Introduction
The macula is an oval-shaped area at the centre of the retina which consists of a dense collection of light-sensitive cone cells responsible for central, high-resolution vision and colour perception(Reference Hirsch and Curcio1). At the centre of the macula lies the fovea, where macular pigment (MP) is located. MP is comprised of three carotenoids: lutein, zeaxanthin and the retinal metabolite of lutein, meso-zeaxanthin(Reference Bone, Landrum and Hime2, Reference Bernstein, Li and Vachali3). Lutein and zeaxanthin are not synthesised de novo in humans but are exclusively of dietary origin, typically derived from a diet rich in coloured fruit and vegetables(Reference Sommerburg, Keunen and Bird4), while meso-zeaxanthin can be generated by conversion from retinal lutein(Reference Johnson, Neuringer and Russell5) or may be obtained from dietary sources such as trout and salmon(Reference Nolan, Meagher and Kashani6). The highest concentration of MP is found in the receptor axon layer of the foveola, while in the parafovea, MP is located in the inner plexiform layers(Reference Snodderly, Auran and Delori7, Reference Trieschmann, Van Kuijk and Alexander8), an area of the retina which is primed for the generation of reactive oxygen species (ROS) and consequently, oxidative damage(Reference Beatty, Koh and Phil9). These hydroxyl-carotenoids are selectively located in the macula to the exclusion of all other dietary carotenoids. Fig. 1 highlights the location of MP and the macula within the eye.

Fig. 1. Diagram highlighting the location of macular pigment and the macula within the eye. For a colour figure, see the online version of the paper.
MP has a number of important functions in the eye. Evidence suggests that MP contributes to visual performance and/or experience, as it acts as an optical filter for blue light (peak absorption approximately 460 nm)(Reference Bernstein, Li and Vachali3, Reference Loughman, Davison and Nolan10). The importance of MP’s antioxidant and anti-inflammatory properties is supported by its capacity to protect against the cumulative damaging effects of oxidative stress(Reference Beatty, Koh and Phil9), and inflammation(Reference Li, Fung and Fu11), which affect the macula in conditions such as age-related macular degeneration (AMD)(Reference Seddon, Ajani and Sperduto12–Reference Chew, Clemons and SanGiovanni15). In light of this evidence, the relatively recent emphasis on MP as a possible ocular-protectant in diabetes(Reference Lima, Rosen and Maia16), a pathological condition similarly associated with inflammation, oxidative stress and progressive retinal damage, is logical and represents a natural extension of previous work in AMD(Reference Seddon, Ajani and Sperduto12–Reference Chew, Clemons and SanGiovanni15).
The underlying molecular mechanisms associated with the onset of diabetes and the potential protective effects of lutein and/or zeaxanthin against retinal oxidative damage, inflammation and visual function have been explored in diabetic murine models(Reference Sasaki, Ozawa and Kurihara17–Reference Arnal, Miranda and Johnsen-Soriano23). The onset of diabetes in alloxan and streptozotocin diabetic mice and rats was accompanied by an increase in markers of oxidative stress and inflammation including: malondialdehyde, 8-hydroxy-2’deoxyguanosine, NF-B and vascular endothelial growth factor (VEGF), with a concomitant decrease in local antioxidants including glutathione (GSH) and glutathione peroxidase (GPx)(Reference Sasaki, Ozawa and Kurihara17–Reference Kowluru, Menon and Gierhart19). Importantly, treatment with antioxidants (lutein and/or zeaxanthin) has been shown to lower oxidative stress and inflammation and increase endogenous antioxidants; findings which in some(Reference Sasaki, Ozawa and Kurihara17, Reference Muriach, Bosch-Morell and Alexander18) but not all(Reference Kowluru, Zhong and Santos20) studies occurred independently of any effects on hyperglycaemia. The beneficial effects of these carotenoids on retinal function have also been observed in diabetic murine models, and these include the preservation of electro-retinogram b-wave amplitude and prevention of neurodegenerative effects on the inner retinal layers(Reference Sasaki, Ozawa and Kurihara17–Reference Kowluru, Menon and Gierhart19). Findings from these studies provide important insights into the potential role that MP may have in protecting against diabetic retinal disease; however, the observed findings need to be interpreted with caution, as higher MP dosages have been used in animal studies compared with those used clinically in humans, and many of these studies have never conclusively demonstrated retinal uptake of the administered carotenoids beyond the retinal pigment epithelium (RPE) and choroid(Reference Gorusupudi and Vallikannan24, Reference Mamatha and Baskaran25). A summary of the experimental animal studies examining MP optical density (MPOD) in diabetes is outlined in Table 1.
Table 1. Summary of experimental animal studies examining the relationship between lutein and/or zeaxanthin and diabetes mellitus (DM)

MDA, malondialdehyde; GSH, glutathione; GPx, glutathione peroxidase; ERG, electroretinogram; STZ- streptozotocin; 8-OHdG, 8-hydroxy-2’deoxyguanosin; iNOS, inducible NO synthase; VEGF, vascular endothelial growth factor; ICAM-1, intercellular adhesion molecule; ROS, reactive oxygen species; ERK, extra-cellular receptor kinase; BiP, binding Ig protein; PERK, protein kinase RNA-like ER kinase; ATF6, activating transcription factor 6; AMPK, AMP-activated protein kinase; Mn SOD, Mn superoxide dismutase; FOXO3α forkhead O transcription factor 3 α; p-AKT; phosphorylated serine/threonine kinase.
A number of cross-sectional studies(Reference Lima, Rosen and Maia16, Reference Davies and Morland26–Reference Mares, LaRowe and Snodderly29), including findings from our own study group(Reference Scanlon, Connell and Ratzlaff28), and randomised controlled trials (RCT)(Reference Hu, Hu and Lin30, Reference Chous, Richer and Gerson31), have explored the relationship between type 1 and type 2 diabetes and MPOD in humans. MPOD is generally found to be lower in diabetes(Reference Lima, Rosen and Maia16, Reference Davies and Morland26, Reference Scanlon, Connell and Ratzlaff28), with some studies suggesting that oxidative stress is implicated in its depletion(Reference Lima, Rosen and Maia16, Reference Davies and Morland26). Lower levels of MPOD have also been associated with raised glycated Hb (HbA1c) levels and the presence of retinopathy in patients with type 2 diabetes(Reference Lima, Rosen and Maia16). In another study, patients with diabetes (type 1 and 2) with grade 2 diabetic maculopathy had significantly lower MPOD compared with those with no maculopathy (P = 0·016)(Reference Davies and Morland26).
Although oxidative stress is implicated as a causative factor, the exact reasons why MP is lower in diabetes, and type 2 diabetes in particular(Reference Lima, Rosen and Maia16, Reference Scanlon, Connell and Ratzlaff28), are not fully understood. One study found lower levels of MP in type 2 v. type 1 diabetes(Reference Scanlon, Connell and Ratzlaff28), suggesting that other coincident metabolic and pathological abnormalities which are characteristic of type 2 diabetes (for example, overweight/obesity, dyslipidaemia) may explain the lower MPOD levels observed. Furthermore, lutein and zeaxanthin supplementation has been shown to exert positive and beneficial ocular effects in those with diabetic eye disease, including structural improvements in measures of macular oedema and functional improvements in visual acuity and other visual function measures(Reference Hu, Hu and Lin30, Reference Chous, Richer and Gerson31). Table 2 outlines a summary of the cross-sectional studies and RCT examining the relationship between MPOD and diabetes (type 1 and 2).
Table 2. Summary of cross-sectional studies and randomised controlled trials (RCT) examining the relationship between macular pigment optical density (MPOD) and diabetes mellitus

MP, macular pigment; CAREDS, Carotenoids in Age-Related Eye Disease Study; HFP heterochromatic flicker photometry; HbA1c, glycated Hb; DR, diabetic retinopathy; OCT, optical coherence tomography; mfERG; multifocal electroretinography; OCTA, optical coherence tomography angiography; VA, visual acuity; CS, contrast sensitivity; DiVFuSS, Diabetes Visual Function Supplement Study; NPDR, non-proliferative diabetic retinopathy; hs-CRP, high-sensitivity C-reactive protein; DPN, diabetic peripheral neuropathy.
Although the evidence exploring MP and diabetes is relatively sparse, the findings that have been reported are sufficient to suggest that MPOD may be adversely affected by the condition, and that there is a plausible rationale to explore the possible benefits of lutein and/or zeaxanthin supplementation for ocular health and visual function in diabetes, particularly type 2. The recent generation of such evidence has prompted this literature review, which is designed to explore and elucidate the metabolic perturbations and candidate causal mechanisms which may underpin the likely complex interdependency of MP and diabetic eye disease (references used in Tables 1 and 2(Reference Lima, Rosen and Maia16–Reference Arnal, Miranda and Johnsen-Soriano23, Reference Davies and Morland26–Reference Cennamo, Lanni and Abbate36)).
Literature search methods
For the purpose of the present review, partly because of the relative dearth of higher-level RCT evidence, we have appraised all forms of published research, even lower-level evidence sources, to ensure that the scientific and clinical implications of the available evidence might be synthesised into useful treatise, which accurately reflects what is known about this area, and what remains to be elucidated. The review was carried out in two stages. First, we identified all relevant published articles from human and animal studies which reported on the relationship between MP (lutein and/or zeaxanthin and/or meso-zeaxanthin) and diabetes (type 1 and type 2), up until the year 2019. The second part of the search involved identifying publications which investigated the relationship between the metabolic perturbations typically associated with diabetes, and type 2 diabetes in particular (for example, adiposity/dyslipidaemia) and MP. Pre-selected keywords including ‘lutein’, ‘zeaxanthin’, ‘macular pigment’, ‘diabetes’, ‘diabetes AND MP’, ‘diabetes AND lutein/zeaxanthin’, ‘BMI’, ‘body fat AND diabetes’, ‘adipose’, ‘high density lipoprotein AND diabetes’, ‘triglycerides AND diabetes’, ‘oxidative stress’, ‘inflammation’, ‘hypertension AND MP’, ‘insulin resistance’ and ‘hyperinsulinemia’ were entered into academic databases and search engines including PubMed, Google Scholar, Mendeley, Scopus, Cochrane Library and the ISRCTN registry to define our search of the literature relating to MP and diabetes up until 2019. Although many supporting publications were retrieved, in total, only eight animal studies (Table 1) and eleven human studies (Table 2) were included to help clarify our current understanding of the links between diabetes and MP. A total of thirteen papers on adiposity and MP (Table 3) and seven papers on MP and dyslipidaemia (Table 4) were included to help analyse the relationship between MPOD and the metabolic correlates of type 2 diabetes (adiposity and dyslipidaemia). The overall findings suggest that MP is lower in diabetes, type 2 diabetes in particular, and that supplementation with macular carotenoids and/or other antioxidants may confer protection against diabetic eye disease. To elucidate the causal mechanisms and metabolic perturbations which might possibly explain the lower MP levels observed in diabetes, we will first explore the condition diabetes mellitus itself, including its association with oxidative stress and inflammation; and subsequently present the evidence linking adiposity and dyslipidaemia with type 2 (or poorly controlled type 1) diabetes and MPOD.
Table 3. Summary of studies linking macular pigment optical density (MPOD) and body fat

MP, macular pigment; CAREDS, Carotenoids in Age-Related Eye Disease Study; TILDA, The Irish Longitudinal Study of Ageing; IQ, intelligence quotient; DXA, dual-energy X-ray absorptiometry.
Table 4. Summary of studies linking macular pigment optical density (MPOD) with serum lipids
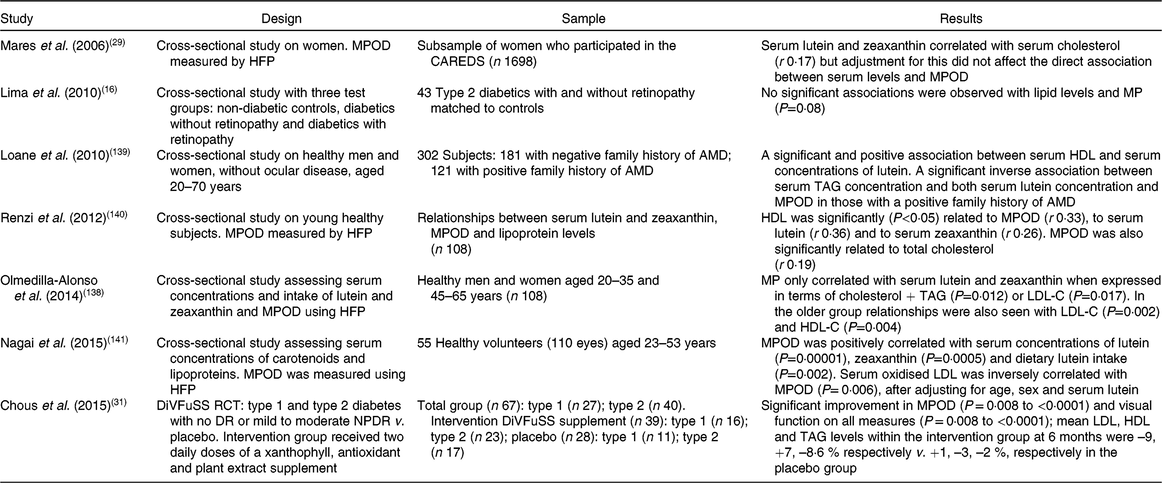
HFP, heterochromatic flicker photometry; CAREDS, Carotenoids in Age-Related Eye Disease Study; MP, macular pigment; AMD, age-related macular degeneration; LDL-C, LDL-cholesterol; HDL-C, HDL-cholesterol; DiVFuSS, Diabetes Visual Function Supplement Study; RCT, randomised controlled trial; DR, diabetic retinopathy; NPDR, non-proliferative diabetic retinopathy.
Diabetes mellitus
Diabetes mellitus is a group of metabolic disorders caused by the complex interaction of genetics, environmental factors and lifestyle choices(Reference Polonsky37). Diabetes is characterised by a deficiency of insulin and/or systemic insulin resistance. Over the past number of decades, the number of individuals with diabetes, particularly type 2 diabetes, has increased dramatically, making it a critical and universal public health challenge(Reference Chen, Magliano and Zimmet38). Type 1 diabetes usually develops in normal-weight children, teenagers and younger adults, and is an autoimmune condition involving the selective destruction of pancreatic β-cells, ultimately resulting in complete deficiency of insulin(Reference Wilkin39). Conversely, type 2 diabetes is linked to a sedentary lifestyle and being overweight, and is characterised by systemic insulin resistance and subsequent pancreatic endocrine dysfunction, which results in attenuated insulin synthesis as well as inhibition of its cellular effects(Reference Polonsky37). Whilst diabetes is characterised by having higher than normal blood glucose levels, the pathogenesis and development of type 1 and type 2 diabetes differ; therefore, the relationship between MPOD and the different forms of diabetes should not be generalised. At presentation, type 2 diabetes is most often accompanied by other co-morbidities including overweight/obesity, insulin resistance, hypertension and dyslipidaemia, features which are less common in type 1 diabetes at diagnosis, but which may occur as complications of type 1 diabetes later in the course of the disease. Oxidative stress and inflammation are implicated in both type 1 and type 2 diabetes; however, research has shown that these metabolic disturbances are very pronounced in type 2 diabetes(Reference Kilhovd, Giardino and Torjesen40–Reference Butkowski and Jelinek44). The oxidative stress, inflammation, adiposity and dyslipidaemia which characterise diabetes may have independent relationships with MPOD, and therefore constitute plausible causal mechanisms in diabetic eye disease. These pathological mechanisms merit further exploration and will be discussed in more detail herein.
Oxidative stress and diabetes
Chronic hyperglycaemia induces oxidative stress in patients with diabetes(Reference Robertson, Harmon and Tran45). Oxidative stress, defined as the excessive production of ROS, results in oxidative injury when the redox balance is upset; i.e. where the level of oxidative species exceeds the capacity of the antioxidant defence system to neutralise them(Reference McCord46, Reference Kowluru and Chan47). ROS can include free radicals, which are partially reduced oxygen species containing one or more unpaired electrons (for example, superoxide anion or hydroxyl radical), and species with their full complement of electrons in an unstable or reactive state (for example, singlet oxygen or H2O2)(Reference Rösen, Nawroth and King48). The body’s natural defence against oxidative damage is neutralisation by endogenous antioxidants(Reference Sies49), which include enzymic antioxidants such as superoxide oxidase (SOD), catalase (CAT) and GPx, and non-enzymic antioxidants such as GSH. Our endogenous antioxidant defence system, however, is incomplete without exogenous antioxidant nutrients including vitamin C, vitamin E, carotenoids (β-carotene, lutein, zeaxanthin and meso-zeaxanthin) and polyphenols(Reference Bouayed and Bohn50). Exogenous antioxidants, such as those available in dietary fruit and vegetables, are therefore necessary to balance redox status. Through normal physiological processes, antioxidants inhibit or quench free radical reactions and can delay or inhibit cellular damage(Reference McCord46). When these processes are overwhelmed, however, as in type 2 diabetes, ROS will readily react with lipids, proteins and nucleic acids, resulting in impaired cell function or cell death(Reference Young and Woodside51, Reference Ceriello52).
The retina is already at an increased risk of oxidative stress and damage, given its high oxygen demand and consumption, its exposure to visible light irradiation and its high concentration of PUFA in the photoreceptor outer segments(Reference Schalch53). These risks increase when the generation of free radicals and ROS is stimulated by environmental factors(Reference Machlin and Bendich54, Reference Borish, Pryor and Venugopal55), short wavelength light exposure(Reference Algvere, Marshall and Seregard56), and other internal stresses including inflammation(Reference Zhang, Liu and Rojas57) and hyperglycaemia(Reference Ceriello52, Reference Cohen, Riahi and Alpert58, Reference Esposito, Nappo and Marfella59). While PUFA in the photoreceptor outer segment are thought to protect against oxidative and inflammatory damage, they can potentially act as a substrate for the propagation of free radicals as they provide a readily available source of electrons(Reference Beatty, Koh and Phil9, Reference Machlin and Bendich54). With increasing age, systemic antioxidant levels decline and ROS levels increase in most tissues, and this is associated with a number of neurodegenerative diseases, including AMD and diabetes(Reference Beatty, Koh and Phil9, Reference Davies and Morland26, Reference Halliwell60).
Hyperglycaemia, oxidative stress and changes in redox homeostasis are fundamental events in the pathogenesis of diabetic retinopathy. Various mechanisms have been suggested to contribute to the formation of reactive oxygen-free radicals. Hyperglycaemia causes tissue damage through five major pathways, namely: increased flux of glucose and other sugars through the polyol pathway; increased intracellular formation of advanced glycation endproducts (AGE); increased expression of the receptor for AGE (RAGE) and its activating ligands; activation of protein kinase-C isoforms; and activity of the hexosamine pathway(Reference Brownlee61). Over-activation of these pathways can lead to damage of cellular organelles and enzymes, increased lipid peroxidation and the development of insulin resistance(Reference Kahn62, Reference Ceriello and Motz63). Several lines of evidence indicate that all five mechanisms are activated by a single upstream event: mitochondrial overproduction of ROS(Reference Brownlee64). Locally, the occurrence of these metabolic changes results in defective angiogenesis and the activation of a number of pro-inflammatory pathways which stimulate an influx of leucocytes which can alter vascular permeability in the retina(Reference Tang, Zhang and Jiang21). The role of hyperglycaemia in diabetic microvascular complications in the eye has been established by large-scale prospective studies in type 2 diabetes (United Kingdom Prospective Diabetes Study)(65). Beyond elevations in blood glucose, however, ROS formation is also increased by elevations in NEFA (a common feature of type 2 diabetes), through their direct effect on mitochondria(Reference Nishikawa, Edelstein and Du66).
While oxidative stress is increased in diabetes, the activities of antioxidant defence enzymes responsible for scavenging free radicals and maintaining redox homeostasis (SOD, CAT, GPx) are concomitantly diminished in the retina of both type 1 and type 2 diabetes(Reference Kowluru and Chan47, Reference Sies49, Reference Matough, Budin and Hamid67). The activity of non-enzymic antioxidants is also depressed during hyperglycaemia-induced oxidative stress(Reference Matough, Budin and Hamid67). Redox balance is important, as its disruption can cause antioxidants to become pro-oxidative, potentially precipitating damage to the retina. It cannot be assumed, however, that a single reductive or antioxidant treatment would be sufficient to reverse the underlying pathophysiological mechanisms of diabetic retinopathy. Antioxidant protection in the retina is provided mainly from vitamins C and E, carotenoids, GPx, SOD and CAT enzymes, and GSH compound(Reference Cabrera and Chihuailaf68), and many of these antioxidants are aided by micronutrients (for example, Cu, Zn, Se) which act as cofactors(Reference Evans and Halliwell69). The carotenoids lutein and zeaxanthin are very effective quenchers of singlet molecular oxygen and lipid peroxy radicals; however, in the process, these carotenoids are themselves oxidised to their corresponding radical cations(Reference Roberts and Dennison70). These cations must be reduced to regenerate the original carotenoid, thereby allowing their reuse as an antioxidant. Vitamin E (α-tocopherol) reduces oxidised carotenoids, which leaves tocopherol oxidised(Reference Evans and Halliwell69). However, oxidised tocopherol can be reduced and regenerated by vitamin C (ascorbic acid) and GSH, which are themselves further reduced by Cu and Zn(Reference Chew, Clemons and SanGiovanni15, Reference Sies, Stahl and Sundquist71). Dietary lutein, zeaxanthin and vitamins E and C, as well as endogenous antioxidant enzymes, therefore act in concert with one another to lower the levels of ROS (via ROS detoxification) and to recycle oxidised lipid antioxidants (via re-reduction).
The presence of oxidation products of the macular carotenoids in human and primate retinal tissue indicates that lutein, zeaxanthin and meso-zeaxanthin play an important antioxidant role there(Reference Khachik, Bernstein and Garland72). Zeaxanthin appears to be a more potent antioxidant than lutein(Reference Cantrell, McGarvey and Truscott73), with meso-zeaxanthin even more efficacious, but only in conjunction with its binding protein(Reference Bhosale and Bernstein74). Li et al.(Reference Li, Ahmed and Bernstein75) demonstrated that a 1:1:1 ratio mixture of lutein, zeaxanthin and meso-zeaxanthin quenches more singlet oxygen than any of these carotenoids individually at the same total concentration(Reference Li, Ahmed and Bernstein75, Reference Akuffo, Nolan and Howard76), suggesting that they are most effective when acting synergistically with one another. Diet is a major lifestyle factor which can greatly influence the incidence and progression of type 2 diabetes. Some dietary and lifestyle modifications associated with enhanced antioxidant supply could therefore be an effective prophylactic means to prevent or limit oxidative stress in diabetic eye disease. Type 2 diabetes is associated with a sedentary lifestyle and being overweight; therefore, patients with type 2 diabetes may not only be exposed to diets which are high in fat and low in antioxidants(Reference Hendrychova, Vytrisalova and Alwarafi77), and to the increased oxidative stress imposed by their diabetes(Reference Lima, Rosen and Maia16, Reference Davies and Morland26, Reference Scanlon, Connell and Ratzlaff28), but may also be subject to greater chronic oxidative stress as a result of their obesity itself(Reference Manna and Jain78). The co-occurrence of these phenomena consequently demands an even greater supply of exogenous antioxidants (vitamins C and E, carotenoids lutein, zeaxanthin and meso-zeaxanthin) to balance redox status and to combat this state of chronic oxidative stress to which the diabetic retina is exposed. While the control of blood glucose is critical in patients with diabetes, neuroprotective strategies and novel antioxidant treatments should also be considered to target the specific aetiologies and underlying pathophysiological mechanisms of the condition, and to ultimately protect against the vascular pathologies of diabetes. It is logical that natural antioxidants (lutein, zeaxanthin and meso-zeaxanthin) may have beneficial implications for diabetic retinopathy in this context. However, larger trials involving intensive assessment of oxidative stress, antioxidant defence activities and their effects on one another in the diabetic state are necessary before empirical supplementation of these carotenoids can be recommended clinically.
Inflammation and diabetes
Diabetic retinopathy has traditionally been considered a disease of the retinal microvasculature, associated with oxidative stress induced by hyperglycaemia. More recently, however, inflammation has been cited as a deleterious factor in the development of diabetic complications(Reference Wellen and Hotamisligil79), and is now also implicated in the pathogenesis of many ocular diseases including AMD(Reference Loane, Kelliher and Beatty80) and diabetic retinopathy(Reference Kowluru81). Numerous interplays exist between inflammation and oxidative stress and vice versa(Reference Ishibashi, Matsui and Maeda82). Hyperglycaemia increases the levels of pro-inflammatory proteins(Reference Wellen and Hotamisligil79), and it is now believed that inflammatory processes underlie many of the functional changes in retinal vasculature observed histologically in early diabetic retinopathy, such as pericyte loss, microaneurysms, and occluded and degenerated capillaries(Reference Semeraro, Cancarini and Rezzola83).
While inflammation is normally a protective response to tissue stress, hyperglycaemia leads to a dysregulation of inflammation, which ultimately becomes chronic. Oxidative stress triggers inflammatory responses, and inflammation also enhances the production of ROS, which creates a self-perpetuating cascade towards oxidative tissue damage(Reference Hollyfield, Bonilha and Rayborn84). The increased expression of many inflammatory proteins in diabetic retinopathy is regulated through the activation of pro-inflammatory transcription factors including NF-κB(Reference Chen, Chen and Wang85). Activation of NF-κB leads to the synthesis of pro-inflammatory molecules such as IL-1, IL-6, TNF-α, c-Jun N-terminal kinase-1 (JNK-1), Iκ-B kinase-β (IKK-β) and growth factors such as VEGF(Reference Tang, Zhang and Jiang21, Reference Chen, Chen and Wang85).
In the retina, the microvascular endothelium forms an effective barrier to control the movement of blood, fluid and proteins across the vessel wall. This barrier is formed by tight junctions, which consist of over forty different proteins and various inflammatory mediators including VEGF, TNF-α, protein kinase-C, IL-1β and IL-6(Reference Semeraro, Cancarini and Rezzola83, Reference Bhagat, Grigorian and Tutela86). Dysfunction of these proteins is highly correlated with the pathogenesis of blood retinal barrier breakdown and increased vascular permeability, which leads to diabetic macular oedema, a major cause of vision loss in diabetic retinopathy(Reference Zhang, Zeng and Bao87). Inflammatory processes can induce oxidative stress, and similarly, oxidative stress can induce inflammation through the activation of multiple pathways. The coincident over-activation of inflammation and oxidative stress can therefore deplete cellular antioxidant capacity and reduce MP levels in the eye(Reference Beatty, Koh and Phil9, Reference Lima, Rosen and Farah88).
The expression of various pro-inflammatory cytokines, such as TNF-α, IL-1 and IL-6, is also increased from visceral adipose tissue (more common in type 2 diabetes), and this has been linked to systemic inflammation and accompanying insulin resistance(Reference McArdle, Finucane and Connaughton89). The inhibition of signalling downstream of the insulin receptor is a primary mechanism through which inflammatory signalling leads to insulin resistance(Reference Wellen and Hotamisligil79). Several cross-sectional studies have shown that insulin resistance and type 2 diabetes are associated with higher levels of C-reactive protein (CRP), IL-6 and TNF-α, all markers of subclinical inflammation(Reference Tangvarasittichai, Pongthaisong and Tangvarasittichai90–Reference Rodrigues, Pietrani and Bosco92). Various longitudinal studies have also shown that elevated levels of CRP and IL-6 are linked to the later development of type 2 diabetes(Reference Barzilay, Abraham and Heckbert93, Reference Freeman, Norrie and Caslake94).
Inhibition of different inflammatory mediators, including NF-κB, IL-1β, VEGF and cyclo-oxygenase-2 (COX-2), has been shown to limit the degeneration of retinal capillaries characteristic of early-stage diabetic retinopathy(Reference Tang and Kern95). There is evidence to suggest that lutein and zeaxanthin may prevent the development of diabetic retinopathy by suppressing ROS induced by inflammation(Reference Izumi-Nagai, Nagai and Ohgami14). Li et al.(Reference Li, Fung and Fu11) investigated the anti-inflammatory effects of lutein on Muller cells in a murine model. Treatment with lutein led to lower production of the pro-inflammatory factors NF-κB, IL-1β and COX-2, suggesting an anti-inflammatory role for lutein in protection against retinal ischaemic/hypoxic injury(Reference Li, Fung and Fu11). In another study, the protective effects of lutein and zeaxanthin against photo-oxidative damage to RPE cells were investigated. Supplementation with carotenoids modulated the inflammatory responses in these cultured RPE cells in response to photo-oxidation(Reference Bian, Gao and Zhou96).
The effects of zeaxanthin on diabetes-induced retinal oxidative damage and inflammation have also been investigated in diabetic rats(Reference Kowluru, Menon and Gierhart19). It was found that zeaxanthin significantly inhibits diabetes-induced retinal oxidative damage and elevations in VEGF and its adhesion molecule, abnormalities which are commonly associated with the pathogenesis of diabetic retinopathy(Reference Kowluru, Menon and Gierhart19). Clinical findings have highlighted the inverse association between lutein and IL-6 in coronary artery disease patients(Reference Chung, Leanderson and Lundberg97). A recent novel RCT, the Diabetes Visual Function Supplement Study (DiVFuSS)(Reference Chous, Richer and Gerson31), explored the effects of a nutritional supplement (which included lutein and zeaxanthin) on patients with type 1 and type 2 diabetes, and suggested that the preparation used mitigated the damaging effects of systemic inflammation on ocular function, and that these effects may have been mediated by enhancements in MPOD. Chronic low-grade inflammation and oxidative stress co-exist in diabetes, particularly type 2 diabetes but also poorly controlled type 1 diabetes. Therefore, findings from DiVFuSS, which achieved significant increases in MPOD (27 % mean increase in DiVFuSS group v. 2 % mean decrease in placebo group), as well as reductions in serum high-sensitivity CRP (60 % mean decrease in DiVFuSS group v. 11 % mean decrease in placebo group), may be due in part to the inclusion of compounds which, in combination, specifically target both inflammation and oxidative stress, and therefore, which block some of the oxidative and/or inflammatory pathways responsible for the progression of diabetic retinopathy(Reference Chous, Richer and Gerson31). The inclusion of many compounds in this test formula and the fact that both types of diabetic patients (type 1 and 2) were examined renders interpretation of the outcome more difficult; however, these findings are promising as they clearly show that MPOD can be augmented in patients with type 2 diabetes(Reference Chous, Richer and Gerson31).
While it is clear that oxidative stress and inflammation are implicated in the initiation and development of diabetic retinopathy, it remains unclear whether the pathological effects of this oxidative stress/inflammation are mediated through MPOD depletion in type 2 diabetes. The current body of evidence which directly links lower MP levels with other metabolic correlates of diabetes, namely adiposity and dyslipidaemia, will now be explored to elucidate the possible mechanisms by which these metabolic factors mediate their effects on MPOD. It is important to note that both increased adiposity and dyslipidaemia (primarily reduced HDL) may adversely affect MP by compromising the availability(Reference Johnson, Hammond and Yeum98–Reference Nolan, O’Donovan and Kavanagh101), and transport(Reference Connor, Duell and Kean102, Reference Wang, Connor and Johnson103), of dietary carotenoids to the retina. It follows that a condition such as type 2 diabetes, which unfavourably affects these parameters, may have deleterious effects on MPOD, with clinically relevant outcome effects. An analysis of the literature is presented describing these putative mechanisms: (a) evidence in relation to MPOD and body fat/adiposity (n 13; Table 3); and (b) evidence concerning MPOD and dyslipidaemia (n 7; Table 4).
Type 2 diabetes, macular pigment optical density and adiposity: the evidence
Diabetes mellitus is a chronic disorder which can alter carbohydrate, protein and fat metabolism. Weight gain, following excessive energy intake, results in the body becoming markedly resistant to the action of insulin. Despite initial impairment in insulin action, glucose tolerance can remain normal for some time because there is a compensatory increase in insulin secretion by pancreatic β-cells, which results in a well-compensated metabolic state, a condition known as hyperinsulinaemia. There is now mounting evidence to indicate that persistently elevated plasma insulin levels can contribute to the development of hypertension, plasma lipid abnormalities and atherosclerosis(Reference Boden104). The escalating rates of insulin secretion as a result of advancing obesity, however, cannot be maintained and type 2 diabetes ensues.
Intra-abdominal fat appears to be an important determinant of insulin sensitivity compared with subcutaneous fat(Reference Nieves, Cnop and Retzlaff105). Visceral fat and subcutaneous fat differ in their phenotypic, physiological and functional aspects, as there are a greater number of macrophages, T lymphocytes and pro-inflammatory molecules in visceral v. subcutaneous fat in obese individuals. Abdominal adipose tissue produces a variety of adipocytokines including IL-1, IL-6, IL-8, TNF-α, leptin and resistin; therefore, the abdominal adipose mass acts as an important mediator of inflammation in diabetes(Reference Castro, Macedo-de la Concha and Pantoja-Meléndez106). Obesity also leads to an increased size of adipocytes. The death of adipocytes, which is rare in healthy individuals, is very common in obese individuals and has been linked to adipocyte hypoxia. The accumulation of macrophages in the adipose tissue of obese individuals not only eliminates the dead cells but also further increases their synthesis of inflammatory mediators IL-8, IL-6, IL-1 and TNF-α(Reference Castro, Macedo-de la Concha and Pantoja-Meléndez106). The resultant inflammation becomes chronic; therefore, obesity is considered a low-grade inflammatory condition which in turn leads to an increase in oxidative stress. Serum 8-hydroxy guanosine (8-OhdG), a known sensitive marker of oxidative DNA damage and of total systemic oxidative stress in vivo, has been shown to be positively correlated with BMI in individuals with type 2 diabetes mellitus(Reference Al-Aubaidy and Jelinek107). Over-expression of oxidative stress, and the concomitant reduction in antioxidant defence, damages cellular structures(Reference Savini, Catani and Evangelista108), often resulting in further inflammatory responses. Antioxidant defences may be lower in overweight/type 2 diabetic patients, due to their lower intake of antioxidant-rich foods (for example, fruits and vegetables), their increased utilisation of these molecules (for example, increased inflammation/ROS in insulin-dependent tissue such as the retina) and their impaired generation of other supportive antioxidants(Reference Savini, Catani and Evangelista108), factors which may collectively lead to MPOD depletion.
The diminished response to insulin in type 2 diabetes also leads to increased lipolysis in adipocytes. Enlarged adipocytes release NEFA and adipocytokines(Reference Matough, Budin and Hamid67, Reference Boden104); therefore greater amounts of NEFA reach the liver via the portal vein, resulting in fatty liver infiltration. Mobilised NEFA are oxidised by vascular endothelium to generate ROS. Elevations of NEFA in serum also inhibit insulin suppression of hepatic glucose production, leading to an increase in glucose production by the liver(Reference Reaven, Hollenbeck and Jeng109). This, in turn, results in both insulin resistance and inflammation in the major insulin target tissues (skeletal muscle, liver and endothelial cells). Abnormal levels of lipids, fatty acids and various adipocytokines from adipose tissue therefore initiate a vicious cycle of fat damage, inflammation, worsening insulin resistance, impaired β-cell insulin secretion and ultimately type 2 diabetes(Reference Boden104, Reference Karpe, Dickmann and Frayn110). The chronic low-grade inflammation associated with obesity and type 2 diabetes leads to increased oxidative stress and further inflammation, putting a greater demand on antioxidant defences.
Apart from its pro-inflammatory and pro-oxidant effects, adipose tissue is also a major body store for carotenoids such as lutein and zeaxanthin. Higher levels of body fat have been shown to be related to lower levels of circulating carotenoids(Reference Burke, Curran-Celentano and Wenzel111), making these pigments less available to retinal tissue(Reference Gruber, Chappell and Millen112). Furthermore, a randomised controlled weight loss trial found that a significant weight loss in the intervention group was related to increases in serum lutein(Reference Kirby, Beatty and Stack113). Higher BMI(Reference Seddon, Cote and Davis114) and higher body fat percentage(Reference Nolan, O’Donovan and Kavanagh101) have both been linked with an increased risk of AMD. Studies have reported an inverse relationship between adiposity and BMI and MPOD(Reference Hammond, Ciulla and Snodderly99, Reference Nolan, Feeney and Kenny115), with the observed relationships largely attributable to participants with a BMI > 29 kg/m2(Reference Hammond, Ciulla and Snodderly99). One study found that higher body fat percentage, even within relatively healthy limits, was associated with lower retinal tissue lutein and zeaxanthin status(Reference Bovier, Lewis and Hammond116), suggesting that adiposity may affect the nutrient status of the retina. Sex differences in MPOD have also been reported(Reference Johnson, Hammond and Yeum98, Reference Nolan, O’Donovan and Kavanagh101), which may imply differences in metabolism of MP, or reflect differences in adipose tissue storage of these dietary carotenoids between men and women. Competitive absorption by a larger overall fat mass (percentage fat mass is generally higher in women)(Reference Blaak117), or specifically by greater abdominal fat mass (which is more common in men)(Reference Björntorp118), may reduce lutein and zeaxanthin uptake by the retina. Concentrations of carotenoids differ according to body site, with levels demonstrably higher in abdominal fat(Reference Chung, Ferreira and Epstein119), which may explain the differences in MPOD currently observed between men and women(Reference Johnson, Hammond and Yeum98, Reference Nolan, O’Donovan and Kavanagh101). Since adipose tissue may trap lutein and zeaxanthin(Reference Bovier, Lewis and Hammond116), individuals who are overweight or obese (type 2 diabetes) may consequently have lower concentrations of the carotenoids lutein and zeaxanthin available at the macula.
Overall, the research appears to suggest that obesity has a negative impact on MPOD; however, there is also some evidence to the contrary. One study found that mean MPOD values did not differ significantly between subjects with various types of obesity and ideal-weight subjects(Reference Gupta, Raman and Biswas120). Our previous study(Reference Scanlon, Connell and Ratzlaff28) is the only one to date which has examined the relationship between BMI and MPOD in a group with diabetes. This study found that MPOD was significantly lower and BMI was significantly higher in patients with type 2 diabetes, compared with type 1 and non-diabetic controls, highlighting the possibility that different relationships exist between MPOD status and type 1 and 2 diabetes. The higher BMI levels observed in type 2 diabetes are reflective of excess adiposity which acts as a store for lutein and zeaxanthin. However, as described previously, obesity is also independently associated with increased oxidative stress(Reference Savini, Catani and Evangelista108, Reference Fernández-Sánchez, Madrigal-Santillan and Bautista121, Reference Bondia-Pons, Ryan and Martinez122), and with inflammation(Reference Kwon and Pessin123) which exacerbates oxidative stress, and may therefore have multiple depletive effects on MP. Unfortunately, the cross-sectional nature of this study(Reference Scanlon, Connell and Ratzlaff28) means that it cannot elucidate the extent or nature of any causal relationships between BMI and MPOD in these type 2 subjects.
The different presenting features of type 1 and 2 diabetes, and the interplay of their disease components with MPOD status (for example, adiposity), may mean that the mechanistic relationship between these conditions and MPOD status is not uniform across both. The fact that much of the research to date includes both diabetic groups may obfuscate any relationships present. Although the evidence to date is somewhat conflicting, there appears to be a theoretical basis for a relationship between the characteristic body fatness of type 2 diabetes and lower levels of MPOD. A summary of the studies investigating the association between MPOD and adiposity are outlined in Table 3(Reference Scanlon, Connell and Ratzlaff28, Reference Mares, LaRowe and Snodderly29, Reference Johnson, Hammond and Yeum98, Reference Hammond, Ciulla and Snodderly99, Reference Nolan, O’Donovan and Kavanagh101, Reference Kirby, Beatty and Stack113, Reference Nolan, Feeney and Kenny115, Reference Bovier, Lewis and Hammond116, Reference Gupta, Raman and Biswas120, Reference Broekmans, Berendschot and Klopping-Ketelaars124–Reference Edwards, Walk and Cannavale127).
Type 2 diabetes, macular pigment optical density and dyslipidaemia: the evidence
Insulin resistance and obesity, metabolic abnormalities commonly associated with type 2 diabetes, may themselves lead to disturbances in the production and clearance of plasma lipoproteins(Reference Goldberg128). Dyslipidaemia is often characterised by low levels of HDL and hypertriacylglycerolaemia. In addition, LDL particles are converted to smaller, more atherogenic lipoproteins(Reference Krauss and Siri129). A number of factors are likely to be responsible for diabetic dyslipidaemia and these include insulin’s effects on liver apoprotein production, dysregulation of lipoprotein lipase (LPL), impaired action of cholesteryl ester transfer protein and the peripheral action of insulin on adipose and muscle(Reference Goldberg128). Hormone-sensitive lipase and LPL are two enzymes that regulate mobilisation and deposition of fatty acids in adipose tissue in a reciprocal manner(Reference Frayn, Coppack and Fielding130). Reduced insulin action leads to increased lipolysis in adipocytes with increased NEFA release, which can mediate many adverse metabolic effects, most notably further insulin resistance(Reference Karpe, Dickmann and Frayn110) and dyslipidaemia(Reference Goldberg128). In diabetes greater amounts of fatty acids returning to the liver are reassembled into TAG and secreted in VLDL(Reference Ginsberg and Illingworth131). In the presence of increased concentrations of VLDL in circulation, cholesteryl ester transfer protein will exchange VLDL TAG for cholesteryl ester in the core of HDL, which results in smaller HDL particles, which are more rapidly cleared from plasma(Reference Horowitz, Goldberg and Merab132). This explains the hypertriacylglycerolaemia and reduced HDL commonly observed in type 2 diabetes(Reference Goldberg128). Cholesteryl ester transfer protein also exchanges VLDL TAG for cholesteryl ester in the core of LDL and the net effect is a decrease in the size and an increase in the density of LDL particles(Reference Goldberg128). In vitro, small dense LDL can be oxidised more easily, and it binds to LDL receptors less avidly than normal LDL. As a result, diabetic patients are at an increased risk of CVD. Many clinicians measure LDL density and/or size to predict risk of CVD. An increase in waist circumference and waist:hip ratio has been found to be associated with small dense LDL particles(Reference Goldberg128, Reference Campos, Willett and Peterson133). Therefore, intra-abdominal fat is a critical determinant of an atherogenic lipoprotein profile, including disruptions in the distribution of cholesterol in the different lipoprotein fractions(Reference Nieves, Cnop and Retzlaff105).
The characteristic lipid profile in an individual with type 2 diabetes (increased serum VLDL, increased TAG, decreased serum HDL and less commonly, an increase in LDL-cholesterol)(Reference Goldberg128) may have important implications for MP levels in the eye, as studies have shown that the carotenoids lutein and zeaxanthin are primarily transported by HDL in plasma(Reference Connor, Duell and Kean102, Reference Wang, Connor and Johnson103). HDL is a known ligand for scavenger receptor B type 1 (SR-B1), and this suggests a ‘piggy-back’ mechanism of lutein and zeaxanthin uptake into the retina via RPE cells which is mediated by SR-B1(Reference During, Doraiswamy and Harrison134). This hypothesis is supported by evidence from lutein deficiencies observed in the retina of chickens with a genetic HDL defect but not in other organs, implying a major role for HDL in the receptor-mediated transport of lutein to the eye(Reference Connor, Duell and Kean102). It has been argued that the subspecies of HDL containing apoE supplies lipids and lipid-soluble lutein and zeaxanthin to the retina, and that by increasing HDL, retinal lutein levels may be simultaneously augmented(Reference Thomson, Toyoda and Langner100).
n-3 Long-chain PUFA supplementation may also lead to increases in serum HDL(Reference Thomas, Smith and Donahue135). Recent epidemiological studies indicate that increased dietary long-chain-PUFA intakes enhance MPOD(Reference Delyfer, Buaud and Korobelnik136), and that DHA facilitates the accumulation of lutein in the blood and macula. The mechanism by which DHA increases MPOD may relate to its effect on the transport and uptake of lutein into the macula by mediating changes in lipoprotein levels(Reference Johnson, Chung and Caldarella137). Putatively, this effect could also be mediated by the anti-inflammatory effects of DHA, but this is conjectural and no direct evidence supports this effect within the retina.
One observational study found that the correlation between MPOD, serum lutein, zeaxanthin and lipids differed depending on the age profile of participants(Reference Olmedilla-Alonso, Beltrán-de-Miguel and Estévez-Santiago138). In younger adults, MPOD was found to be influenced by serum lutein, whereas in older adults, circulating lipids were an added determining factor(Reference Olmedilla-Alonso, Beltrán-de-Miguel and Estévez-Santiago138). Mares et al.(Reference Mares, LaRowe and Snodderly29) queried whether any association with serum lipids is truly strong enough to play a meaningful role in the pathogenesis of MPOD depletion. One study which used a diabetic cohort, involving a small group of forty-three participants (fourteen controls, seventeen with diabetes but without retinopathy and twelve with diabetes and with mild non-proliferative retinopathy), found no significant correlation between MPOD and serum lipid levels(Reference Lima, Rosen and Maia16). Our own research group(Reference Scanlon, Connell and Ratzlaff28), however, reported lower levels of MPOD in type 2 v. type 1 diabetics and noted significantly lower HDL values within the type 2 group, raising the possibility that lower HDL levels may have contributed to this difference. More recently, the DiVFuSS study(Reference Chous, Richer and Gerson31) has demonstrated significant improvements in serum LDL-cholesterol level (9 % mean decrease in the intervention group v. 1 % mean increase in placebo group), HDL-cholesterol (7 % mean increase in the intervention group v. 3 % decrease in placebo group) and TAG (8·6 % mean decrease in the intervention group v. 2 % mean increase in placebo group), suggesting a positive effect of the study formulation on serum lipids. Whilst this effect was probably attributable to other non-carotenoid components of the supplement, the synchronous increase in MPOD observed in the intervention group may have been partially mediated by these favourable changes in lipid profile and their consequential impact on carotenoid transport to the retina(Reference Connor, Duell and Kean102, Reference Wang, Connor and Johnson103, Reference Loane, Nolan and Beatty139). In the broader context, the evidence from this study is sufficiently robust to prompt further lines of investigation into the role of MP supplementation either in isolation or as a multi-component supplement in diabetes. The research to date, however, is not conclusive and it remains unclear from the limited amount of observational and RCT data whether serum lipids (primarily HDL) play a meaningful role in determining MPOD status. Given the theoretical basis for an association between lipid profile and MP status, and the fact that lipid profile is often altered deleteriously in diabetes, further investigation is warranted. The relationship between dyslipidaemia and lower levels of MP is outlined in Table 4(Reference Lima, Rosen and Maia16, Reference Mares, LaRowe and Snodderly29, Reference Chous, Richer and Gerson31, Reference Olmedilla-Alonso, Beltrán-de-Miguel and Estévez-Santiago138–Reference Nagai, Izumi-Nagai and Suzuki141).
Causal mechanisms and metabolic perturbations associated with lower macular pigment in diabetes
The candidate causal mechanisms explored herein as contributors to lower MPOD levels in diabetes relate primarily to type 2 diabetes (or poorly controlled type 1). The complex inter-relationship between oxidative stress/inflammation and the metabolic correlates of diabetes (adiposity, dyslipidaemia, insulin resistance, hyperinsulinaemia and hyperglycaemia), and their associations with MPOD, both individually and as a whole, represent a significant scientific challenge. In summary, our analysis indicates that MPOD might be depleted through at least four causal mechanisms previously described, all of which are outlined in Fig. 2.

Fig. 2. Metabolic perturbations and supply deficiencies contributing to macular pigment depletion in diabetes. MPOD, macular pigment optical density; ROS, reactive oxygen species. For a colour figure, see the online version of the paper.
Dietary determinants of macular pigment optical density in diabetes
First and foremost, patients with type 2 diabetes may experience lower levels of MP as a result of poor dietary intake of antioxidant nutrients (lutein, zeaxanthin and meso-zeaxanthin). Lutein and zeaxanthin are not synthesised de novo in humans; therefore, a diet rich in fruit and vegetables is necessary to enhance MP levels in the eye(Reference Sommerburg, Keunen and Bird4). While the exact cause of diabetes is still not fully understood, dietary factors have garnered particular attention over the last number of years. In fact, some research indicates that up to 74 % of type 2 diabetes cases are directly attributable to obesity(Reference Janssen142).
Many studies have reported a positive association between a high intake of sugars and the development of type 2 diabetes(Reference Gross, Li and Ford143–Reference Elliott, Keim and Stern145). Research has also found an association between high fat intakes and type 2 diabetes and impaired glucose tolerance(Reference Marshall, Hamman and Baxter146, Reference Saldana, Siega-Riz and Adair147), with some literature suggesting that high-fat, low-carbohydrate diets are associated with the onset of type 2 diabetes(Reference Marshall, Hamman and Baxter146). A global change in dietary intake has occurred over recent decades resulting from an increased use of sweeteners such as fructose and sucrose by the food industry(Reference Nseir, Nassar and Assy148). Recent evidence suggests a causal link between the high intake of sugar-sweetened soft drinks and obesity and diabetes. This is thought to arise from the large amount of fructose in these soft drinks, which impairs insulin sensitivity and raises blood glucose levels and BMI to dangerous levels(Reference Bray, Nielsen and Popkin144, Reference Raben, Vasilaras and Møller149). Fructose consumption increases postprandial TAG concentrations within hours(Reference Swarbrick, Stanhope and Elliott150), which suggests that postprandial hypertriacylglycerolaemia is one of the earliest metabolic perturbations associated with fructose consumption. The most likely mechanism for this hypertriacylglycerolaemia is increased hepatic de novo lipogenesis, which in turn up-regulates VLDL production and secretion(Reference Stanhope and Havel151), factors which have a knock-on suppressive effect on HDL, a known transporter of carotenoids to the eye(Reference Connor, Duell and Kean102, Reference Wang, Connor and Johnson103).
Studies have also found an inverse correlation between the intake of green leafy vegetables and risk of developing type 2 diabetes(Reference Wang, Fang and Gao152, Reference Li, Fan and Zhang153), and that the consumption of fruits and vegetables may protect against the development of type 2 diabetes, as they are rich in micronutrients, fibre and antioxidants(Reference Li, Fan and Zhang153), dietary components also known to enhance MPOD. A recent meta-analysis revealed that a higher intake of fruit, especially berries and green leafy vegetables, yellow vegetables and cruciferous vegetables or their fibre, is associated with a lower risk of type 2 diabetes(Reference Wang, Fang and Gao152). Health benefits have also been observed with the Mediterranean diet(Reference Tsoupras, Lordan and Zabetakis154, Reference Romagnolo and Selmin155), a diet which contains an abundance of fruit and vegetables. While the beneficial effects of fruits and vegetables on diabetes risk are likely to be multiple, these foods are the primary dietary source of carotenoids including lutein and zeaxanthin, highlighting their consumption as a key determinant of MPOD. Lower levels of MPOD in diabetes might also be associated with high intakes of fried foods and fat(Reference Guallar-Castillón, Rodriguez-Artalejo and Fornes156), alcohol(Reference Traversy and Chaput157), red and processed meat(Reference Wang and Beydoun158), sugar-sweetened beverages(Reference Gross, Li and Ford143, Reference Bray, Nielsen and Popkin144) and high-glycaemic index foods(Reference Ludwig, Majzoub and Al-Zahrani159), all of which have been linked with higher levels of visceral fat and increased waist circumference. Numerous studies have shown that obesity (in particular, central obesity) can increase the risk of type 2 diabetes(Reference Mokdad, Ford and Bowman160).
While the body’s natural defence against oxidative damage and inflammation is neutralisation by endogenous antioxidants (enzymic and non-enzymic), in association with exogenous antioxidants (vitamin C, vitamin E, carotenoids, β-carotene, lutein, zeaxanthin and meso-zeaxanthin)(Reference Bouayed and Bohn50, Reference Cabrera and Chihuailaf68), these antioxidant defences may be compromised in diabetes. Hence, it is clear that both over-utilisation and under-supply of antioxidant nutrients may contribute to lower levels of MP in the diabetic retina.
Based on the outlined associations between nutritional and dietary factors and the components of the metabolic syndrome which may contribute to MPOD depletion, we outline dietary factors which: (1) may exacerbate the metabolic correlates associated with type 2 diabetes (Table 5 column a); and (2) may help to prevent or resolve the metabolic perturbations associated with type 2 diabetes (Table 5 column b).
Table 5. Dietary factors associated with the metabolic correlates of type 2 diabetes mellitus

FA, fatty acids.
Overall, Western dietary habits are a significant factor in the development of the metabolic syndrome. The continuous over-provision of energy via dietary carbohydrate and lipid, when unmatched by physical activity-induced energy expenditure, leads to a state of excess adiposity, chronic low-grade inflammation, and oxidative stress and dyslipidaemia; features associated with type 2 diabetes and which may lead to MPOD depletion.
The importance of diet cannot be overemphasised in diabetes management. Given the variety of mechanisms which may contribute to MPOD depletion and the broader ocular risks associated with such MPOD depletion in diabetes, a comprehensive dietary approach is therefore necessary to optimise ocular health and MPOD status. The generous provision of brightly coloured fruits and vegetables which are rich in a broad range of antioxidants including the target carotenoids lutein, zeaxanthin and meso-zeaxanthin represents an obvious recommendation. This, however, should be complemented by the inclusion of wholegrain-fortified breakfast cereals for their folate, vitamin D and anti-obesogenic fibre content; and with plenty of oily fish to provide further vitamin D and n-3 essential fatty acids to attenuate inflammation and correct or prevent hypertriacylglycerolaemia. These foods should be used to displace those which are high in fat, saturated fat, refined sugar and fructose; for example, oily fish should replace red or processed meats at several main meals each week, fruit or raw vegetables should be used to replace snack foods high in fat, saturated fat, trans-fat and refined sugar (for example, baked goods and confectionery), and low-fat fortified milk should replace sugar-sweetened soft drinks. Starchy carbohydrates should be evenly distributed over the day to achieve better glycaemic control, with sterol- or stanol-enriched products used to reduce serum LDL and consequently enhance LDL:HDL ratio. Finally, from a broader lifestyle perspective, these individuals should be advised to moderate their alcohol consumption to no more than two standard drinks per d with several alcohol-free days each week, and to engage in moderate-intensity exercise (for example, walking) on most days of the week to enhance their insulin sensitivity and optimise their blood glucose and lipoprotein profiles. Table 5 (column a) outlines the dietary factors causally associated with the metabolic correlates of type 2 diabetes, and Table 5 (column b) outlines the dietary factors associated with protection against the metabolic perturbations of type 2 diabetes.
Conclusion
The present review outlines our current understanding of the relationship between diabetes and MPOD. MP appears to be generally lower in diabetic populations compared with healthy controls(Reference Lima, Rosen and Maia16), and this is noted particularly amongst patients with type 2 diabetes(Reference Scanlon, Connell and Ratzlaff28). Impaired defence against reactive oxidative species (ROS) at the retina may not only be attributable to lower intake of foods rich in carotenoids and other antioxidants (for example, fruit, vegetables, legumes), however, but may also arise from the increased utilisation of these antioxidants in diabetes (via chronic inflammation and oxidative stress). Adiposity, particularly abdominal fatness, is closely linked to type 2 diabetes, and is a significant factor in the condition’s pathogenesis. Excess fat tissue may not only compete with the retina for uptake of dietary carotenoids, but may also contribute to increased oxidative stress and inflammation, factors which may have a negative impact on MP levels in the eye. Similarly, the hyperglycaemia which is characteristic of diabetes also contributes to inflammation and oxidative stress, and their depletive effects on MPOD. Dyslipidaemia, more common in poorly controlled diabetes, is a metabolic correlate which may adversely affect the transport and assimilation of carotenes into retinal tissue, by limiting the availability of their principal carrier HDL. The chronic low-grade inflammation and increased oxidative stress observed in patients with type 2 diabetes may also increase the use of these carotenoids at the molecular level, and, in so doing, may elevate our dietary requirement for them.
It is important to note the significant limitations which currently prevail in the reviewed literature. The heterogeneity of studies is a major drawback. Small sample sizes, the merging of type 1 and type 2 diabetic patients in statistical analyses, and the complex interplay of diabetes and its accessory factors (adiposity, dyslipidaemia, oxidative stress and inflammation) with MPOD status are also challenging. While there is some preliminary positive evidence, primarily from animal studies(Reference Kowluru, Menon and Gierhart19, Reference Kowluru, Zhong and Santos20) and the DiVFuss trial(Reference Chous, Richer and Gerson31), that higher MP levels may be protective against retinal damage, there is limited evidence that low MPOD levels are detrimental to ocular health in diabetes, and the actual benefits of higher MPOD in terms of retinal disease development and/or progression in diabetes remain to be elucidated.
In view of the limited evidence available, supplementation with lutein, zeaxanthin and/or meso-zeaxanthin, either with or without other antioxidants, represents a fertile area for future interventional research. It is important to recognise, however, that in order to minimise the risk of MPOD depletion and/or ocular damage in diabetes, it is not sufficient to simply supplement with macular carotenoids. Rather, our review suggests the need for a more holistic evaluation of diet and lifestyle in relation to MPOD in diabetes. In this context, inflammation and oxidative stress, the dual pathogenic driving forces of diabetes which occur independent of hyperglycaemia, may be amenable to dietary intervention and nutritional supplementation. The incorporation of a number of dietary modifications (for example, enhanced antioxidant, vitamin D and n-3 fatty acid intake; and reduced fat, saturated fat, trans-fat and refined sugar consumption), alongside lifestyle changes such as increased physical activity, may not only address the under-supply of macular carotenoids, but may also abrogate the competitive storage, transport, inflammatory and oxidative features of type 2 diabetes which may compromise MPOD status. The safety and low cost of such interventions further commend their potential value as a therapeutic adjunct in diabetic eye disease, and may come to represent an essential element of ocular care for this important and expanding patient group.
Acknowledgements
We would like to give special thanks to Kate Loskutova for her help with the graphics in Fig. 2, and to Dr Kate Younger for her review of the draft manuscript.
The present review received no grant from any funding agency, commercial or not-for-profit sectors.
G. S. wrote the paper and has primary responsibility for the final content. J. L. and D. M. helped to draft, evaluate and edit the paper. D. F. helped with the initial drafting and content of the paper.
There are no conflicts of interest.