The escalating epidemic of the metabolic syndromes, including obesity and diabetes, represents one of the most pressing and costly medical challenges in public health of the twenty-first century. A recent study has reported that 336 million people had diabetes in 2011, and this number is expected to rise to 552 million by 2030( Reference Whiting, Guariguata and Weil 1 ). Diabetes is recognised by hyperglycaemia and glucose intolerance due to insulin deficiency, impaired insulin sensitivity or both. Type 2 diabetes mellitus (T2DM) is predominant and accounts for 90 % of patients with diabetes( Reference Stumvoll, Goldstein and van Haeften 2 ). It is well established that diabetes has been associated with slowly progressing brain damage that impaired cognitive function( Reference Manschot, Brands and van der Grond 3 – Reference Qiu, Sigurdsson and Zhang 5 ). Although there is some evidence for a relationship between T2DM and brain damage, the mechanisms driving it remain unknown.
Clinical studies have indicated that cognitive dysfunction is correlated with brain atrophy in patients with diabetes( Reference Manschot, Brands and van der Grond 3 , Reference Novak, Zhao and Manor 6 , Reference Moran, Phan and Chen 7 ). Kumar et al. ( Reference Kumar, Haroon and Darwin 8 ) reported smaller total brain grey matter volumes in patients with diabetes, and the decrease is more pronounced in the cortical grey matter of the temporal lobe( Reference Brundel, van den Heuvel and de Bresser 9 ). Despite the limited knowledge on the underlying mechanisms for cognitive dysfunction during the progression of T2DM, mitochondrial dysfunction and oxidative stress have been suggested as major contributors( Reference Zhao, Pan and Shen 10 – Reference Milone 12 ). A previous study has indicated that high glucose concentration, a major pathological characteristic of diabetes, could induce overproduction of superoxide from mitochondrial electron transport chain, which is considered the first and key event involved in the pathogenesis of diabetic and subsequent complications( Reference Ceriello 13 ). Overexpression of Mn superoxide dismutase (SOD)2 protects neurons against hyperglycaemic injury in db/db diabetic mice( Reference Vincent, Russell and Sullivan 14 ). It has also been reported that high glucose concentrations could enhance the formation of advanced glycation end products, which can induce reactive oxygen species (ROS) production from the mitochondria and promote oxidative damage to the heart and brain( Reference Valente, Gella and Fernandez-Busquets 15 , Reference Teshima, Takahashi and Nishio 16 ). Given the importance of the mitochondria as a source of both ATP and ROS, mitochondrial dysfunction has been considered a link between diabetes and neurodegenerative diseases such as Alzheimer's disease( Reference Moreira, Santos and Seica 17 ). While numerous mechanisms regulating mitochondrial function have been delineated, including mitochondrial biogenesis, dynamics, modification and mitophagy, the clear mechanisms accounting for mitochondrial dysfunction during T2DM-associated brain damage remain to be elucidated.
Hydroxytyrosol (HT), a natural polyphenol from virgin olive oil, is considered to be one of the most effective antioxidants. Consumption of HT has certain health benefits, and the responsible mechanisms for these effects have been mainly attributed to its ability to scavenge ROS and enhance endogenous antioxidant systems( Reference Visioli, Bellomo and Galli 18 , Reference Stupans, Kirlich and Tuck 19 ). In our previous studies, we have found that HT could protect retinal epithelial pigment cells and adipocytes against oxidative damage through activating the nuclear transcription factor erythroid 2p45-related factor-2 (Nrf2)/Kelch-like ECH-associated protein 1 pathway( Reference Zhu, Liu and Feng 20 – Reference Liu, Sun and Zhu 22 ) and stimulate mitochondrial biogenesis through PPARγ coactivator-1 (PGC-1)α activation( Reference Zhu, Liu and Feng 20 , Reference Hao, Shen and Yu 23 ). These studies have suggested that HT possesses the ability to reduce oxidative stress and improve mitochondrial function. However, the beneficial effects of HT on diabetic brain damage remain unknown. Thus, because HT was reported to efficiently cross the blood–brain barrier( Reference Wu, Lin and Tsai 24 ), db/db mice, a widely used human T2DM animal model, were used to explore the in vivo neuroprotective effects of HT and its underlying mechanisms.
Experimental methods
Chemicals
An antibody against β-actin was obtained from Sigma. Antibodies against complexes I (NADH dehydrogenase (ubiquinone) Fe-S protein 3; NDUFS3), II (subunit 30 kDa), III (subunit core 2), IV (subunit I) and V (subunit α) were obtained from Invitrogen. Antibodies against AMP-activated protein kinase (AMPK), phospho-AMPK (p-AMPK) and PGC-1 were obtained from Cell Signaling Technology. Antibodies against sirtuin 1 (Sirt1), p62 (sequestosome-1), haeme oxygenase 1 (HO-1), SOD1 and SOD2 were obtained from Santa Cruz Biotech. The BCA Protein Assay kit (Pierce 23225) and enhanced chemiluminescence (ECL) Western blotting detection kit were purchased from Pierce. Nitrocellulose membranes were obtained from PerkinElmer Life Sciences. The TRIzol reagent, oligomycin, carbonyl cyanide 4-(trifluoromethoxy) phenylhydrazone (FCCP), rotenone and compound C were obtained from Invitrogen, and the immunoprecipitation lysis buffer was from Beyotime. HT was purchased from Xi'an APP-Chem Bio(Tech) Company Limited.
Animals and treatments
Male db/db mice aged 4-week-old from a C57BL/6J genetic background were purchased from SLAC Laboratory Animals Company Limited. After 1 week of acclimatisation, mice were randomly divided into the following three groups: db/db mice; db/db mice with a daily oral administration of low-dose HT (10 mg/kg per d); db/db mice with a daily oral administration of high-dose HT (50 mg/kg per d). After 8 weeks of feeding, mice were fasted overnight and killed. All animals were housed in a temperature-controlled (25–27°C) and humidity-controlled (60 %) animal room and maintained on a 12 h light–12 h dark cycle (light from 08.00 to 20.00 hours) with food and water provided during the experiments. All the procedures were performed in accordance with the United States Public Health Services Guide for the Care and Use of Laboratory Animals, and all efforts were made to minimise the suffering and the number of animals used in the present study.
Western blotting
Cortical brain tissue was lysed with Western and immunoprecipitation lysis buffer. Lysates were centrifuged at 13 000 g for 10 min at 4°C. Supernatant protein concentrations were determined with a BCA protein assay kit (Pierce). Equal amounts of protein per sample (20 μg) were subjected to 10 % (w/v) SDS–PAGE; proteins were then transferred to nitrocellulose membranes and blocked with a 5 % (w/v) non-fat milk/TBST (Tris-buffered saline Tween 20) for 1 h at room temperature. Membranes were incubated with primary antibodies against β-actin (1:5000), mitochondrial complex I–V (1:3000), AMPK (1:1000), phospho-AMPK (1:1000), PGC-1 (1:1000), Sirt1 (1:1000), p62 (1:1000), HO-1 (1:1000), SOD1 (1:1000) and SOD2 (1:1000) in 5 % (w/v) milk/TBST at 4°C overnight. Membranes were washed with TBST three times and were then incubated with anti-rabbit or anti-mouse antibodies at room temperature for 1 h. Chemiluminescent detection was performed by an ECL Western blotting detection kit (Pierce) and quantified by scanning densitometry.
Real-time PCR
Total RNA was extracted from cells using TRIzol reagent according to the manufacturer's instructions. Reverse transcription from RNA to complementary DNA was performed using the PrimeScript RT-PCR Kit (Takara) followed by semiquantitative real-time PCR using gene-specific primers. The following primers were used for the RT-PCR analysis: activity-regulated cytoskeleton-associated protein (Arc), GGTAAGTGCCGAGCTGAGATG (forward) and CGACCTGTGCAACCCTTTC (reverse); N-methyl-d-aspartate receptor 1 (NMDAR1), GCCCAACGCCATACAGATG (forward) and GGCGGGTGACTAACTAGGATAGC (reverse); nerve growth factor (NGF), TTGCCAAGGACGCAGCTTT (forward) and TGCCTGTACGCCGATCAAA (reverse); β-actin, CCACACCTTCTACAATGAGC (forward) and GGTCTCAAACATGATCTGGG (reverse).
Assays for mitochondrial complex activities
Mitochondria were isolated from mouse cortical brain tissue, and mitochondrial protein concentrations were determined using the BCA protein assay kit (Pierce). NADH–ubiquinone reductase (complex I) activities were measured spectrometrically using conventional assays, as described previously( Reference Cao, Xu and Zou 25 ).
Cell viability assay
SH-SY-5Y cells were seeded in ninety-six-well plates at a density of 4 × 104 cells per well for 24 h. These cells were then treated with different concentrations of glucose or HT for the indicated time periods. Then, the number of viable cells was determined by addition of 3-(4,5-dimethylthiazol-2-yl)-2,5-diphenyltetrazolium bromide. Optical densities were read at 550 nm using a microplate spectrophotometer (Multiskan Ascent; Thermo Fisher Scientific, Inc.).
Oxidative status assessment
2′,7′-Dichlorodihydrofluorescein diacetate (2′,7′-dichlorofluorescin diacetate) is a freely permeable fluorogenic tracer used for the assessment of oxidative status. After treatment, cells were incubated with 10 mm-2′,7′-dichlorofluorescin diacetate for 30 min and then washed with PBS three times. Cell lysis was prepared with a lysis solution (10 mm-Tris, 150 mm-NaCl, 0·1 mm-EDTA and 0·5 % Triton X-100, pH 7·5). The supernatant (200 μl) was analysed using a spectrofluorometer with excitation 485 nm and emission 538 nm (Fluoroskan Ascent; Thermo Fisher Scientific, Inc.). An aliquot of supernatant was used in a BCA protein assay to determine the concentration of total protein. ROS levels were expressed as the relative 2,7-dichlorofluorescien (DCF) fluorescence per μg of protein.
VO2
Cell VO2 by intact cells, which is a marker for mitochondrial respiration activity, was measured. Basal, oligomycin and FCCP-treated VO2 rates were investigated after high glucose or HT treatment using Seahorse Extracellular Flux Analyzer (Seahorse Bioscience) according to the manufacturer's instructions.
Statistical analysis
Data are presented as means with their standard errors. The statistical significance of differences among groups were analysed using a one-way ANOVA followed by Dunnett's multiple comparison test. A P value < 0·05 was considered to be statistically significant.
Results
Effects of hydroxytyrosol on the expression and activities of mitochondrial complexes in the db/db mouse brain cortex
The db/db mice exhibited over obesity and hyperglycaemia relative to normal. Comparing with normal C57BL6 mice, no significant changes were observed on the expression of mitochondrial complexes in the db/db mice brain cortex (see online Supplementary Fig. S1). After a 2-month HT supplement in db/db mice, the expression of mitochondrial complexes I, II and IV was significantly improved (Fig. 1(a)–(d)), while the expression of complexes III and V was not affected. We also measured the activities of these complexes and found that the activity of complex I was significantly increased after HT treatment compared with the db/db control group (Fig. 1(e)), while the activities of other complexes were not changed (data not shown).
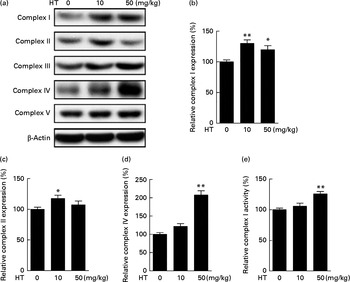
Fig. 1 Effects of hydroxytyrosol (HT) on the expression and activities of mitochondrial complexes in the db/db mouse brain cortex. After 8 weeks of treatment with HT, mice were killed for brain protein and mitochondrial isolation. Protein expression levels of brain mitochondrial complex subunits were determined by the following Western blotting analysis: Western blot image (a), statistical analysis of complex I subunit (b), complex II subunit (c) and complex IV subunit (d) levels. Mitochondrial respiratory chain complex I activity was analysed spectrometrically (e). Expression of each protein was normalised to the β-actin loading control. Values are means, with their standard errors represented by vertical bars (n 8). Mean value was significantly different from that of control group: * P< 0·05, ** P< 0·01.
Hydroxytyrosol induced phase II antioxidant systems in the brain of db/db mice
The induction of phase II enzymes is another effect of HT in our previous in vitro studies. In the present study, we found that the phase II antioxidant enzymes, including HO-1 (Fig. 2(a) and (c)), SOD1 (Fig. 2(a) and (d)) and SOD2 (Fig. 2(a) and (e)), were significantly increased by HT treatment. Since SOD2 was found markedly decreased in db/db mice compared with C57BL6 control mice (see online Supplementary Fig. S2), the major effect of HT on SOD content in the db/db mice was assumed to sufficiently prevent the decrease in SOD2. A previous study has indicated that p62 can activate Nrf2, a key regulator of phase II enzymes, and induce antioxidant proteins and detoxification enzymes( Reference Komatsu, Kurokawa and Waguri 26 ). Thus, we measured p62 protein expression and found a significant increase in p62 expression induced after HT treatment (Fig. 2(a) and (b)).
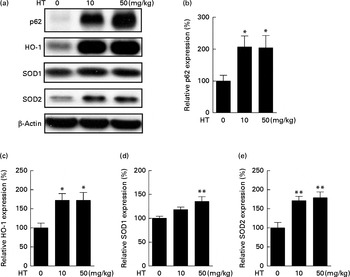
Fig. 2 Effects of hydroxytyrosol (HT) on the phase II antioxidant system in the brain of db/db mice. After 8 weeks of treatment with HT, mice were killed, and the brain tissues were collected. The activation of the phase II antioxidant system was detected by the following Western blotting analysis: Western blot image (a), statistical analysis of p62 (b), haeme oxygenase 1 (HO-1) (c), superoxide dismutase (SOD)1 (d) and SOD2 (e). Expression of each protein was normalised to β-actin loading controls. Values are means, with their standard errors represented by vertical bars (n 8). Mean value was significantly different from that of control group:* P< 0·05, ** P< 0·01.
Hydroxytyrosol inhibited protein oxidation in the brain of db/db mice
Protein carbonyl content is an indicator of protein peroxidation. As shown in Fig. 3, protein oxidation was effectively decreased by both low and high doses of HT supplements, suggesting that HT may contribute to reduce oxidative stress in the brain of db/db mice.
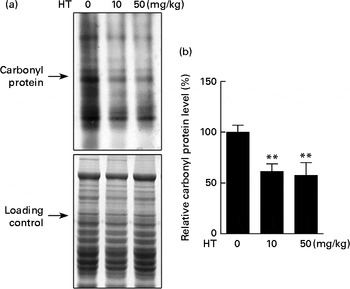
Fig. 3 Effects of hydroxytyrosol (HT) on protein oxidation in the brain of db/db mice. After 8 weeks of treatment with HT, mice were killed, and the brain tissues were collected. The carbonyl protein content was analysed as an indicator of protein oxidation. (a) Western blotting image and (b) statistical analysis is shown. Expression of protein was normalised to the total protein content loading controls. Values are means, with their standard errors represented by vertical bars (n 8). ** Mean value was significantly different from that of control group (P< 0·01).
Hydroxytyrosol activated AMP-activated protein kinase pathway in the brain of db/db mice
The AMPK/Sirt1/PGC-1 pathway has been suggested as a mechanism for controlling mitochondrial function( Reference Lira, Brown and Lira 27 , Reference Chau, Gao and Yang 28 ) and Nrf2-regulated antioxidative enzymes( Reference Mo, Wang and Zhang 29 ). Hence, we detected the AMPK/Sirt1/PGC-1 pathway in the brain of db/db mice. As expected, the expression of p-AMPK/AMPK (Fig. 4(a) and (b)), PGC-1 (Fig. 4(c) and (d)) and Sirt1 (Fig. 4(e) and (f)) levels were all significantly increased by both low and high doses of HT supplements, suggesting that HT activated the AMPK/Sirt1/PGC-1 pathway in the brain of db/db mice.
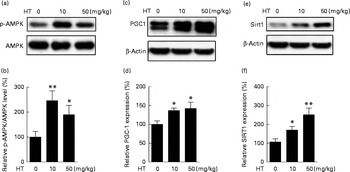
Fig. 4 Effects of hydroxytyrosol (HT) on the AMP-activated protein kinase (AMPK) pathway in the brain of db/db mice. After 8 weeks of HT treatment, mice were killed and brain proteins were isolated for Western blotting analysis. The expression of phospho-AMPK (p-AMPK)/AMPK ((a) Western blot image, (b) statistical analysis), PPARγ coactivator-1 (PGC-1) ((c) Western blot image, (d) statistical analysis) and sirtuin 1 (Sirt1) ((e) Western blot image, (f) statistical analysis) were tested. The expression of each protein was adjusted to AMPK or the β-actin loading control. Values are means, with their standard errors represented by vertical bars (n 8). Mean value was significantly different from that of control group: * P< 0·05, ** P< 0·01.
Hydroxytyrosol improved neuronal survival of db/db mice
Previous study has reported that neuron loss was observed in the dorsal root ganglia of db/db mice( Reference Shi, Zhang and Zeberg 30 ). In the present study, neuron loss was observed in the brain cortex of db/db mice compared with normal C57BL6 control mice evidenced by decreased neuron factors including Arc, NMDAR1 and NGF (see online Supplementary Fig. S3). HT supplements, especially the high dose, significantly increased the mRNA levels of Arc, NMDAR1 and NGF compared with the db/db control mice, suggesting that HT improved neuronal cell survival in the brain of db/db mice (Fig. 5(a)–(c)).
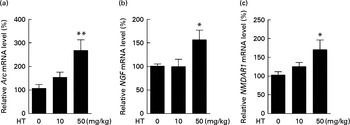
Fig. 5 Effects of hydroxytyrosol (HT) on the mRNA expression of neuronal survival biomarkers in the brain of db/db mice. After 8 weeks of treatment with HT, mice were killed and total RNA was isolated. The following mRNA expression levels of neuronal factors were determined by real-time PCR as an indication of neuron survival: (a) activity-regulated cytoskeleton-associated protein (Arc) mRNA levels; (b) N-methyl-d-aspartate receptor 1 (NMDAR1) levels; (c) nerve growth factor (NGF) levels. Values are means, with their standard errors represented by vertical bars (n 8). Mean value was significantly different from that of control group: * P< 0·05, ** P< 0·01.
Hydroxytyrosol protected against high glucose-induced SH-SY-5Y neuronal cell damage
Hyperglycaemia is a major factor for the complications derived from diabetes. Therefore, a toxicity model induced by high glucose in SH-SY-5Y cells was used to confirm the possible underlying mechanism of HT's neuroprotective effects in vitro. Similar to a previous report( Reference Kapoor and Kakkar 31 ), high levels of glucose (45 and 175 mm) inhibited cell viability in a time-dependent manner within 24 h (Fig. 6(a)). Consistent with previous studies( Reference Hsu, Tseng and Lo 32 – Reference Russell, Golovoy and Vincent 35 ), increased DCF fluorescence were observed 3 h after treatment with 45 mm-glucose and plateaued after 6–24 h (Fig. 6(b)). Meanwhile, pre-treatments with 2, 5 and 10 μm-HT sufficiently increased cell viability after a 45 mm-glucose challenge (Fig. 6(c)). Although we have previously reported that HT could be efficiently uptake in mice and reached 16 μmol/l (2·5 μg/ml) at 5 min in serum after 50 mg/kg administration( Reference Cao, Xu and Zou 25 ), we also measured HT in culture medium with or without SH-SY-5Y cells, data suggested that HT could efficiently uptake in the cells (see online Supplementary Fig. S4). In addition, we analysed basal and uncoupled mitochondrial VO2 capacity with Seahorse analyzer (Seahorse Bioscience) (see online Supplementary Fig. S5). Normalised data indicated that the basal VO2 capacity, ATP production potential and maximal respiration were all significantly decreased by a 45 mm-glucose treatment, while basal and maximal respiration were efficiently protected by a 10 μm-HT pre-treatment (Fig. 6(d)).
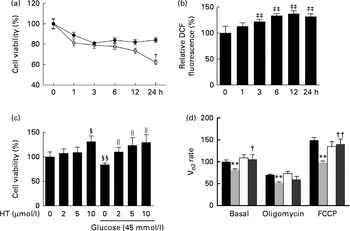
Fig. 6 Effects of hydroxytyrosol (HT) on high glucose-induced neuronal cell damage. (a) SH-SY-5Y cells were treated with glucose at the indicated concentrations (45 mm (
) and 175 mm (
)) for 1, 3, 6, 12 or 24 h, and cell viability was detected by the MTT (3-(4,5-dimethylthiazol-2-yl)-2,5-diphenyltetrazolium bromide) method. (b) SH-SY-5Y cells were treated with 45 mm-glucose for 1, 3, 6, 12 or 24 h, and oxidative status was assessed with a 2,7-dichlorofluorescien diacetate (DCF-DA) assay. (c) SH-SY-5Y cells were pretreated with the indicated concentrations of HT (2, 5 and 10 μm) for 24 h, followed by a 6 h treatment with 45 mm-glucose, and cell viability was then detected by the MTT method. (d) SH-SY-5Y cells were pretreated with 10 μm-HT for 24 h, followed by a 6 h treatment with 45 mm-glucose, and the basal, oligomycin-treated and carbonyl cyanide 4-(trifluoromethoxy) phenylhydrazone (FCCP) uncoupled cell VO2 capacity was detected with a Seahorse analyzer (Seahorse Bioscience).
, Control;
, glucose 45;
, HT,
, HT+glucose 45. Values are means, with standard errors represented by vertical bars (n 3). ** Mean value was significantly different from that for control (P< 0·01). Mean value was significantly different from that for the 45 mm-glucose treatment: † P< 0·05, †† P< 0·01. ‡‡ Mean value was significantly different from that at time zero (P< 0·01). Mean value was significantly different from that for the no-glucose, no-HT treatment: § P< 0·05, §§ P< 0·01. ∥ Mean value was significantly different from that for the 45 mm-glucose, no-HT treatment (P< 0·05).
Hydroxytyrosol prevented high glucose-induced SH-SY-5Y cellular damage through AMP-activated protein kinase activation
Time-dependent treatment of SH-SY-5Y cells with 10 μm-HT increased p-AMPK levels at 10 and 30 min, which then gradually decreased, suggesting that HT could rapidly activate the AMPK pathway (Fig. 7(a) and (b)). Meanwhile, HT treatment significantly increased the protein expression of mitochondrial complex IV and HO-1, and the induction effect was abolished by AMPK-specific inhibitor compound C (Fig. 7(c) and (d)). Although HT showed no significant induction on SOD2 protein expression, the inhibition of AMPK sufficiently reduced its content (Fig. 7(c) and (d)), suggesting the major regulatory effect of AMPK pathway on mitochondrial and antioxidative protein expression. In addition, 45 mm-glucose treatment significantly decreased the levels of p-AMPK, while 10 μm-HT pre-treatment effectively restored the levels of p-AMPK (Fig. 8(a) and (b)), and the protective effects of HT against high glucose-induced cell toxicity were abolished when activation of AMPK was inhibited by compound C (Fig. 8(c)), indicating that AMPK pathway activation contributed to HT's protective effect.
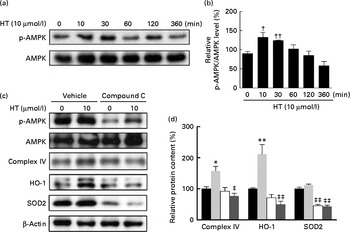
Fig. 7 Effects of hydroxytyrosol (HT) on AMP-activated protein kinase (AMPK) activation with and without glucose treatment. SH-SY-5Y cells were treated with 10 μm-HT for 10, 30, 60, 120, 360 min without glucose treatment, and phospho-AMPK (p-AMPK) was detected by Western blotting: (a) Western blotting image, (b) statistical analysis. SH-SY-5Y cells were pretreated AMPK inhibitor compound C with or without 10 μm-HT for 24 h, protein expression of p-AMPK, AMPK, complex IV, haeme oxygenase 1 (HO-1) and superoxide dismutase 2 (SOD2) was detected by Western blotting: (c) Western blotting image, (d) statistical analysis. , Control;
, HT;
, compound C;
, HT+compound C. Values are means, with standard errors represented by vertical bars (n 3). Mean value was significantly different from that for control: * P< 0·05, ** P< 0·01. Mean value was significantly different from that at time zero: † P< 0·05, †† P< 0·01. Mean value was significantly different from that for the HT-only treatment: ‡ P< 0·05, ‡‡ P< 0·01.
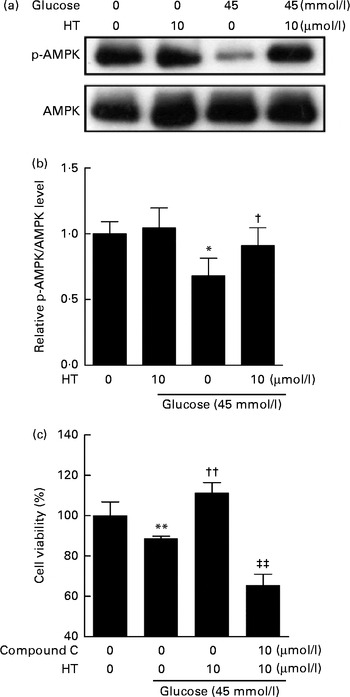
Fig. 8 Inhibition of the AMP-activated protein kinase (AMPK) pathway abolished the protective effects of hydroxytyrosol (HT). SH-SY-5Y cells were pretreated with 10 μm-HT followed by 6 h high glucose treatment. The activation of AMPK pathway was measured by Western blot: (a) Western blot image; (b) statistical analysis. Cells were treated with 10 μm-HT with or without compound C for 24 h, followed by a 6 h treatment with 45 mm-glucose, and cell viability was measured by the 3-(4,5-dimethylthiazol-2-yl)-2,5-diphenyltetrazolium bromide method (c). Values are means, with standard errors represented by vertical bars (n 3). Mean value was significantly different from that for the no-glucose, no-HT treatment: * P< 0·05, ** P< 0·01. Mean value was significantly different from that for the 45 mm-glucose, no-HT treatment: † P< 0·05, †† P< 0·01. ‡‡ Mean value was significantly different from that for the 45 mm-glucose, 10 μm-HT, no compound C treatment (P< 0·01). p-AMPK, phospho-AMPK.
Discussion
The Mediterranean diet is rich in olive oil and has been associated with a slower decline in cognition( Reference Lourida, Soni and Thompson-Coon 36 , Reference Valls-Pedret, Lamuela-Raventos and Medina-Remon 37 ). Limited studies have suggested that polyphenols with anti-oxidative properties may account for the benefits of the Mediterranean diet; however, the primary active components and mechanisms are still unclear. HT is a major polyphenol found in virgin olive oil and has been observed to have anti-inflammatory, anti-cancer and anti-obesity effects. In the present study, we are the first to link the beneficial effects of HT to the diabetic brain with its ability to improve mitochondrial function and activation of phase II antioxidant enzymes by activation of AMPK signalling.
The db/db mice have been widely used as a model of T2DM and other metabolic conditions, such as obesity and dyslipidaemia. A previous study has also observed impaired long-term potentiation and spatial memory in db/db mice( Reference Li, Aou and Oomura 38 ). Furthermore, a recent study has reported central nervous system and peripheral molecular changes in diabetic db/db mice with significant reduction in mitochondria-related molecules including complexes subunit, TCA cycle and the antioxidant SOD2, and concluded that these changes might contribute to the cognitive dysfunction in db/db mice( Reference Ernst, Sharma and Elased 39 ). In the present study, HT treatment could significantly improve the expression levels of mitochondrial complexes I/II/IV significantly in the brain and increase the activity of complex I, the major complex in the electron transport chain and oxidative phosphorylation in the db/db mice. In addition, HT treatment increased antioxidative enzymes, such as HO-1, SOD1 and SOD2, and decreased the protein carbonyl content in the brain of db/db mice. Collected data suggested that HT could improve mitochondrial function and reduce oxidative stress in the brain of db/db mice. Therefore, the mitochondrial protective effects and reduction in oxidative stress might be contributing to the increases in neuronal survival.
Previous study has shown 33 % neuron loss in the brain of db/db mice at 8-month age compared with the control( Reference Shi, Zhang and Zeberg 30 ), which suggested that significant neuron loss may actually happen in the brain of db/db mice. In the present study, we measured the expression of neuron markers including Arc, NMDAR1 and NGF. Arc has been associated with synaptic potentiation and the long-term consolidation of memory( Reference Guzowski, Lyford and Stevenson 40 ). Arc levels are decreased in high-fat diet-fed animals and Alzheimer's diseased brains( Reference Mateos, Akterin and Gil-Bea 41 ). NMDAR, a neurotransmitter receptor, is a key molecule to mediate synaptic plasticity, and the activation of NMDAR is required for Arc function( Reference Steward and Worley 42 ). NGF plays a key role in the development and maintenance of sympathetic and sensory neurons as well as regulation of axonal growth and guidance. Previous studies have reported that NGF could reverse diabetes-related biochemical alterations( Reference Unger, Klitzsch and Pera 43 , Reference Muangman, Muffley and Anthony 44 ). In the present study, all the three factors were significantly higher after HT treatment compared with db/db control mice, suggesting that HT has neuroprotective effects and may potentially improve neuron survival. Whether this protection is attributed to decreased oxidative stress and the improvement of mitochondrial function or other independent mechanisms warrants further investigation.
AMPK is a key player that regulates energy metabolism and is crucial during the development and treatment of obesity, diabetes and other metabolic disorders( Reference Amato and Man 45 ). In addition, brain development and function are closely connected to AMPK signalling, which is also involved in neurodegenerative disorders( Reference Amato and Man 45 , Reference Ronnett, Ramamurthy and Kleman 46 ). Impaired AMPK signalling is linked to mitochondrial dysfunction in brain cells and peripheral neuropathy in diabetes( Reference Chowdhury, Smith and Saleh 47 ). Resveratrol is known to enhance neurite outgrowth and reduce kidney oxidative stress in diabetic rats via activating AMPK signalling( Reference Kitada, Kume and Imaizumi 48 ). It has also been reported that HT could activate AMPK to reduce intracellular ROS levels in vascular endothelial cells( Reference Zrelli, Matsuoka and Kitazaki 49 ). In this study, p-AMPK and two other regulators of AMPK signalling, Sirt1 and PGC-1, were all significantly increased in the brain of HT-treated db/db mice. To further confirm that the AMPK pathway drives the effects of HT, we chose a high glucose-induced toxicity model in SH-HY-5Y neuroblastoma cells. Similar to previous observations in primary neurons, high glucose significantly induced oxidative stress, mitochondrial dysfunction and decreased cell viability( Reference Russell, Golovoy and Vincent 35 ). HT pretreatment efficiently protected mitochondrial function and cell viability, and the addition of compound C, an AMPK inhibitor, abolished these HT effects. Meanwhile, HT induced overexpression of complex IV, one of the mitochondrial electron transport chain and HO-1, one of the antioxidative enzymes was also diminished by compound C. Thus, we suggest that AMPK activation may play major roles in HT-induced mitochondrial biogenesis and phase II enzyme activation, which then protects against high glucose toxicity.
Animal studies have shown that HT can be dose dependently absorbed and excreted in urine( Reference Visioli, Caruso and Plasmati 50 ), and HT concentrations in rat plasma can reach 1·22 μg/ml in 5 min and 1·91 μg/ml in 10 min after 20 mg/kg HT oral administration( Reference Ruiz-Gutierrez, Juan and Cert 51 ), suggesting that the doses of HT used in the present study should be efficiently absorbed and accumulated in vivo. However, the HT content of olive oil varies from 1·55 to 14·42 mg/kg( Reference Tuck and Hayball 52 ), thus humans with an intake of 50 g olive oil/d would receive 0·7 mg of HT at most. The dose of 10 mg/kg used in the present study could only be achieved in humans through the use of very high dose supplements, typically supplementation studies only achieve a total intake of approximately 50 mg/d( Reference Vazquez-Velasco, Esperanza Diaz and Lucas 53 ). The present study showed that very high doses of HT improved mitochondrial function and induced phase II antioxidative enzymes, which decreased oxidative stress in brain of db/db mice. Furthermore, the activation of AMPK signalling by HT may contribute to its protective effects. However, caution should be exercised in extrapolating these findings to lower doses of HT more typically found in humans. There remains the potential for synergistic effects of HT with other polyphenols in olive oil, which could lead to benefits in a realistic dose range suggesting that HT might be an effective agent for the prevention and treatment of diabetic complications such as brain damage.
Supplementary material
To view supplementary material for this article, please visit http://dx.doi.org/10.1017/S0007114515000884
Acknowledgements
This work was supported by the National Basic Research Program (2015CB553602, 2014CB548200), the Fundamental Research Funds for the Central Universities (no. 08143008), the National Natural Science Foundation of China (81201023, 31370844), and the National ‘Twelfth Five-Year’ Plan for Science & Technology Support (2012BAH30F03). The funders had no role in the design, analysis or writing of this article.
The authors' contributions are as follows: A. Z., J. Liu and Z. F. designed the study; A. Z., Ha. L., J. X., K. C., Hu. L., W. P. and Z. Y. conducted the research; A. Z., Y. P. and J. Long analysed the data; J. Liu and Z. F. wrote the manuscript and Z. F. had primary responsibility for the final content. All authors have read and approved the final manuscript.
The authors declare that they have no conflicts of interest.