52720 and paratype FMNH PE 52724, respectively; both with extensive calcitic overgrowths, original epispires indicated by darker infilling; (2) exterior mid-calyx region indicated in Figure 1.1, ~2 cm aboral to the calyx-tegmen juncture, primary plates at center and surrounding secondary rosette, adjoining secondaries with large dark calcitic gaps indicating epispire locations; larger secondaries beyond those contacting primary; (4) interior mid-calyx region ~2 cm aboral to calyx-tegmen juncture, primary, surrounding rosette of secondaries, gaps visible between adjacent elements mark epispires, larger secondaries beyond.
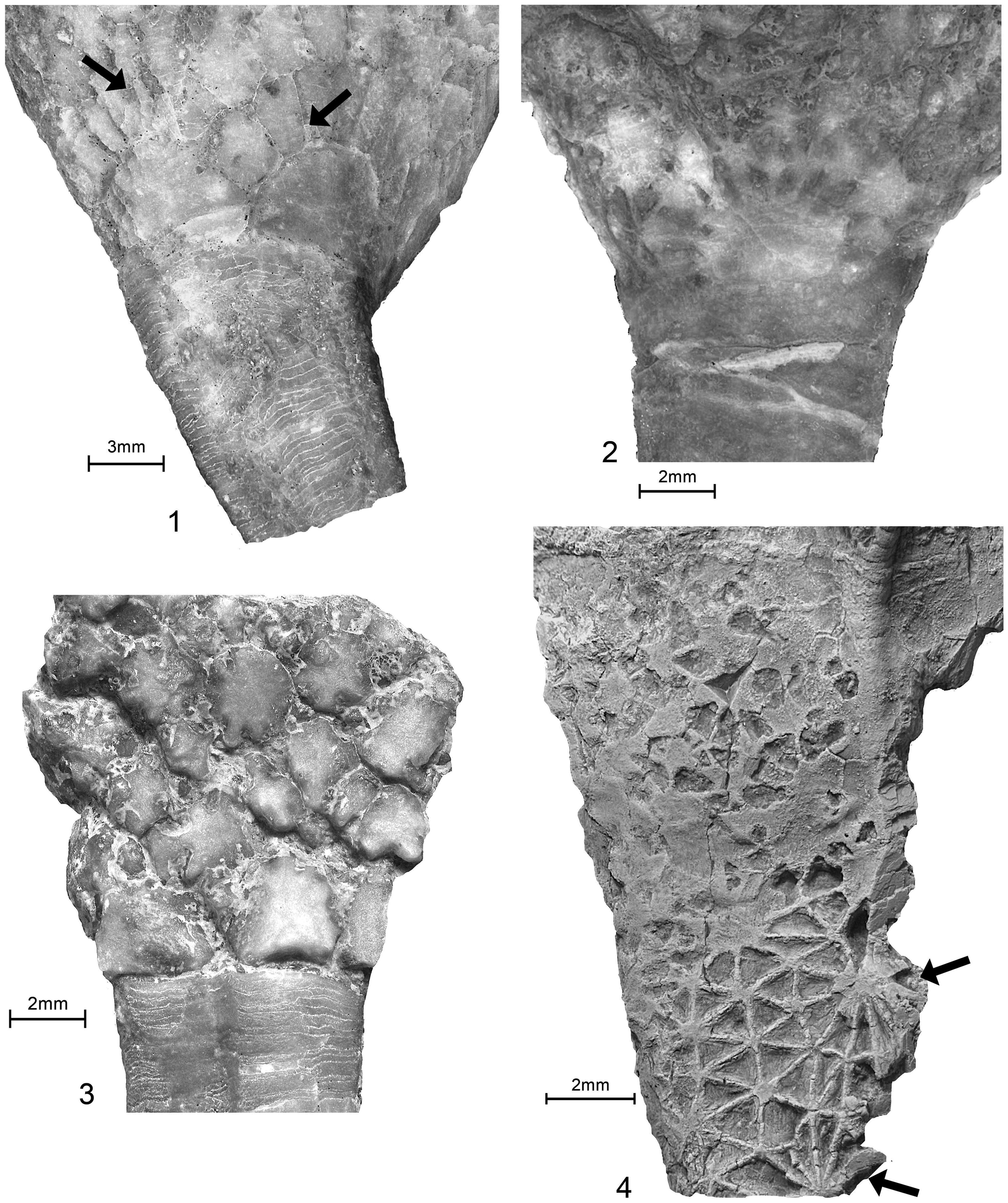
Figure 4. Titanocrinus sumralli Guensburg and Sprinkle, Reference Guensburg and Sprinkle2003. (1) Holotype FMNH PE 52720, boundaries indicated in Figure 2.1, aboralmost calyx and proximal stem, immersed, naturally etched, with extensive calcitic overgrowths; semicircular infrabasals visible at left and center right, each surrounded by hemi-rosette of secondaries with faint epispires, two examples indicated by arrows; stem-calyx juncture irregular, meres alternating with infrabasals; (2, 4) paratype FMNH PE 52721; (2) lower calyx and proximal stem, extensive calcitic overgrowths, immersed; semicirclular D oriented infrabasal at center, surrounded by secondary hemi-rosette with dark calcitic infillings in large epispires extending to stem on BC and CD sides, stem-calyx juncture an irregular surface; (4) upper calyx, coated, damaged B ray on right, with series of five fixed brachials, the initial four of which are large, calyx-like, B ray primary/radial below, aligned with fixed B ray plates above indicated by upper right arrow; C ray folded just out of view at left; BC interray with relatively uniform secondaries, except one partial primary indicated by arrow below; (3) FMNH PE 52726, aboralmost calyx, flattened and slightly disarticulated, proximal stem; infrabasals alternate with stem meres, meres highly irregular, scalloped calyx plate margins mark epispire locations.
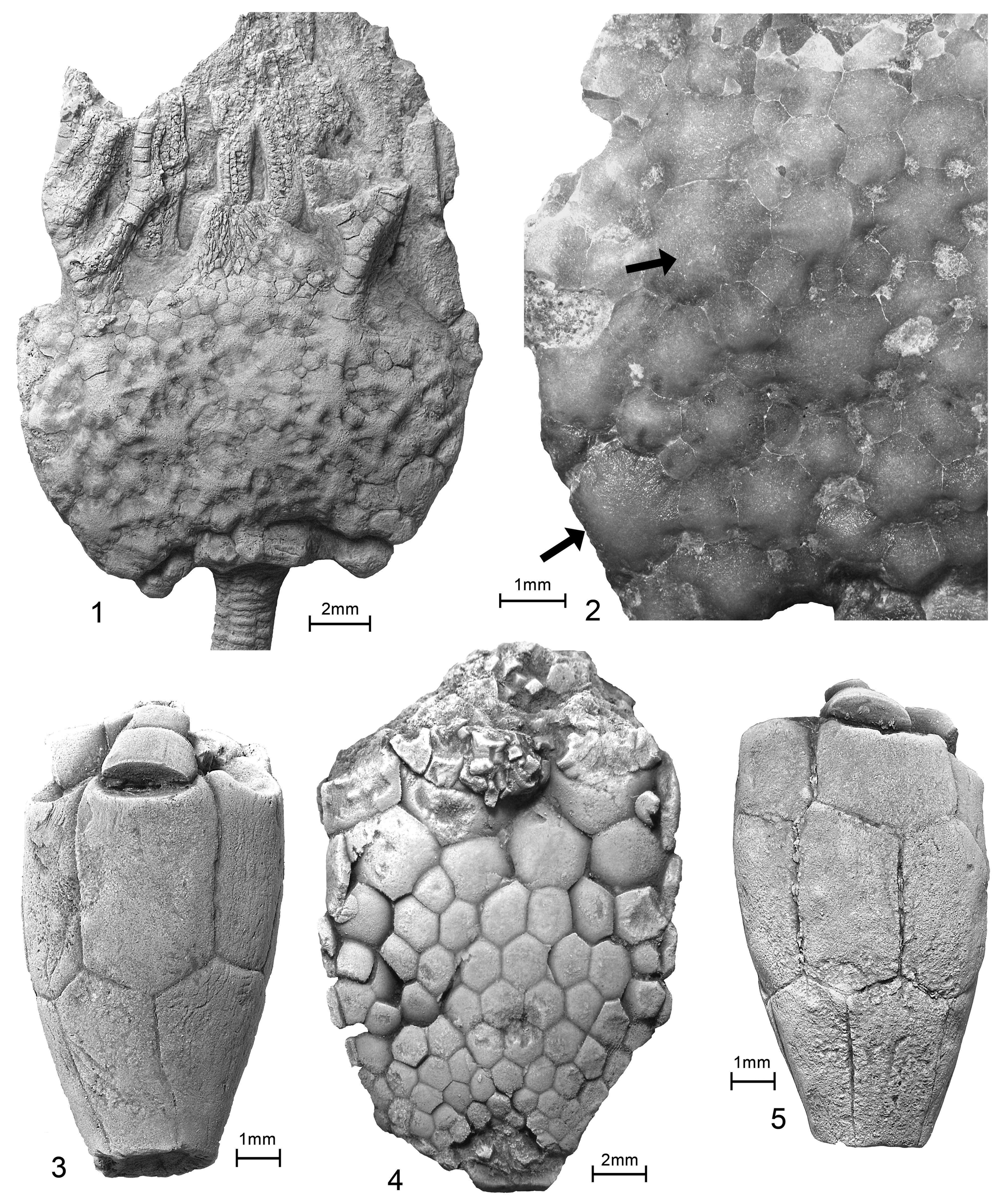
Figure 5. (1, 2) Glenocrinus globularis Guensburg and Sprinkle, Reference Guensburg and Sprinkle2003; holotype FMNH PE 52733. (1) Partial crown and proximal stem, CD interray view, coated; (2) enlargement, left side of calyx, apparent irregular epispires with dark calcitic infilling, uncoated; primary plate homologies as follows: upper arrow indicates a radial, lower arrow points to potential DE parabasal, these primaries surrounded by rosettes. (3, 5) Bactrocrinites fieldi (Springer and Slocum, Reference Springer and Slocum1906) (= Hypsocrinus fieldi Springer and Slocum, Reference Springer and Slocum1906), FMNH PE 7979; referred specimen, coated; (3) E and (5) C views, respectively, showing both monocyclic and dicyclic patterns on opposite sides of the same calyx: monocyclic calyx is E ray view with infrabasals and radials; and dicyclic calyx is C ray view with infrabasals, basals, and radials. (4) Acrocrinus shumardi Yandell, Reference Yandell1855, FMNH UC 14403, flattened calyx, coated; mid-calyx with many small plates arranged in graduated ranks, not primary-secondary pattern or epispires as developed in protocrinoids.
Certain primary elements of the calyx in Titanocrinus are positioned in accordance with those seen in more strongly ordered crinoid calyces. These include a differentiated calyx base circlet and initial ray plates, incipient radials, and infrabasals. Of these, only calyx base plates are distinguishable from other primaries in terms of size and shape. Undifferentiated primaries occur at the proximal bases of rays, with differentiated fixed brachials continuing distally. Primaries at the base of the calyx are large, roughly semi-circular, and surrounded by secondaries in a fan shape, these collectively forming hemi-rosettes (Fig. 2.1–2.3). These articulate with adjacent primaries in the anterior region, but not in the posterior region, where secondaries extend to the stem, creating intervening gaps (Fig. 4.1, 4.2) (Guensburg and Sprinkle, Reference Guensburg and Sprinkle2003). One or two gaps occur in Titanocrinus. These gaps and accompanying gap plates are known elsewhere only in other Tremadocian taxa, including Eknomocrinus wahwahensis Guensburg and Sprinkle, Reference Guensburg and Sprinkle2003, and Glenocrinus globularis Guensburg and Sprinkle, Reference Guensburg and Sprinkle2003, and rarely or intermittently in the Late Ordovician Anomalocrinus incurvus (Meek and Worthen, Reference Meek and Worthen1865). Position and number of mid-calyx primaries in Titanocrinus are unclear, although these appear to be interradial in the holotype FMNH PE 52720 and paratype FMNH PE 52721. Another protocrinoid, Glenocrinus, expresses mid-calyx morphology (Fig. 5.1, 5.2) intermediate between Titanocrinus and well-ordered early diplobathrid camerates such as Proexenocrinus Strimple and McGinnis, Reference Strimple and McGinnis1972. Large primary plates occupying interradial positions in the mid-calyx represent candidate basals. These are surrounded by smaller intervening secondaries recalling the Titanocrinus pattern. Dark spots along plate margins in the aboral region of the Glenocrinus calyx suggest vestigial epispires in this taxon (Fig. 5.2).
The primary-secondary thecal plate pattern seen in protocrinoids is found in one of the earliest radial echinoderms (early Cambrian, stage 3-4 boundary, series 2), Camptostroma roddyi Ruedemann, Reference Ruedemann1933. Note that Paul and Smith (Reference Paul and Smith1984) used the term “stellate plates” for what we refer to as primaries here. Camptostroma also expresses another similarity, epispires, comparable to those of protocrinoids (compare Fig. 3.1 to 3.2, and Fig. 3.3 to 3.4). Camptostroma is a biscuit-shaped edrioasteroid-like taxon, likely with ambulacra extending off the theca to form short, arm-like extensions (Durham, Reference Durham1966). Much important Camptostroma material that can shed light on these extensions has been examined but remains undescribed. The status of the arm-like structures is considered tentative, although new evidence (Derstler et al., Reference Derstler, Guensburg, Blake and Sprinkle2018) seems to support Durham's (Reference Durham1966) interpretation. Nevertheless, the reconstruction of Camptostroma as an edrioasteroid-like taxon is considered accurate (Durham, Reference Durham1966; Paul and Smith, Reference Paul and Smith1984).
Given the phylogenetic distribution of these morphologies, we interpret the protocrinoid expressions to include deep-seated apomorphies of radial echinoderms, plesiomorphic at the level of blastozoans and crinoids. Even the early Cambrian pentaradial echinoderm, Sprinkleglobus, shows many of these expressions (Zhao et al., Reference Zhao, Rahman, Zamora, Chen and Cong2022). Details further support this conclusion, but not the inference that primary-secondary plating represents a synapomorphy exclusive to blastozoans and crinoids (e.g., Ausich et al., Reference Ausich, Kammer, Rhenberg and Wright2015a). Gogiids and diploporites express primary-secondary thecal plate patterns (e.g., the Cambrian Gogia palmeri Sprinkle, Reference Sprinkle1973, Ordovician Eumorphocystis multiporata Branson and Peck, Reference Branson and Peck1940, and Silurian Holocystites alternatus Hall, Reference Hall1864). Of these, only G. palmeri has epispires comparable to those in Camptostroma. However, this and all blastozoans have feeding appendages that lack left and right somatocoels, a character complex present in all crinoids (Guensburg et al., Reference Guensburg, Blake, Sprinkle and Mooi2016, Reference Guensburg, Sprinkle, Mooi and Lefebvre2021).
This is not merely a preferred interpretation based on what can and cannot be seen in fossils lacking soft tissues. Adult anatomy and developmental morphology illustrate that the plesiomorphic topological order through the ambulacral region of both fossil and extant forms proceeds from the external part of the animal through the hydrocoel (water vascular system), which in turn is underlain by the axial region (i.e., floor plates). This constitutes the body wall of the ambulacral region, with the left and right somatocoels internal to that. The most parsimonious interpretation for blastozoans is to recognize that brachioles are apomorphic, free ambulacra (Paul and Smith, Reference Paul and Smith1984; David et al., Reference David, Lefebvre, Mooi and Parsley2000; Mooi et al., Reference Mooi, David and Wray2005), and that these lack extensions of the theca that could include anatomically internal coelomic extensions of the somatocoels. This is why there is no connectivity between the thecal cavity and blastozoan feeding appendages, including pseudo-arms (Guensburg et al., Reference Guensburg, Sprinkle, Mooi and Lefebvre2021). This anatomy is clearly very different from that seen in all crinoids, extant and extinct, in which the somatocoels send extensions from the theca out into the arms, topologically internal to the axial series.
Interestingly, primary-secondary extraxial morphology even occurs in cornute stylophorans, such as on the supracentral and right infracentral areas of Chauvelicystis spinosa Ubaghs, Reference Ubaghs1970 (see Ubaghs, Reference Ubaghs, Courtessole, Marek, Pillet, Ubaghs and Vizcaino1983, plate VIII, fig 4a, c) and in certain scotiaecystids such as Thoralicarpus jefferiesi (Gil Cid et al., Reference Gil Cid, Domínguez, Silván Pobes and Escribano Ródenas1996) (Lefebvre et al., Reference Lefebvre, Nohejlová, Kašička and Zicha2022).
Transitional morphologies support primary-secondary plating as plesiomorphic among crinoids
Although much of the calyx is not preserved in the single known specimen of the Tremadocian stem cladid Apektocrinus ubaghsi, one primary-secondary rosette occurs high in the CD interradius (Guensburg and Sprinkle, Reference Guensburg and Sprinkle2009, fig. 5.1). Primary-secondary plating with epispires occurs in the late Floian taxon Habrotecrinus ibexensis Guensburg and Sprinkle, Reference Guensburg and Sprinkle2003 (Fig. 6.1). The late Floian Adelphicrinus fortuitus Guensburg and Sprinkle, Reference Guensburg and Sprinkle2003, has epispires (Fig. 6.2). A primary-secondary pattern is uncommon in more crownward crinoids but where we have encountered it, it is stratigraphically early. The Middle Ordovician Deocrinus asperatus Hudson, Reference Hudson1907 (Fig. 7) possesses primary-secondary rosettes, as do the Late Ordovician species Diabolocrinus perplexus Wachsmuth and Springer, Reference Wachsmuth and Springer1897 (Ubaghs, Reference Ubaghs, Moore and Teichert1978, fig. 234) and Paradiabolocrinus stellatus Kolata, Reference Kolata and Sprinkle1982. None of these taxa has accompanying epispires or other respiratory structures. Small secondary (accessory) plates are associated with pleated respiratory structures in the Middle Ordovician cladid Perittocrinus radiatus Jaekel, Reference Jaekel1902, but in this case, the presumed respiratory structures are pleated stereom (goniospires) that are unlike epispires (Fig. 8).
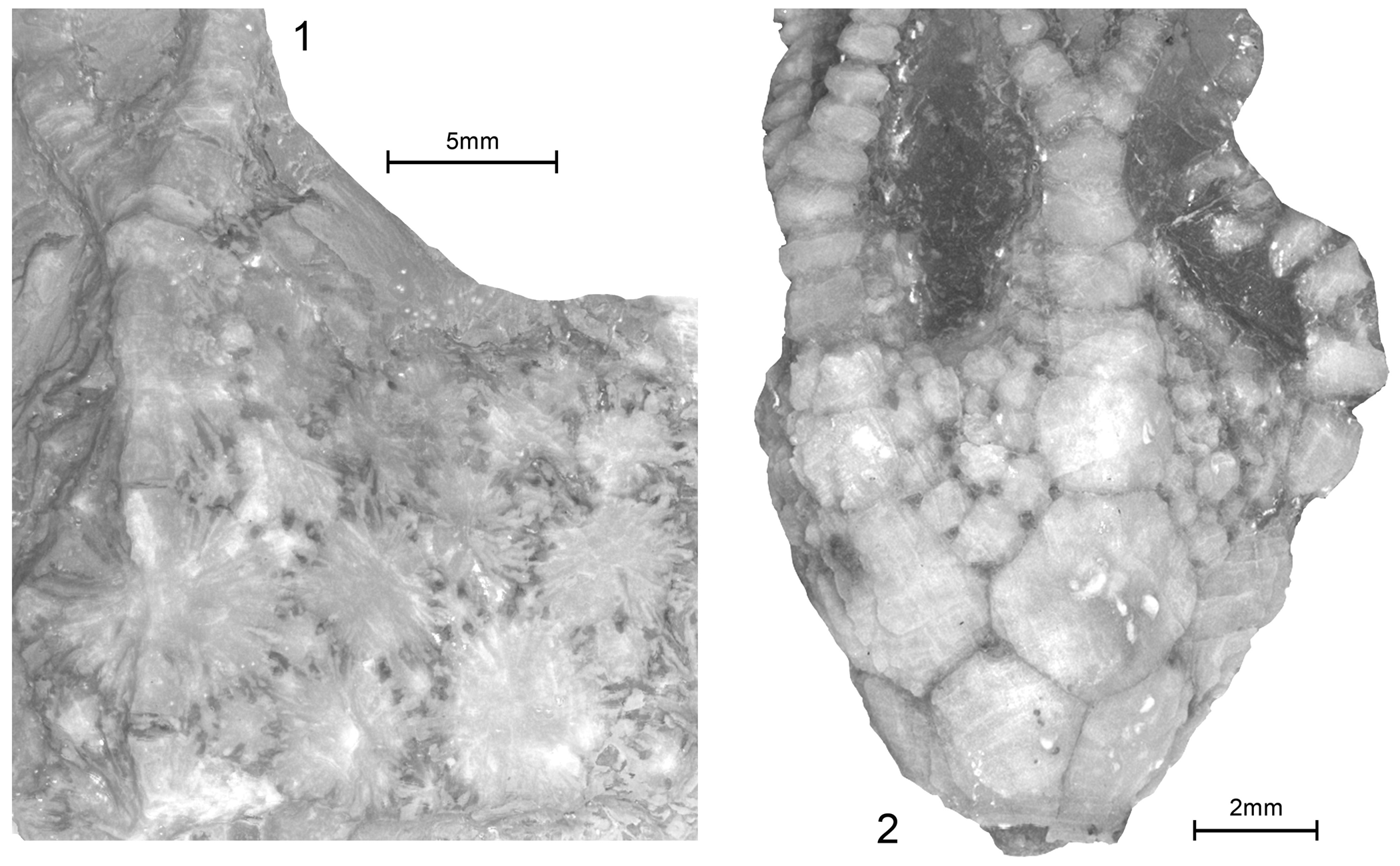
Figure 6. (1) Calyx plating of Habrotecrinus ibexensis Guensburg and Sprinkle, Reference Guensburg and Sprinkle2003; holotype FMNH PE 52740, immersed; ray at left showing three fixed brachials, partial plate below; interray at right with large primaries and small intercalated secondaries, darker epispires along plate margins; numerous small radiating ridges, larger, heavier ray ridges. (2) Adelphicrinus fortuitus, holotype FMNH PE 52739, immersed; calyx and proximal arms, interrays with several small plates, epispires at plate triple junctures, possible small epispires at adoral apices of infrabasals.

Figure 7. Calyx plating of Deocrinus asperatus (Billings, Reference Billings1859), refigured, from Hudson (Reference Hudson1907, pl. 5), showing posterior interray (at 12 o'clock) with two large, stacked plates forming possible short anitaxis, anterior interradii each with large central primary and surrounding secondaries; aboralmost calyx with infrabasals beneath stem facet, and large parabasals beyond below radials/rays, much enlarged (specimen calyx ~12.5 mm in diameter); by permission, New York State Museum.

Figure 8. Plate diagram of Perittocrinus radiatus Jaekel, Reference Jaekel1902, relabeled to conform to interpretations herein; original plate diagram from Ubaghs (Reference Ubaghs1971). Largest radial ~10 mm across.
Other earliest crinoids display radial-interradial mid-calyx symmetry in the form of basals or a lack of mid-calyx plates. However, for the most part, these express some differences from typical, regular, orderly patterns. This is seen, for example, in the calyx of the stem group cladid Apektocrinus (see Guensburg and Sprinkle, Reference Guensburg and Sprinkle2009), below the A radial of Aethocrinus (see Ubaghs, Reference Ubaghs1969), and in the interrays of the stem disparid Alphacrinus (Guensburg et al., Reference Guensburg, Blake, Sprinkle and Mooi2016). Stratigraphic distribution of early crinoids suggests that development of regular alternating circlets post-dates the diversification of crinoids into camerate and cladid clades. Disparids, lacking mid-calyx elements, seem to have adopted regularity early in their history, but even here, the earliest examples, Alphacrinus and Athenacrinus (Guensburg, Reference Guensburg2010; Guensburg et al., Reference Guensburg, Sprinkle, Mooi, Lefebvre, David, Roux and Derstler2020), express crinoid plesiomorphies, including among interradial plating and a wide, multi-plated posterior interray.
New information and rationale for a dual reference system
Adoption of a dual reference system requires that the mid-calyx be capable of variable expressions, and of adopting orientations according to whatever plate configurations lie adoral to it. The protocrinoid mid-calyx, with its impressively many-plated, only partly ordered construction, is a manifestation of this plasticity (Guensburg and Sprinkle, Reference Guensburg and Sprinkle2003). Stated another way, we postulate that, plesiomorphically in crinoids, there are no conjoined calyx circlets in fixed alternating orientations. With strong support that this type of calyx is plesiomorphic within the Crinoidea, it follows that adjustment to a regular organization of fewer plates occurred through suppression of secondary plate formation during ontogeny. Camerates continued to express extensive interradial plating much later in their history, but in this instance, such expressions encompass the adoral calyx region at, or oral to (i.e., above) radials.
Other evidence supports this view of evolutionary change in earliest crinoids. First, an analysis finds precise correspondence of the appearance of “infrabasals” and the embryological origin of the chambered organ (Engle, Reference Engle2012). The appearance of an infrabasal circlet correlates with ontogenetic events that give rise to nervous system and coelomic extensions within the stem. This finding, never cited in previous paleontological work that relies on elements of the Carpenter system, indicates infrabasals represent primordial plates (i.e., the “true” primordial cup base circlet). This finding is further corroborated by positional criteria derived from studies of early ontogeny of stalked crinoids (Amemiya et al., Reference Amemiya, Omori, Tsurugaya, Hibino, Yamaguchi, Kuraishi, Kiyomoto and Minokawa2016, fig. 2I).
Second, other evidence from late Paleozoic crinoids supports the concept of lability in expression of mid-calyx architecture. Examples include acrocrinid camerates in which large numbers of plates were added to a monocyclic condition already exhibiting simplification due to a loss of secondaries (see Moore and Laudon, Reference Moore and Laudon1943). Another example is the lineage including Atelestocrinus, Bactrocrinites, Belemnocrinus, Thalamocrinus, and others, in which dicyclic forms transition to monocyclic calyces (MacIntosh, Reference McIntosh1979; Gahn, Reference Gahn2022). In these cases, the calyx base circlet and radials adjust, rotating through a 36° arc in order to accomplish the transition (Fig. 5.3, 5.5).
Third, data developed from our own and other previous work reveal other early crinoids with irregular mid-calyces (e.g., Belanskicrinus westoni Strimple and Levorson, Reference Strimple and Levorson1969; Tryssocrinus endotomitus Guensburg, Reference Guensburg1984; Quechuacrinus ticsa Guensburg and Waisfeld, Reference Guensburg and Waisfeld2015; and Reteocrinus varabilicaulis Guensburg, Reference Guensburg1984).
Phylogenetic analysis
Basis of phylogenetic analysis
The present analysis, with 38 taxa (Table 2), is expanded from Guensburg et al. (Reference Guensburg, Sprinkle, Mooi, Lefebvre, David, Roux and Derstler2020) and includes the new findings regarding crinoid calyx plate homologies reported here. Both maximum parsimony and Bayesian analyses were performed. All taxa from the earlier analysis, with one exception, are included in the present work. The exception, Gogia palmeri, was substituted for G. kitchnerensis Sprinkle, Reference Sprinkle1973, because the former taxon's morphology includes primary and secondary thecal plating similar to that of early crinoids. This substitution is a more conservative test of homology schemes. The primary goal has been and continues to be a test of crinoid ancestry itself, and diverse ingroup forms, both stem-radiate and blastozoan taxa, are included, along with a preponderance of early crinoids. All Early Ordovician crinoid genera were surveyed as well as those referenced above in the text. Stromatocystites pentangularis, a Cambrian stem radial echinoderm considered to exemplify early pentaradial organization, serves as the outgroup.
Table 2. List of taxa used in analysis.

The new understanding of calyx plate homologies presented here invites further analysis of early crinoid phylogeny, a second but important goal of this paper. To explore this adequately, it is critical to appreciate that camerate-, cladid-, and disparid-like representatives occur early, within the Early Ordovician, during the middle Tremadocian through Floian stages, 482–470 mya (Walker and Geissman, Reference Walker and Geissman2022). For example, the posited middle Tremadocian stem camerate or camerate sister group taxon, Titanocrinus, expresses just a single exclusive camerate apomorphy, submerged ambulacra. It is also important to recognize that the late Floian Proexenocrinus expresses typical diplobathrid camerate characters including, among others, pinnulate arms and rays bifurcating within the calyx. Similar introductions of evolutionary novelty can be seen in earliest cladids and disparids (Guensburg, Reference Guensburg2012). Therefore, morphologies that evolved to characterize early and middle Paleozoic crinoids had largely appeared by the close of the Early Ordovician. This finding guided our design of analyses targeting the origin and early diversification of crinoids.
Seventeen of the 20 Early Ordovician genera were coded in this analysis. Type specimens of Inyocrinus strimplei Ausich, Reference Ausich1986, Pogonipocrinus antiquus (Kelly and Ausich, Reference Kelly and Ausich1979), and Habrotecrinus ibexensis Guensburg and Sprinkle, Reference Guensburg and Sprinkle2003, were examined and eliminated from consideration due to excessive lack of data for characters employed in our matrix.
The constrained temporal emphasis on the majority of taxa mitigates, but of course does not eliminate, possible homoplasy. Therefore, seven other crinoids were added to the Early Ordovician taxon list. These include Late Ordovician cladids Carabocrinus and Hybocrinus, taxa proposed to link blastozoans and crinoids based on their similar oral regions (Kammer et al., Reference Kammer, Sumrall, Zamora, Ausich and Deline2013; Sumrall, Reference Sumrall, Zamora and Rábano2015). The Late Ordovician camerate Gaurocrinus was included in a previous phylogenetic analysis placing the blastozoan Eumorphocystis as the sister taxon to the Crinoidea (Sheffield and Sumrall, Reference Sheffield and Sumrall2019), so it is included here to test this finding. Two Middle Ordovician taxa, the camerate Deocrinus and cladid Perittocrinus, are considered to retain vestigial primary-secondary plating similar to that of protocrinoids, though without epispires (compare Figs. 2 and 3 with Figs. 6 and 7). The camerate Trichinocrinus and disparid Schaldichocrinus, both from the Middle Ordovician, were included because of their early stratigraphic occurrence and relatively complete datasets.
Characters used in phylogenetic analyses of earliest crinoids
We provide a detailed character analysis (Appendix 1) that includes descriptions of each of the features coded into the matrix (Appendix 2). The development of this character suite results, in part, from previous analyses (e.g., Guensburg et al., Reference Guensburg, Sprinkle, Mooi, Lefebvre, David, Roux and Derstler2020), but also relies on observations made from protocrinoids directly relevant to the determination of homologies discussed above. These forms have not been used consistently in previous analyses seeking to place crinoids in a broader evolutionary context, perhaps in part because of misunderstandings concerning their significance. We hope to ameliorate this situation, especially because protocrinoids generally pose problems for hypotheses that rely on derivation of crinoids from within the blastozoans (see Guensburg et al., Reference Guensburg, Sprinkle, Mooi, Lefebvre, David, Roux and Derstler2020, Reference Guensburg, Sprinkle, Mooi and Lefebvre2021, for reviews of the contentious nature of the issues involved). It is important to recognize that all characters used here, and that code exclusively within the crinoids, occur within Early Ordovician taxa (i.e., they can be accepted as deep homologies among the earliest Crinoidea).
These 44 characters were assembled for scoring using Mesquite Version 3.2 (build 801), and the nexus file run on PAUP* 4.0a (build 167) for Macintosh (Swofford, Reference Swofford2003). All characters were unordered and unweighted. A heuristic analysis was run using 1,000 random replicates holding ten trees at each step with tree-bisection-reconnection (TBR) branch-swapping. Strict and 50% majority rule consensus trees were computed, and a bootstrap analysis of 1,000 replicates was run using a fast heuristic search.
Parsimony analyses were repeated using new technology tree searches in TNT v. 1.5 (Goloboff and Catalano, Reference Goloboff and Catalano2016), and continued until the same tree length was independently encountered in 100 replicates. The dataset was also analyzed under a Bayesian framework in MrBayes v. 3.2.7a (Ronquist et al., Reference Ronquist, Teslenko, van der Mark, Ayres, Darling, Höhna, Larget, Liu, Suchard and Huelsenbeck2012). Character evolution was modeled using an Mkpars + Г model (Lewis, Reference Lewis2001), accounting for variability in evolutionary rates as well as biases introduced by coding only parsimony-informative characters. Four runs of four chains each were continued for ~12.6 million generations, at which point an average standard deviation of split frequencies of 0.005 was reached. The initial 50% of samples were discarded as burn-in. Convergence and stationarity were confirmed using Tracer v. 1.7.1 (Rambaut et al., Reference Rambaut, Drummond, Xie, Baele and Suchard2018); all parameters attained effective sample sizes >4,000 and potential scale reduction factors of 1.0. Posterior topologies were summarized using a majority rule consensus. Resulting trees were rooted using Stromatocystites as the outgroup.
Results
Maximum parsimony analysis recovered a single island of 8,628 trees with length 117, consistency index of 0.453, retention index of 0.772, rescaled consistency index of 0.350, and homoplasy index of 0.547. Results from the TNT analysis are virtually identical except for a slightly higher number of equally parsimonious trees (8,640). Strict consensus and majority rule consensus trees are shown in Figure 9.

Figure 9. Results from the parsimony analyses of matrix in Appendix 2. (1) Strict consensus of 8,628 shortest trees found using PAUP*. This tree is identical in topology to the strict consensus recovered using TNT; (2) 50% majority rule tree found with PAUP* analysis, frequency of nodes shown above lines (only for frequencies less than 50%), bootstrap values for majority-rule consensus of 8,628 trees shown in parentheses below the lines (only for values greater than 50%).
The majority rule consensus of the Bayesian analyses revealed a topology consistent with that of the parsimony analyses (Fig. 10), although with lower precision, notably in not finding an exclusive relationship of Camptostroma with the crinoids (see below). In addition, resolution among more crownward crinoid taxa was also much lower than in the parsimony analyses, although protocrinoids, Apektocrinus, and Eknomocrinus, all branched from the earliest node among crinoids with a high posterior probability.

Figure 10. Results of the Bayesian analysis (see Klopfstein and Spasojevic, Reference Klopfstein and Spasojevic2019, for RoguePlot methodology). Posterior probabilities with values <1.00 are indicated by numbers above the lines.
Results continue to show little or no evidence to link blastozoans and crinoids to the exclusion of other early echinoderms. For example, in the Bayesian analysis, the posterior probability that the crinoids and blastozoans share exclusive common ancestry was actually zero. In conjunction with all other findings of the parsimony analyses, this further supports separate derivation of these two major clades from ancestry deeper in the Echinodermata as a whole, most likely among early pentaradial forms.
With our conservative coding, the position of Camptostroma in the Bayesian analysis remains slightly more ambiguous than in the parsimony analyses. Through taxon removal, we explored whether Camptostroma would resolve more precisely than in a stemward polytomy. Only the removal of all three of the other taxa in this polytomy resulted in Camptostroma resolving within the clade containing crinoids, but with weak support (posterior probability of 50.4%). Nevertheless, other alternatives had even weaker support, with Camptostroma as sister to blastoids and all others at 21.3%, or as sister to both blastozoans and crinoids at 28.2%. No tree was found in which Ceratocystis was not the sister group to blastozoans.
In early crinoid phylogeny, an important aspect of crinoid evolution has become clearer by virtue of the increased number of Ordovician crinoids in this analysis. These taxa bring into focus a previously unrecognized early crinoid history characterized by a remarkably diverse assemblage (Figure 11). Many of the earliest taxa possess character states that do not fit with our current understanding of early crinoid architectures without employing ad hoc reasoning. Far more diverse assemblages occur in the early Late Ordovician (Sandbian), with these faunas dominated by familiar camerate, cladid, and disparid clades or subclades (Kolata, Reference Kolata1975; Sprinkle, Reference Sprinkle1982a; Guensburg, Reference Guensburg1984) (Figure 11). Earlier works that attempted to include Early Ordovician taxa in crinoid phylogenies almost never took this divergence into account, in large measure because of fewer available taxa (Guensburg and Sprinkle, Reference Guensburg and Sprinkle2003; Guensburg, Reference Guensburg2010; Ausich et al., Reference Ausich, Rozhnov and Kammer2015b). A more accurate picture of Early Ordovician crinoid history is emerging in which early diversification was rampant, followed by general pruning and later predominance of the more familiar architectures beginning in the late Floian.
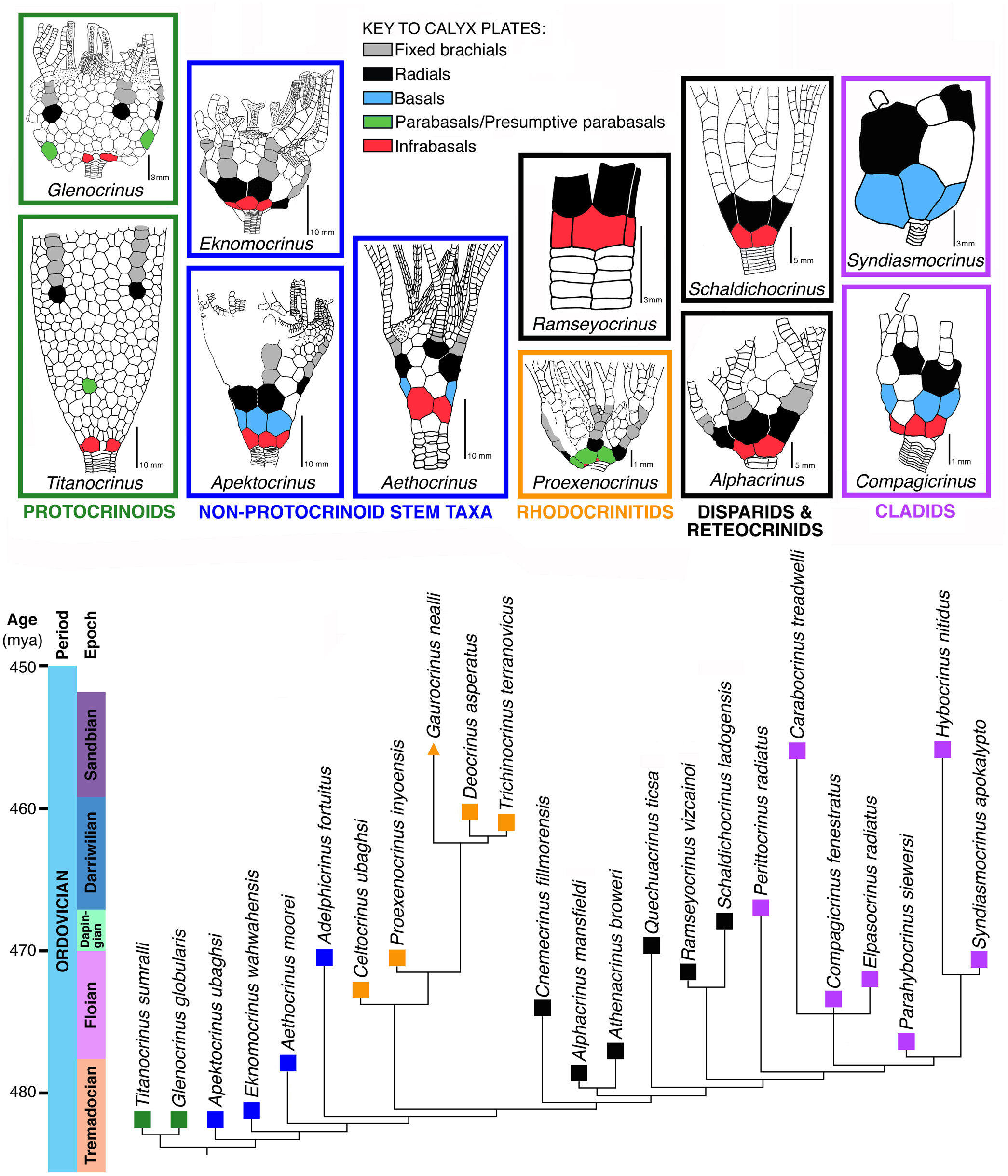
Figure 11. Evolutionary tree for crinoids (based on 50% majority rule consensus, Fig. 9.2). Origination times for taxa are calibrated using the GSA Geologic Time Scale (Walker and Geissman, Reference Walker and Geissman2022) at left; colored squares for taxa correspond to thumbnail figures in upper half of figure. The younger, off scale, Gaurocrinus nealli occurrence is indicated by an arrow. These thumbnails show posterior views of calyx for selected taxa in phylogenetic tree, illustrating key evolutionary changes in configuration of plate circlets among clades.
However, certain apomorphies have been recognized as particularly useful in recognition of subclass-level clades early in crinoid history (Guensburg and Sprinkle, Reference Guensburg and Sprinkle2009; Guensburg, Reference Guensburg2010). Submerged ambulacra (Character 8) originated at the protocrinoid level of organization and persisted among camerate crinoids. Origination of sub-ray posterior calyx morphology (with anal X, radianal, and related plating) (Character 29) constitutes a subclass level apomorphy of cladids expressed since the earliest-known taxa among camerates and disparids. On the other hand, a linear posterior series, or anitaxis (Character 30), links early (Floian), but more crownward, camerates and disparids to the exclusion of cladids (Gahn, Reference Gahn, Zamora and Rabano2015).
Early crinoid phylogeny recovered in this analysis is presented in Figure 11. Major clade boundaries are established based on the key apomorphies delineated above. Crownward to crinoid-stem forms taxa group into long-recognized clades, and in this limited sense, our findings largely agree with traditional classifications (Ubaghs, Reference Ubaghs, Moore and Teichert1978), even those with radically different concepts of homologies and outgroup selection (e.g., Ausich et al., Reference Ausich, Kammer, Rhenberg and Wright2015a).
One peculiar result in this analysis finds reteocrinids sharing common ancestry with a subset of disparid taxa. Reteocrinoids have long been viewed as problematic, in part due to peculiarities of the stem (Guensburg, Reference Guensburg1984, Reference Guensburg1992), but traditionally placed within the Camerata. Cnemecrinus and Quechuacrinus demonstrate the independent deep origins of this group from the rhodocrinitid camerates. Reteocrinids also share an anitaxis with disparids and early camerates. We acknowledge the reteocrinid position generated here may be an artifact of insufficient data, but it does raise questions as to the relationships among all these early lineages (see Gahn, Reference Gahn, Zamora and Rabano2015).
Conclusions
Differentiated primary and epispire-bearing secondary plating characterized earliest-known crinoids, the protocrinoids. Primaries appear to be centers around which relatively smaller secondaries were added sequentially at their margins. Previously formed secondaries gradually shifted distally from these centers during development. Homologous expressions extend back to the early Cambrian stem echinoderms such as Camptostroma. Therefore, the discovery of this configuration in protocrinoids indicates that, for Crinoidea, these are plesiomorphic features. A plesiomorphic crinoid calyx agrees with recently published descriptions, indicating that crinoid arms evolved from an early radial echinoderm morphotype by projection of the axial ambulacra, along with adjacent extraxial body wall enclosing extensions of the left and right coeloms (Mooi et al., Reference Mooi, David, Marchand, David, Guille, Féral and Roux1994; David and Mooi, Reference David and Mooi1996; Mooi and David, Reference Mooi, David, Waters and Maples1997; Guensburg et al., Reference Guensburg, Blake, Sprinkle and Mooi2016, Reference Guensburg, Sprinkle, Mooi, Lefebvre, David, Roux and Derstler2020; Derstler et al., Reference Derstler, Guensburg, Blake and Sprinkle2018). This congruent character evolution in both the crinoid arm and calyx further strengthens the case for independent origin of crinoids from stem pentaradial echinoderms, and not from within the blastozoans. There remains the possibility that blastozoans and crinoids are sister taxa. However, the aforementioned congruence of unique features of the crinoid arm and calyx strongly suggests that blastozoans and crinoids evolved only superficially similar systems of feeding appendages raised into the water column, and not through specialization of crinoids from some subset of blastozoans. Closer examination of these superficially similar, but not homologous constructs, even in the stem itself, indicates major anatomical differences in the ways blastozoans and crinoids responded to selection for raised suspension feeding systems.
Positional evidence reveals certain protocrinoid primaries to be nascent infrabasal, basal, and radial plates of crownward crinoids. These were simplified into their fully differentiated patterns by restriction or elimination of secondaries. Elimination of, or in a few early crinoids, modification of epispires occurred during this transition. Early crinoids such as Perittocrinus and Deocrinus, among others, represent more derived, transitional exemplars with vestiges of the primary-secondary plesiomorphy (Fig. 11). Asynchronous origination of a differentiated basal circlet provides evidence of nonhomology in cladids and diplobathrid camerates. Phylogenetic analysis indicates that superficially similar expressions of diplobathrid camerate and cladid mid-calyx circlet patterns evolved independently. This distinction is signified herein by the use of “basals” in cladids and “parabasals” for diplobathrid camerates.
Elongation of the calyx resulting in addition of embedded fixed-ray plating in a linear sequence (radials, brachials) with intervening interbrachials is part of the same embryological processes by which left and right somatocoels accompanied crinoid arm development. All earliest camerate, cladid, and disparid crinoids exhibit this pattern, supporting the conclusion that this linear fixed-ray series is a stem crinoid apomorphy, preceding or accompanying differentiation of the lower calyx into simplified series of differentiated circlets. This elongation was further reduced to even fewer fixed brachials in later Early Ordovician lineages, accompanying the emergence of what are now recognized as subclass rank taxa: three plates below ray branching within the calyx of camerates, one or two unequal plates (radial and first primibrachial) in disparids, and a single plate (a radial) in cladids. These reductions culminated later on with adoral (upper) radial margins forming the calyx/tegmen boundary.
In spite of its strong phylogenetic and ontogenetic support, we recognize a potential objection to our system of calyx plate homologies in that the needed changes require ability of the mid-calyx to rotate to accommodate monocyclic and dicyclic patterns. However, this objection is mitigated by evidence from protocrinoids themselves and later middle Paleozoic lineages that show transition from dicyclic to monocyclic patterns or expansion of the mid-calyx by addition of plates (Fig. 5.3–5.5). Therefore, dicyclicity and monocyclicity early in the crinoid record, prior to later canalization within familiar clades, is of limited phylogenetic significance. This kind of variation in expression is a demonstrable hallmark of the extraxial body wall region of which the calyx is constructed.
Based upon the present record, standardization of camerate, cladid, and disparid calyx configurations seem to have been derived independently from forms that first emerged during the Early Ordovician (Fig. 11). Morphologies characterizing traditional camerate, cladid, and disparid clades had fully emerged by the end of the Early Ordovician, although certain plesiomorphic traits persisted in a few lineages.
We have been able to demonstrate that calyx plate patterns simplify through loss of secondaries, although sometimes incompletely (e.g., Perittocrinus), in taxa above the node at which the protocrinoids branch. The question of how these crownward patterns appear remains somewhat open in the sense that there remain few data to indicate exactly which primaries in the calyx region were eliminated in conjunction with secondary loss during plate suppression. With the recognition that such changes occur, the next step will be to determine if and how this happens convergently among crinoids, and to obtain ontogenetic or phylogenetic data to illustrate how plate patterns are altered in taxa in which the plate losses are strongly suggested to be homologous.
We also have been able to show that in the calyx, notably outside the tegmen, epispires are also lost during crinoid evolution. We know next to nothing about how the expression of epispires and primary and secondary plates is regulated, and it is equally unknown whether these might be regulated as a unit. Mapping their expression patterns onto phylogenies could indicate whether or not loss of these different features occurs as separate evolutionary events. For example, it would seem that forms such as Adelphicrinus, which possesses epispires, seem to have what could be identified as secondary plates in fields filling interbrachial areas only. This implies that expression of primary and secondary plates is independent, and their expression seems similarly decoupled among other crinoids as well. Whether that kind of decoupling is also true for epispires or whether they are ineluctably linked to expression of primaries (at least) is difficult to determine. This is because when epispires are absent, differentiation of primary from secondary plates becomes problematic. In other words, given that secondary plates seem to be the first calycinal system to be lost through reduction of plate addition during crinoid evolution, epispires or their derivatives (e.g., goniospires) remain the only hallmarks of the primary-secondary plate system, even in cases when only the primary plates remain (e.g., in Carabocrinus).
The recovery of strong evidence that epispires accompany the expression of primary-secondary plating in earliest crinoids as well as early pentaradiate forms has major implications for the interpretation of body wall patterning among crinoids in general. Although the question was set aside in argumentation concerning the unique nature of crinoid arms and their distinction from brachioles (Guensburg et al., Reference Guensburg, Blake, Sprinkle and Mooi2016), previous interpretations largely left open the question of whether crinoid calyces were perforate extraxial, imperforate extraxial, or a combination of the two. Mooi and David (Reference Mooi and David2008, fig. 2) hinted at the possibility that the tegmen was a sole remnant of perforate extraxial region, due to its association with the left somatocoel. The right somatocoel, in occupying the bulk of the remainder of the calyx, implied strong expression of imperforate extraxial body wall aborally, and into the stem. However, in earliest crinoids, perforate extraxial (as characterized by the presence of epispires, at least) seems to dominate large areas of the calyx, due to homology with perforate extraxial regions in early pentaradial forms. This, in turn, implies that the brachials of crinoids, which have been suggested to be part of the imperforate extraxial region due to their consistent association with the right somatocoel in the arms, are either connected to the remainder of the imperforate region in the stem through a hitherto unrecognized sequence of plates that was not yet lost from the calyx of earliest crinoids, or they are genuinely disconnected from that region. However, a third possibility exists in which there is actually no imperforate extraxial body wall in either the arms or the calyx, and that it is restricted to the stem and, at most, the aboralmost basal circlet in the form of the infrabasals. These observations are presently under consideration in the context of the extraxial-axial theory in a more general work on crinoid body wall homologies.
Both the phylogenetic analysis (Figs. 9, 10) and the fossil record as it is now understood show that clavate stem-bearing architecture evolved independently in early pentaradial echinoderms by extension from a similar circumferential position near, but below ambulacra. Distantly related forms such as blastozoans, crinoids, and certain pedunculate edrioasteroids (edrioblastoids) are all constructed in this way because of phylogenetic constraints, but each is derived independently from ancestors that lacked stems entirely. Our analyses do not rule out the possibility that blastozoans and crinoids are sister taxa. However, if Camptostroma is on the lineage to the crinoids, as strongly suggested by recent data on its morphology and its position in the parsimony analyses, then blastozoans and crinoids would each have non-pedunculate taxa in their ancestry: imbricates for the blastozoans, and Camptostroma for the crinoids. Crinoids evolved pinnulate arms instead of brachioles, and in a fashion similar to, but not at all homologous with those of blastozoans (see Guensburg et al., Reference Guensburg, Blake, Sprinkle and Mooi2016, Reference Guensburg, Sprinkle, Mooi, Lefebvre, David, Roux and Derstler2020, Reference Guensburg, Sprinkle, Mooi and Lefebvre2021), and added a stem to hold the entire complex feeding apparatus up in the water column. As the present study indicates, specialization of the calyx, and consolidation of mid-cup plate architecture served not only to strengthen that part of the animal devoted to supporting a widely spread system of arms in the water column, but to lighten the entire structure.
The analysis presented here does not attempt to place the other four extant classes, Asteroidea, Ophiuroidea, Echinoidea, and Holothuroidea (the “AOEH” clade). In some analyses, it is tacitly assumed (or recovered) that the AOEH clade comprises a sister to the Crinoidea (e.g., David et al., Reference David, Lefebvre, Mooi and Parsley2000). Others recover the AOEH clade in a topology that harkens back to the concept of the eleutherozoans (e.g., Deline et al., Reference Deline, Thompson, Smith, Zamora, Rahman, Sheffield, Ausich, Kammer and Sumrall2020). This scenario is much as described by Smith and Jell (Reference Smith and Jell1990), in which asterozoans (and presumably the remainder of the AOEH clade) would be derived from a subset of the earliest pentaradial forms. In our assessment, placement of the AOEH clade depends on hypotheses of homology of structures such as arms, and the configuration of body wall regions (Mooi and David, Reference Mooi, David, Waters and Maples1997). If the arms of crinoids and asterozoans are homologous, then there would be support for placement of the AOEH clade as sister to the crinoids in the context of the analysis presented here. However, there is some support for the idea that arms of crinoids and those of asterozoans are not homologous, as implied by the topology in Deline et al. (Reference Deline, Thompson, Smith, Zamora, Rahman, Sheffield, Ausich, Kammer and Sumrall2020). Although tests of these ideas were beyond the scope of the present work, such considerations are important in the context of recent discoveries such as Yorkicystis (Zamora et al., Reference Zamora, Rahman, Sumrall, Gibson and Thompson2022), as well as the debate over asterozoan origins, as discussed in Hunter and Ortega-Hernández (Reference Hunter and Ortega-Hernández2021) and Blake and Hotchkiss (Reference Blake and Hotchkiss2022). We agree with the latter authors that Hunter and Ortega-Hernández (Reference Hunter and Ortega-Hernández2021) have misinterpreted the nature of virgals through a misapplication of the extraxial-axial theory. This debate, which places emphasis on the placement of crinoids as sister to asterozoans, requires a more rigorous exploration of alternative topologies than has been presented to date.
Acknowledgments
Special thanks are due to F. Gahn, Brigham Young University, for initial discussions of crinoid plate homologies and for commenting on an earlier draft of the manuscript. Casts of Camptostroma roddyi were generously provided to the Field Museum, Chicago, courtesy of K. Derstler, University of New Orleans. D. Quednau of the Field Museum, Chicago, provided valued expertise in preparing illustrations. P. Mayer, also of the Field Museum, provided curatorial assistance. J. Thomas, University of Illinois, provided additional help with imagery. We gratefully acknowledge the helpful reviews of C. Paul, who ably presented views different from ours, compelling us to revise and strengthen some of our argumentation, and B. Lefebrve, who pointed out refinements in the text and the matrix. The editors, notably S. Zamora, provided guidance in clarifying parts of the manuscript. Their views are not necessarily those of the authors. NMK was supported by NSF grant DEB 2036186 (awarded to G.W. Rouse). RM was supported by his NSF grant, DEB 2036298.
Declaration of competing interests
The authors declare no competing interests.
Appendix 1
Character analysis
1. Left and right somatocoels.—Left and right somatocoels underlie ambulacra along their entire length, including instances in which such a relationship exists in feeding appendages (0); free ambulacra (1). State 0 includes those feeding appendage-bearing taxa with cavities extending uninterrupted from thecal shoulders and extending into feeding appendages. State (1) includes taxa without accompanying left and right somatocoel-bearing appendages. This latter condition is signified by the lack of cavities for somatocoels extending through thecal shoulders. As regards this study, condition 0 is observed in Cambrian edrioasteroid-like radiate echinoderms, including Camptostroma, with its apparent short feeding appendages (Durham, Reference Durham1966; Derstler et al., Reference Derstler, Guensburg, Blake and Sprinkle2018), and crinoids. Clausen et al. (Reference Clausen, Jell, Legrain and Smith2009) and Sheffield and Sumrall (Reference Sheffield and Sumrall2019) each proposed blastozoan intermediaries linking states 0 and 1 (that is, blastozoans with left and right somatocoel extensions in feeding appendages) but, in each case, evidence has been published contradicting these claims (Guensburg et al., Reference Guensburg, Blake, Sprinkle and Mooi2016, Reference Guensburg, Sprinkle, Mooi, Lefebvre, David, Roux and Derstler2020, Reference Guensburg, Sprinkle, Mooi and Lefebvre2021).
2. Podial pores or basins.—Present (0); absent (1). Determining the existence of podial pores or podial basins is crucial to assessing relationships among early crinoids, as well as with other early echinoderm groups. The fossils can be difficult to interpret when weathering and diagenesis obscure plate boundaries in fossils such as the ones treated here (see section on Taphonomy and preparation). The best supported interpretation, obtained by coated, submersed, and dry images, is that there are podial basins if not actual pores in basins that could extend to water vascular elements such as ampullae that lie internal to the floor plates. Although not documented in later Paleozoic crinoids, these structures can be seen in Aethocrinus, Athenacrinus, Apektocrinus, Titanocrinus, and possibly Glenocrinus (Guensburg et al., Reference Guensburg, Sprinkle, Mooi, Lefebvre, David, Roux and Derstler2020, figs. 4.4, 4,6, 10.3, 10.4). Derstler et al. (Reference Derstler, Guensburg, Blake and Sprinkle2018) uncovered evidence that feeding appendages of Camptostroma consist of axial and epispire-bearing regions (i.e., are true arms) accompanied by podial pores; this interpretation is tentatively accepted pending further confirmation. Therefore, any internal manifestations of the water vascular system, such as ampullae, would be wholly contained within the body cavity, similar, but not necessarily homologous to the condition seen in crownward asteroids.
3. Floor plates on the theca.—Floor plates short, relatively wide (0); long, relatively narrow (1). This trait does not code for appendage morphology.
4. Floor plates in appendages.—Plates thin, slat-like, not providing primary appendage supports (0); thick, blocky, forming primary appendage skeletal supports (1). This character is inapplicable for taxa lacking feeding appendages.
5. Ambulacral cover plates (when hinged).—Arranged in lateral and medial tiers (0); in a single biseries of lateral plates (medial tier not expressed) (1). Medial and lateral tiers were previously referred to as primary and secondary cover plates (Paul and Smith, Reference Paul and Smith1984, utilized ‘primary’ for lateral, and ‘secondary’ for medial). Single cover plate tiers can be arranged in an alternating double or other multiple series, but essentially forming one level. This differs from the two-tiered pattern where cover plates form two distinct levels (Guensburg et al., Reference Guensburg, Sprinkle, Mooi, Lefebvre, David, Roux and Derstler2020). Patterns can be difficult to interpret in plesiomorphic Cambrian forms where plates are more irregular, but an incipient two-tiered pattern can be discerned (Smith and Jell, Reference Smith and Jell1990; Zhao et al., Reference Zhao, Sumrall, Parsley and Peng2010).
6. Medial cover plates.—Graduated overlapping elements diminishing in size as they arch over the perradial suture (0); an alternating double biseries (1). This character requires medial cover plates and is scored as inapplicable for those taxa lacking medial cover plates.
7. Axial orals.—Absent (0); expressed as differentiated interradial elements surrounding the peristome in all interrays and forming junctions of ambulacra, with multiple CD elements (1). Incongruent plate number and hydropore configuration support homoplasy with similarly positioned oral-like plates such as those of modern crinoids or of the Late Ordovician Hybocrinus nitidus and Carabocrinus treadwelli (see Guensburg et al., Reference Guensburg, Blake, Sprinkle and Mooi2016, for supporting argumentation). The plating of the oral region of Stromatocystites pentangularis includes oral-like plating in the AB and EA interrays (Zamora et al., Reference Zamora, Lefebvre, Hosgör, Franzen, Nardin, Fatka and Álvaro2015, fig. 3.3). This latter novel state is interpreted autapomorphic among the taxa studied and is omitted from the analysis.
8. Hinged thecal (non-appendage) cover plates: operative, that is, capable of opening and closing (0); fixed, forming closed ambulacral tunnels over the thecal surface (1).
9. Brachioles.—Absent (0); present (1). Brachioles are entirely axial in construction whether uniserial or biserial, their primary support structures arise from (axial) floor plates, except in a few derived taxa.
10. Fixed portion of coelom-bearing rays.—Contacted entirely by non-standardized secondary (extraxial) plating (0); contacted by standardized circlet(s) in part or entirely (1). Fixed rays are a uniserial series in continuity with the plate series constituting the primary appendage support. This character is inapplicable for those taxa lacking true arms (sensu David and Mooi, Reference David and Mooi1996, and David et al.. Reference David, Lefebvre, Mooi and Parsley2000) and intended to identify taxa lacking an organized calyx of articulating circlets. A broad survey of all stemmed echinoderms (blastozoans, crinoids, certain edrioasteroids) reveals a strong tendency for radial-interradial organization, underscoring potential for homoplasy.
11. Calyx/thecal respiratory structures.—Epispires (0); not visible (1); goniospires or other pleated structures at plate corners (2). State (2) includes taxa with thin, corrugated stereom. This character is scored inapplicable for Eumorphocystis and Hemicosmites, each of which expresses unique respiratory anatomy, diplopores and pore rhombs, respectively; therefore, coding them would be parsimony uninformative.
12. Circlet forming thecal base with trilobate or irregular stem lumen (basals).—Absent (0); present (1).
13. Dorsal cup or calyx.—Conical (0); bowl-shaped (1). The term “dorsal cup” requires left and right somatocoels extending from the thecal shoulders (character 1 above). This character is inapplicable for those taxa lacking true arms according to David and Mooi (Reference David and Mooi1996) and David et al. (Reference David, Lefebvre, Mooi and Parsley2000) (see character 19).
14. CD interradius elevation.—Not expressed except for periproct or anal cone (0); long cylindrical sac (1). Those taxa in which the tegmen is expanded into a domal structure with submerged ambulacra are scored as (0).
15. CD interradius gap plate(s) (or “open” calyx infrabasal circlet).—Present (0); absent (1). This character requires the presence of true arms. State (0) requires extension of the CD interray gap to the stem/stalk (i.e., they interrupt the cup base circlet). Gap plates are relatively small and interrupt the thecal infrabasal circlet (character 4).
16. Mid-calyx circlet, basals or parabasals, contacting radials and, if present, infrabasals.—Absent (0); basals (1); parabasals (2). State (1) requires the presence of true arms and is therefore marked as not applicable in cases when true arms are absent (character 19). In addition, this character is inapplicable for protocrinoids, which lack primary circlets but do express potential basals. State (2) requires submerged ambulacra on tegmen (character 7). States (1) and (2) are supported by the timing of origin of the mid-calyx. Hybocrinids lack infrabasals, but their former presence is indicated by alignment of the basal circlet and stem meres at the calyx base, in violation of the ‘Law of Wachsmuth and Springer.’
17. Secondary median grooves in feeding appendages.—Absent (0); expressed in feeding appendages (1). State (1) refers to a subsidiary channel along the interior aboral surface of the presumed coelomic channels in feeding appendages and extending from the theca. This groove could have housed the brachial nerve.
18. True radials.—First thecal plate bearing coelomic notch (sometimes early in ontogeny): absent (0); present (1). True radials are present in all crinoids, even those lacking free arms (certain hybocrinids, microcrinoids, others). The blastozoan Eumorphocystis expresses extraxial elements superficially similar to radials of the type seen in derived crinoids, in which these elements form the adoral region of the cup. However, unlike crinoids, the posited Eumorphocystis ‘radials’ have no coelomic notches or other evidence of any communication to the thecal interior, including those earlier in ontogeny (see Sheffield and Sumrall, Reference Sheffield and Sumrall2019; Guensburg et al., Reference Guensburg, Sprinkle, Mooi and Lefebvre2021).
19. True arms, feeding appendages expressing left and right somatocoels extended off the theca.—Absent (0); present (1). This character does not necessarily require true radial or brachial plates, but this is the case for nearly all taxa. Derstler et al. (Reference Derstler, Guensburg, Blake and Sprinkle2018) followed Durham (Reference Durham1966) in reporting short arms with apparent aboral extraxial, plating in Camptostroma. This finding is tentatively accepted.
20. True arm branching pattern.—True arms atomous, non-branching (0); isotomously branching (1); endotomously branching (2). This character is scored inapplicable for taxa lacking true arms (character 19). Potential arms in Camptostroma reported by Durham (Reference Durham1966) and Derstler et al. (Reference Derstler, Guensburg, Blake and Sprinkle2018) are atomous and coded as such. However, Paul and Smith (Reference Paul and Smith1984) did not show arms in their reconstruction of Camptostroma, and the issue remains open to further study.
21. Brachials.—Absent (0); present (1). These are primary supporting elements for free rays cradling canals arising from thecal shoulders apart from the peristome. Brachials are extraxial and form primary appendage supports (Mooi and David, Reference Mooi, David, Marchand, David, Guille, Féral and Roux1994). This character requires feeding appendages and is scored inapplicable for taxa lacking feeding appendages. Eumorphocystis is unique among blastozoans in that its feeding appendages also have a uniserial series of aboral backing plates. Backing and brachial plates were proposed to be homologous (see Sheffield and Sumrall, Reference Sheffield and Sumrall2019). However, restudy of additional Eumorphocystis specimens concluded that proposed anatomical similarities and inferred homologies could not be corroborated (Guensburg et al., Reference Guensburg, Sprinkle, Mooi and Lefebvre2021). Both types of plates cannot be anything other than extraxial, which does not in itself support an a priori inference of their homology. Eumorphocystis backing plates constitute an apomorphy unique to that taxon and are scored inapplicable in this analysis.
22. Extraxial laterals.—Present, accompanying extended thecal wall out along arms; present (0); absent (1). This state requires true arms and is scored inapplicable for taxa lacking true arms. Extraxial laterals, when present, occupy aboral arm surfaces aside from brachials. Here, (0) requires continuity of these plates out along the arms and therefore Carabocrinus treadwelli, which expresses small plates disposed in alcoves running up the proximal arms, is scored (1).
23. Platelet webs at branchings.—Present (0); absent (1). These plate fields are most parsimoniously regarded as extensions of extraxial lateral plating (see character 22). This character requires true arms and is scored inapplicable for taxa lacking true arms.
24. Fixed brachials.—Present (0); absent (1). Fixed brachials are ray plates that extend aborally from true radials and are embedded in the cup; they articulate laterally with interradial plates. This character requires true arms and is scored inapplicable for taxa lacking true arms.
25. Calyx-like fixed brachials.—Three or more in all rays (0); none to two in all rays (1). “Calyx-like” indicates plates embedded in the cup with margins flush with adjacent cup plates, much like radials. This character requires true arms and is therefore scored inapplicable for taxa lacking true arms. Disparids have fixed brachials (biradials) in varying numbers of rays. None of these patterns occurs in more than a single coded taxon and are therefore uninformative in this analysis.
26. One or more brachial pairs in lateral union above branchings.—Present (0); absent, not paired above branchings (1). This character requires true arms and branchings and is therefore scored inapplicable for taxa lacking true arms or taxa without branches.
27. Interradial plate fields separating multiple fixed primibrachials in adjacent rays.—Much wider than fixed rays (0); interradial fields not as wide as fixed rays (1); interradial plate fields absent (2). Width is assessed across the widest portion of the field and compared with the widest fixed brachial. This character requires true arms and is therefore scored inapplicable for taxa lacking true arms.
28. CD interradius.—CD interradius extending downward to the base of the thecal cavity (0); contacting mid-calyx circlet (1); ending at true radials (2). State (0) indicates the calyx primordial circlet is interrupted across the CD interradius, state (1) requires laterally contiguous mid-calyx circlet (or dicyclic calyx) and state (2) indicates radials are laterally contiguous below the CD interradius. This character requires the presence of true arms and is therefore scored as not applicable for those forms lacking them.
29. Radianal(s) and anal X plates.—Absent (0); present (1). State (1) consists of differentiated plates occupying the space below and to the left of a “raised” C radial. The radianal can be absent in later, more derived taxa, but not those treated here. States (0) and (1) require presence of true arms and are scored as not applicable for those forms lacking them.
30. Anibrachial plate.—Absent (0); or present (1). This character also implies the presence of an anitaxis, a plate column branching from the C ray, and requires true arms, so is scored inapplicable for those taxa lacking true arms.
31. Peduncle, stem, or stalk.—Absent or only slightly developed as attachment structure (0); imbricate plated peduncle (1); irregularly tessellated peduncle with pinched demarcation at base of theca (2); monomeric (holomeric) stem (3); pentameric or tetrameric stalk or stem (4). Carabocrinus treadwelli and Hybocrinus nitidus pentameres are inconspicuous (see Sprinkle, Reference Sprinkle and Sprinkle1982b, fig. 40G). Note: the presence of a stem traditionally has been used as a key feature linking blastozoans and crinoids, to make up the “pelmatozoans.” Stems are now known among edrioasteroids as well as blastozoans and crinoids (Guensburg and Sprinkle, Reference Guensburg and Sprinkle2007; Guensburg et al., Reference Guensburg, Blake, Sprinkle and Mooi2016). That stems/stalks evolved more than once is evident (Sprinkle, Reference Sprinkle1973). Here we identify types of stems in which, at least among treated taxa, a pattern emerges whereby blastozoan and earliest crinoid stems are distinguishable. This approach does not apply to later, more crownward taxa in which homoplasy leads to similar constructs that are superficially similar. That the earliest forms of both blastozoans and crinoids did not have peduncles like those in more crownward forms further underscores the fact that these structures are not homologous in the two clades.
32. Stalk/stem lumen.—Lacking (0); subround or irregular trilobate in cross section (1); pentagonal to pentalobate or tetralobate in cross section (2). States (1) and (2) require a stem and (0) indicates complete absence of a meric stem.
33. Ray length on theca.—Long, approaching the stem in extraxial body wall (0); short, restricted to the region around peristome and not approaching the stem (1).
34. Extraxial “orals”.—Absent (0); present (1). The interradial circlet bordering the peristome of Hybocrinus nitidus and Carabocrinus treadwelli is considered extraxial and homologous among these and certain other “cyathocrinine” crinoids (e.g., Porocrinus, Palaeocrinus); these are all characterized by a nearly flat tegmen of few plates and with a hydropore within a single posterior “oral.”
35. Gonopore.—Differentiated from hydropore (0); undifferentiated from hydropore (1). State (0) requires an opening in the CD interray separate from the hydropore. Although unobserved in Pseudriophus guensburgi, this taxon is very similar to other edrioasterid edrioasteroids and therefore also scored as state (1).
36. Hydropore or combined hydropore-gonopore.—An interplate pore bordered by small platelets (0); a slit shared across two plates separate from hydropore (1); an intraplate pore (2); subcircular pore shared across two plates (3).
37. Pinnules.—Absent (0); present (1). This character requires true arms. Pinnules are supported by extraxial elements, and are constructed nearly identically to true arms, including containment of coeloms characteristic of arms. Pinnules are not homologous with brachioles, which can nonetheless superficially resemble pinnules, as in Eumorphocystis.
38. Ray branching in calyx/theca.—None (0); branching from fixed brachial on the theca (1).
39. Uniserial posterior plate column or anitaxis.—Absent (0); present (1). This character requires true arms and therefore is inapplicable for taxa lacking them.
40. Secondary thecal plates arrayed around primaries.—Absent (0); present (1). This character implies multiple calcification centers around primary margins.
41. Secondaries arrayed in complete or hemi-rosettes.—Absent (0); present (1). Secondaries are in continuous lateral contact.
42. Thecal basal concavity.—Absent (0); present (1). This character requires true arms and is therefore inapplicable for taxa lacking them.
43. Calyx base circlet.—Infrabasals alternating with stem meres (0); basals aligned with meres (1). This character is inapplicable for taxa lacking true arms.
44. Stem/theca juncture.—Interlocking or undulating (0); subplanar to planar (1). This character requires true arms and is scored inapplicable for those lacking them.
Appendix 2
Matrix used in phylogenetic analysis
