Introduction
I started my career in 1979 at the CNRS (Centre National de la Recherche Scientifique – National Center for the Scientific Research) in Meudon close to Paris, in the Seed Biology Laboratory supervised by Prof Daniel Côme. Then from 1990 to 1994, I became a Senior lecturer at the University Pierre et Marie Curie (UPMC in Paris) in the team of D. Côme, and from 1994 to 2020, I was a Professor of Plant Physiology in the same University. From 1997 to 2008, I was a Director of the Seed Physiology Laboratory (EA 2388, UPMC) and from 2008 to 2009 I was a Head of the research unit Seed Germination and Dormancy (UR5, UPMC). With the help of Daniel Côme, collaborations and my students, I can say that during my career, I succeeded to be a seed biologist and had the honour to be President-Elect (from 2011 to 2014) and President from 2014 to 2017 of the International Society for Seed Science (ISSS). With Prof D. Côme, we organized the fourth ISSS triennial meeting in Angers in 1992. From 2010 to 2017, I reduced my research activities since I accepted the position of Vice-President delegate to estate of my University, and came back in my laboratory in 2017 to supervising my last PhD students. From 1983, the year of obtaining my PhD degree (Corbineau, Reference Corbineau1983), my scientific work concerned the following main topics:
(1) Physiological, biochemical and hormonal regulation of seed germination and dormancy as related to external factors, in particular temperature and oxygen; (2) Regulation of ethylene biosynthesis and involvement of this plant hormone in germination and breaking of dormancy; (3) Physiological and biochemical mechanisms involved in the response of seeds and seedlings to abiotic stresses (dehydration, chilling, high temperatures); (4) Ageing and storage of orthodox and recalcitrant seeds and (5) Evaluation and improvement of seed quality.
As oxygen was always a key factor of the scientific work that I have done in relation to seed photosensitivity (Corbineau, Reference Corbineau1983; Corbineau and Côme, Reference Corbineau and Côme1985), metabolism, energy charge (EC) and the pentose-phosphate pathway, expression and breaking of seed dormancy (Corbineau and Côme, Reference Corbineau, Côme, Kigel and Galili1995), I have chosen to talk about oxygen as a key signalling factor in the control of seed germination and dormancy for this specific J. Derek Bewley, Career Lecture.
Seed germination is subjected to a very precise regulation, the complexity of which originates both in the environmental factors and the seeds themselves. Water, oxygen and temperature are the three essential factors regulating seed germination and dormancy expression (Bewley and Black, Reference Bewley and Black1994; Corbineau and Côme, Reference Corbineau, Côme, Kigel and Galili1995; Baskin and Baskin, Reference Baskin and Baskin1998; Finch-Savage and Leubner-Metzger, Reference Finch-Savage and Leubner-Metzger2006; Finch-Savage and Footitt, Reference Finch-Savage and Footitt2017). Water uptake is required in order to allow resumption of seed metabolism among which respiration, protein and RNA synthesis, plant hormone (in particular abscisic acid, gibberellins and ethylene) biosynthesis and signalling pathways (Leopold and Vertucci, Reference Leopold, Vertucci, Stanwood and McDonald1989; Bewley and Black, Reference Bewley and Black1994; Bradford, Reference Bradford, Kigel and Galili1995; Hadas, Reference Hadas, Benech-Arnold and Sanchez2004; Benech-Arnold et al., Reference Benech-Arnold, Gualano, Leymarie, Côme and Corbineau2006; Bradford et al., Reference Bradford, Benech-Arnold, Côme and Corbineau2008; Holdsworth et al., Reference Holdsworth, Bentsink and Soppe2008; Nonogaki, Reference Nonogaki2010, Reference Nonogaki2014, Reference Nonogaki2017, Reference Nonogaki2019; Nonogaki et al., Reference Nonogaki, Bassel and Bewley2010).
Oxygen is essential for respiration but its requirement for seed germination depends on the species, depth of dormancy and temperature (Al-Ani et al., Reference Al-Ani, Bruzau, Raymond, Saint-Ges, Leblanc and Pradet1985; Côme et al., Reference Côme, Corbineau and Lecat1988; Corbineau and Côme, Reference Corbineau, Côme, Kigel and Galili1995; Bradford et al., Reference Bradford, Côme and Corbineau2007, Reference Bradford, Benech-Arnold, Côme and Corbineau2008). In anoxia/hypoxia, the production of ATP by fermentation allows the maintenance of EC in the seeds (Raymond et al., Reference Raymond, Al-Ani and Pradet1985; Côme and Corbineau, Reference Côme, Corbineau and Taylorson1989). However, partial or total oxygen deprivation may be beneficial to germination of various aquatic species or some dormant seeds and complete deprivation of oxygen can break dormancy of some seeds (Tissaoui and Côme, Reference Tissaoui and Côme1973; Côme et al., Reference Côme, Corbineau, Soudain, Jackson, Davies and Lambers1991; Corbineau and Côme, Reference Corbineau, Côme, Kigel and Galili1995; Baskin and Baskin, Reference Baskin and Baskin1998).
In the present paper, major data obtained from 60 to 85s that mainly take the form of descriptive physiology (1) investigating the sensitivity of seeds to oxygen supply and the role played by surrounding structures in the diffusion of oxygen from the medium to the embryo (Côme, Reference Côme1967, Reference Côme1970; Côme and Tissaoui, Reference Côme, Tissaoui and Heydecker1973; Côme and Corbineau, Reference Côme, Corbineau, Jiarui and Khan1992, Reference Côme, Corbineau and Mazliak1998; Corbineau and Côme, Reference Corbineau, Côme, Kigel and Galili1995) and (2) measuring the respiration activity and energy metabolism during germination of non-dormant and dormant seeds incubated in low oxygen supply (Côme et al., Reference Côme, Corbineau and Lecat1988; Côme and Corbineau, Reference Côme, Corbineau and Taylorson1989; Corbineau and Côme, Reference Corbineau, Côme, Nicolas, Bradford, Côme and Pritchard2003) are highlighted.
I also highlight recent developments obtained over the last 30 years concerning the hormonal metabolism and signalling in seed responsiveness to low oxygen (Shu et al., Reference Shu, Zhang, Wang, Chen and Wu2013; Nonogaki, Reference Nonogaki2017, Reference Nonogaki2019), the role of the N-end rule pathway as the oxygen signalling pathway (Gibbs et al., Reference Gibbs, Lee, Isa, Gramuglia, Fukao, Bassel, Correia, Corbineau, Theodoulou, Bailey-Serres and Holdsworth2011, Reference Gibbs, Bacardit, Bachmair and Holdsworth2014a,Reference Gibbs, Isa, Movahedi, Lozano-Juste, Mendiondo and Berckhanb, Reference Gibbs, Conde, Berckhan, Prasad, Mendiondo and Holdsworth2015, Licausi, Reference Licausi2011, Reference Licausi2013; Licausi et al., Reference Licausi, Kosmacz, Weits, Giuntoli, Giorgi, Voesenek, Perata and Van Dongen2011, Reference Licausi, Pucciariello and Perata2013; Bailey-Serres et al., Reference Bailey-Serres, Fukao, Gibbs, Holdsworth, Lee, Licausi, Perata, Voesenek and Van Dongen2012) and the emerging role for ROS and NO and interorganelle communication in control of seed germination (Benamar et al., Reference Benamar, Rolletschek, Borisjuk, Avelange-Macherel, Curien, Mostefai, Andriantsitohaina and Macherel2008; Wang and Auwerx, Reference Wang and Auwerx2017; Meng et al., Reference Meng, Li, Narsai, De Clercq, Whelan and Berkowitz2020; Bailly and Merendino, Reference Bailly and Merendino2021; Jurdak et al., Reference Jurdak, Launay-Avon, Paysant-le Roux and Bailly2021).
Diffusion of oxygen from the atmosphere, in the soil and in the seed
The soil atmosphere composition
Partial pressure of oxygen depends on soil structure and compaction (Hadas, Reference Hadas, Benech-Arnold and Sanchez2004). In agricultural soils, oxygen content has been found to vary from 19 to 21%, thus indicating that it is not limiting if the soil is well drained (Richard and Boiffin, Reference Richard and Boiffin1990). However, the oxygen level can decrease to 10% when a crust is present at the soil surface leading to reduced exchange between the atmosphere and the soil (Richard and Guerif, Reference Richard and Guerif1988a,Reference Richard and Guerifb; Dekker, Reference Dekker, Black, Bradford and Vazquez-Ramos2000; Hadas, Reference Hadas, Benech-Arnold and Sanchez2004). In flooded soil when the pore system is filled with water (Gambrell et al., Reference Gambrell, Delaune, Patrick, Jackson, Davies and Lambers1991), the oxygen level can be less than 1% since there is a 104 reduction in oxygen diffusion in water relative to air, and oxygen solubility in water decreases with increasing temperature being 14.56 mg l−1 at 0°C, 11.25 mg l−1 at 10°C, 9.09 mg l−1 at 20°C and 7.49 mg l−1 at 30°C.
The level of CO2 does not usually exceed 0.5–1% in drained soil, but can increase up to 5–8% in flooded soils and silt loams when decomposition of organic matter occurs (Buyanovsky and Wagner, Reference Buyanovsky and Wagner1983; Nakayama and Kimball, Reference Nakayama and Kimball1988).
Seed coat structures: barriers to oxygen diffusion to the embryo
Embryo covering structures include nucellus and testa and endosperm in some species. The pericarp is fused to the seed testa in the case of caryopses, and it is not fused to the testa in the case of achenes. They are associated with various other structures among which hulls consisting of glumellae or glumes depending on the Poaceae species, and dead perianth tissues, for example in beet. They inhibit seed germination by controlling seed water uptake and oxygen supply to the embryo (Bewley and Black, Reference Bewley and Black1994; MacGregor et al., Reference MacGregor, Kendall, Florance, Fedi, Moore, Paszkiewicz, Smirnoff and Penfield2015). Surrounding structures impact oxygen diffusion and are responsible for a reduction of oxygen diffusion to the embryo that depends on their thickness and their biochemical properties. Oxygen goes through the seed coat by dissolving in the water they contain during seed imbibition, but since oxygen solubility is low, little oxygen reaches the embryo. Imbibed coats become then a barrier to oxygen diffusion since they constitute a continuous wet layer surrounding the embryo (Côme, Reference Côme1970, Reference Côme and Mazliak1982; Côme and Tissaoui, Reference Côme, Tissaoui and Heydecker1973; Côme and Corbineau, Reference Côme, Corbineau, Jiarui and Khan1992, Reference Côme, Corbineau and Mazliak1998).
Furthermore, seed coats of numerous seeds often contain phenolic compounds such as phenolic acids (p-hydroxybenzoic-, vanillic-, gallic-, p-coumaric-, caffeic-, ferulic-, sinapic-, chlorogenic acids), coumarins, flavonoids and tannins that are end products of flavonoid biosynthetic pathway (Glennie, Reference Glennie1981; Lenoir et al., Reference Lenoir, Corbineau and Côme1986; Winkel-Shirley, Reference Winkel-Shirley1998, Reference Winkel-Shirley2001; Croteau et al., Reference Croteau, Kutchan, Lewis, Buchanam, Gruissem and Jones2000; Tian et al., Reference Tian, Nakamura and Kayara2004; Lepiniec et al., Reference Lepiniec, Debeaujon, Routaboul, Baudry, Pourcel, Nesi and Caboche2006; Rodriguez et al., Reference Rodriguez, Barrero, Corbineau, Gubler and Benech-Arnold2015). In the presence of oxygen, the oxidation of the phenolic compounds is mediated by various enzymes: monophenol oxidase which converts monophenols into o-diphenols, catechol oxidase which oxidizes the o-diphenols into o-quinones and laccases (Macheix et al., Reference Macheix, Fleuriet and Billot1990, Reference Macheix, Fleuriet and Jay-Allemand2005; Debeaujon et al., Reference Debeaujon, Lepiniec, Pourcel, Routaboul, Bradford and Nonogaki2007). In both cases, quinones are then polymerized into brown pigment by a non-enzymatic mechanism. For example, flavan-4-ols, precursors of phlobaphenes, confer a red colour to wheat, maize and rice grains (Grotewold et al., Reference Grotewold, Drummond, Bowen and Peterson1994; Himi et al., Reference Himi, Mares, Yanagisawa and Noda2002, Reference Himi, Yamashita, Haruyama, Yanagisawa, Maekawa and Taketa2012), but condensation of flavan-3-ols leads to proanthocyanidin synthesis the oxidation of which confers a brown colour (Winkel-Shirley, Reference Winkel-Shirley1998).
Many studies have demonstrated that phenolic compounds, in particular flavonoids, secondary metabolites derived from the phenylpropanoid pathway (Lepiniec et al., Reference Lepiniec, Debeaujon, Routaboul, Baudry, Pourcel, Nesi and Caboche2006; Debeaujon et al., Reference Debeaujon, Lepiniec, Pourcel, Routaboul, Bradford and Nonogaki2007) reinforce coat-imposed dormancy. For example, Arabidopsis seeds accumulate flavonols, and proanthocyanidins during development, the brown colour being conferred by proanthocyanidin after oxidation. This pathway has been characterized using transparent testa tt(g) mutants that are affected in seed coat pigmentation (Debeaujon et al., Reference Debeaujon, Lepiniec, Pourcel, Routaboul, Bradford and Nonogaki2007; North et al., Reference North, Baud, Debeaujon, Dubos, Dubreucq, Grappin, Jullien, Lepiniec, Mario-Poll, Miquel, Rajjou, Routaboul and Caboche2010); mutants lacking PAs germinate faster than the corresponding wild-type brown seeds. Flavonoids, particularly condensed tannins, are known to regulate water uptake (Debeaujon et al., Reference Debeaujon, Léon-Kloosterziel and Koornneef2000; MacGregor et al., Reference MacGregor, Kendall, Florance, Fedi, Moore, Paszkiewicz, Smirnoff and Penfield2015), however, their impact on oxygen diffusion is unknown. In wheat and rice, red grains show a more intense dormancy than the white grain lines and thus have a higher resistance to pre-harvest sprouting (PHS) (Cohn and Hughes, Reference Cohn and Hughes1981; Flintham, Reference Flintham2000), this trait has provided breeders with an easy character to select cultivars with higher dormancy even if ‘red gene’ contribution to dormancy is small (Warner et al., Reference Warner, Kudrna, Spaeth and Jones2000; Himi et al., Reference Himi, Yamashita, Haruyama, Yanagisawa, Maekawa and Taketa2012).
In cereals, the covering structures result in the inability of the grains to germinate at temperature higher than 10–15°C. In barley, the glumellae have the main role in dormancy (Lenoir et al., Reference Lenoir, Corbineau and Côme1983, Reference Lenoir, Corbineau and Côme1986; Corbineau and Côme, Reference Corbineau and Côme1996), while in oat both glumellae with pericarp, and probably testa participate to dormancy (Corbineau et al., Reference Corbineau, Lecat and Côme1986). In naked grains such as sorghum and wheat, dormancy results from the pericarp, testa and endosperm (Steinbach et al., Reference Steinbach, Benech-Arnold, Kristof, Sanchez and Marcucci Poltri1995). The surrounding structures inhibit germination by reducing oxygen supply to the embryo due to enzymatic and/or non-enzymatic oxidation of the phenolic compounds (Côme, Reference Côme1967, Reference Côme1980/81; Lenoir et al., Reference Lenoir, Corbineau and Côme1986; Fig. 1). The inhibitory effect of the covering structures increases with increasing temperature since the fixation of oxygen in the surrounding structures increases with temperatures (Lenoir et al., Reference Lenoir, Corbineau and Côme1986; Lecat et al., Reference Lecat, Corbineau and Côme1992). In barley, for example, more than 50% of the total oxygen uptake by the grain results from oxidation of phenolic compounds in the glumellae, whereas it is less than 20% at 10–15°C (Côme et al., Reference Côme, Corbineau and Lecat1988; Fig. 1). More recently using fibre-optic O2 micro-sensor, Hoang et al. (Reference Hoang, Bailly, Corbineau and Leymarie2013) demonstrated that the oxygen content in the embryo below the glumellae is around 15.8% at 15°C, temperature that allows germination, but only 0.3% at 30°C, temperature at which grains remain dormant.
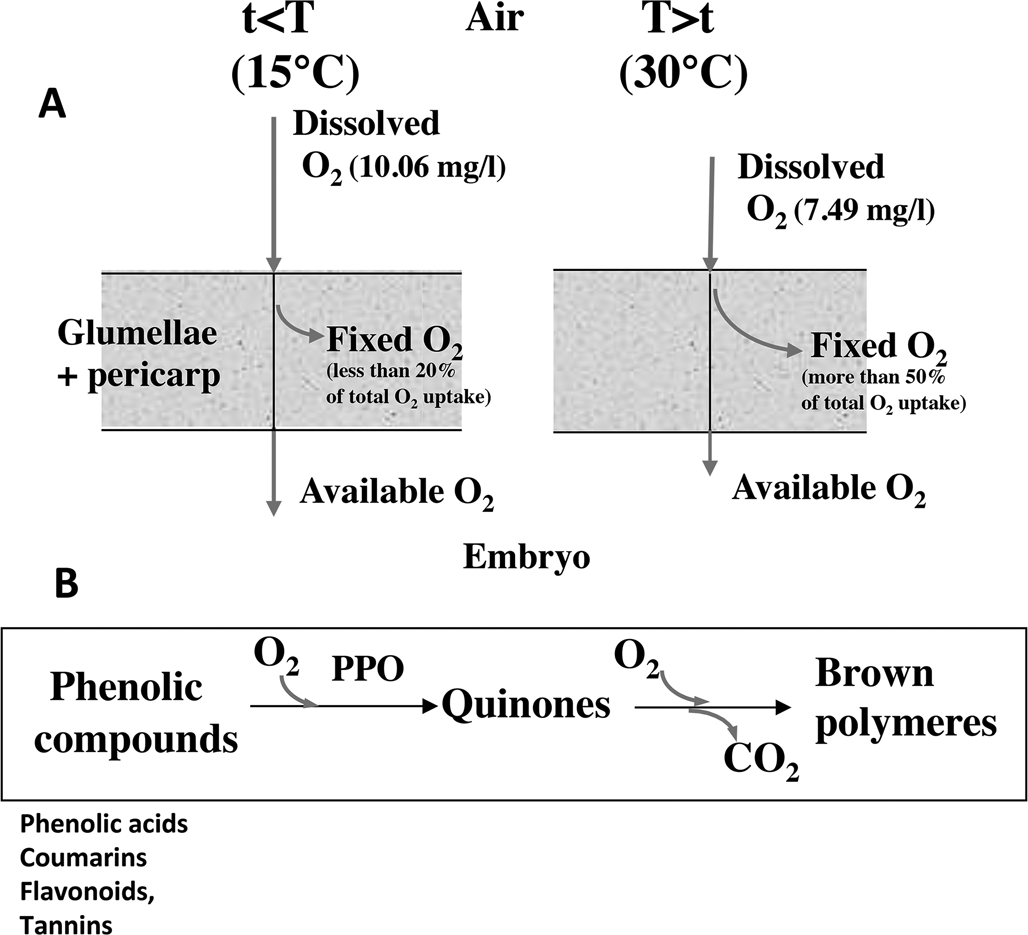
Fig. 1. Effects of the surrounding structures on oxygen supply to the embryo. (A) Effects of temperature on the oxygen flux through the coats (pericarp + glumellae) in dormant barley grains incubated at 15°C (left) and 30°C (right). Increase in temperature results in a decrease in oxygen solubility in water during seed imbibition and in an increase in oxygen uptake through oxidation of phenolic compounds. As a result, the embryo receives less oxygen at 30°C than at 10–15°C. Adapted from Côme and Tissaoui (Reference Côme, Tissaoui and Heydecker1973), Lenoir et al. (Reference Lenoir, Corbineau and Côme1983, Reference Lenoir, Corbineau and Côme1986), Côme et al. (Reference Côme, Corbineau and Lecat1988), Côme and Corbineau (Reference Côme, Corbineau, Jiarui and Khan1992) and Corbineau and Côme (Reference Corbineau, Côme, Kigel and Galili1995). (B) Oxidation of phenolic compounds in the seed coats. The phenolic compounds are first oxidized to quinones by polyphenol oxidases (PPO) and then the quinones undergo non-enzymatic oxidative polymerization. From Côme and Corbineau (Reference Côme, Corbineau, Jiarui and Khan1992) and Corbineau and Côme (Reference Corbineau, Côme, Kigel and Galili1995).
However, afterripening that breaks dormancy has no effect on the phenolic compound composition nor on the polyphenol oxidase activity, but delays in time the oxygen uptake by the glumellae allowing a good oxygen supply to the embryo during the first hours of imbibition (Lenoir et al., Reference Lenoir, Corbineau and Côme1983, Reference Lenoir, Corbineau and Côme1986; Corbineau et al., Reference Corbineau, Lecat and Côme1986).
Oxygen requirement for germination of non-dormant seeds
Oxygen requirements for germination have been reviewed by different authors (Côme, Reference Côme and Mazliak1982; Mayer and Poljakoff-Mayber, Reference Mayer and Poljakoff-Mayber1989; Côme and Corbineau, Reference Côme, Corbineau, Jiarui and Khan1992; Bewley and Black, Reference Bewley and Black1994; Corbineau and Côme, Reference Corbineau, Côme, Kigel and Galili1995; Bradford et al., Reference Bradford, Côme and Corbineau2007, Reference Bradford, Benech-Arnold, Côme and Corbineau2008). However, it is not always indicated whether seeds under investigation are dormant or not, although seed sensitivity to oxygen depends on the depth of dormancy (Corbineau and Côme, Reference Corbineau, Côme, Kigel and Galili1995; Bradford et al., Reference Bradford, Côme and Corbineau2007, Reference Bradford, Benech-Arnold, Côme and Corbineau2008) and other environmental factors as temperature, light or water potential of the medium.
For most species, seeds fail to germinate when deprived of oxygen, and restricted oxygen availability prevents seedling growth. However, several aquatic species like Alisma plantago (water plantain), Cynodon dactylon (bermuda grass), Echinochloa turnerana (barnyard grass), Leersia oryzoides (rice cut-grass), Thypha latifolia (cattail), Trapa natans (water caltrop) and Zizania aquatica (wild rice) germinate better under reduced oxygen concentrations than in air (review by Corbineau and Côme (Reference Corbineau, Côme, Kigel and Galili1995)). Al-Ani et al. (Reference Al-Ani, Leblanc, Raymond and Pradet1982, Reference Al-Ani, Bruzau, Raymond, Saint-Ges, Leblanc and Pradet1985) classified seeds in two groups according to their response to low oxygen concentration. Group I corresponds to fatty seeds (cabbage, flax, lettuce, radish, soybean, sunflower, turnip) the germination of which is completely inhibited when oxygen concentration is close to 2% (Fig. 2). Seeds of group II are mainly starchy seeds among which cereals (barley, maize, oat, rice, sorghum, wheat) and pea, and they did not germinate in atmosphere containing less than 1% oxygen (Fig. 2). Figure 2 indicates also that 50% of the seed population germinate in 1–3 and 7–8% oxygen for groups II and I, respectively.
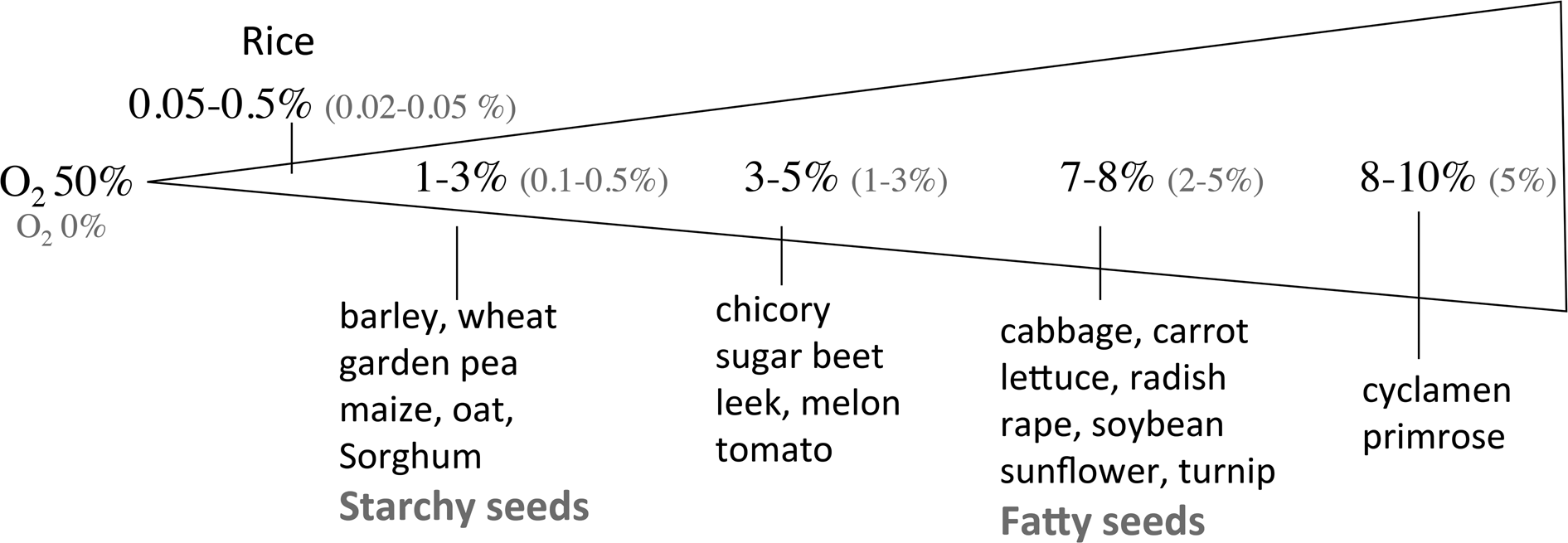
Fig. 2. Oxygen concentration of the atmosphere below which no seed germinated (O2 0% in red) or which allowed 50% germination (O2 50% in black). From Corbineau (Reference Corbineau1995) and Corbineau and Côme (Reference Corbineau, Côme, Kigel and Galili1995).
Oxygen requirement for germination depends on environmental factors including temperature, osmotic pressure of the medium and light (review by Corbineau and Côme (Reference Corbineau, Côme, Kigel and Galili1995)). For many species, the sensitivity to oxygen deprivation decreases with decreasing temperature, probably because oxygen solubility in water increases with decreasing temperature. In addition, sunflower (Smok et al., Reference Smok, Chojnowski, Corbineau, Côme, Côme and Corbineau1993) and tomato seeds (Corbineau and Côme, Reference Corbineau, Côme, Kigel and Galili1995) require more oxygen for their germination when placed on a medium with low water potential. For example, in the case of tomato, 50% of the population germinate within 7 d on water at 25°C in around 5% oxygen, but germination requires at least 10% oxygen when seeds were incubated on a PEG-6000 solution at 0.5 MPa. Continuous white light also reinforces the seed responsiveness to hypoxia in case of negative photosensitive seeds of Amaranthus caudatus (Gutterman et al., Reference Gutterman, Corbineau and Côme1992) and Bromus rubens (Corbineau et al., Reference Corbineau, Belaid and Côme1992).
Table 1 indicates that 2–3% oxygen in the atmosphere is sufficient for the germination of isolated embryos. Isolated embryos from non-dormant seeds require less oxygen for germinating than the corresponding intact seeds indicating that even in non-dormant seeds, the seed coats inhibit the germination by reducing the oxygen supply to the embryos (Table 1; Corbineau and Côme, Reference Corbineau, Côme, Kigel and Galili1995; Côme and Corbineau, Reference Côme, Corbineau and Mazliak1998).
Table 1. Effects of oxygen tensions on the germination of non-dormant seeds of melon, oat and sunflower placed for 7 d at 20°C and of the corresponding isolated embryos
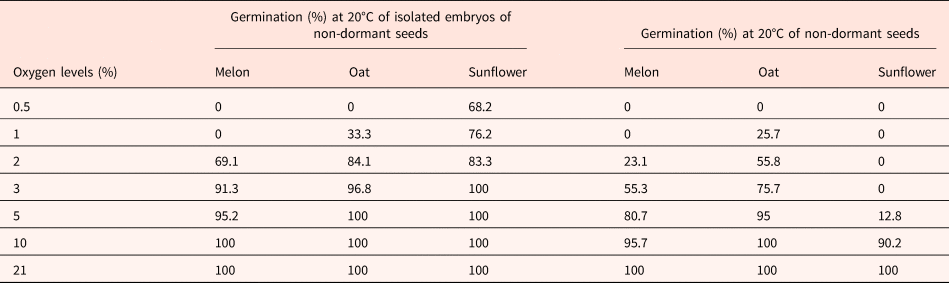
Means of 4 replicates.
Modified from Corbineau and Côme (Reference Corbineau, Côme, Kigel and Galili1995) and Côme and Corbineau (Reference Côme, Corbineau and Mazliak1998).
Consequence of dormancy on oxygen requirement for germination
Relationships between seed dormancy and responsiveness to oxygen
For some species, dormant seeds do not germinate in air (21% oxygen), but their germination capacities are improved in hypoxia. Table 2 shows the sensitivity to oxygen of Oldenlandia corymbosa L. seeds as a function of oxygen in dormant seeds (at harvest) and non-dormant ones (after breaking of dormancy by a chilling treatment) (Corbineau and Côme, Reference Corbineau and Côme1980a). In dormant seeds, 91–95% of the population easily germinate in a narrow range of oxygen (5–7%) when the non-dormant seeds germinate in air and in a wide range of oxygen concentration from 5 to 21%. Similar behaviour was observed in dormant apple embryos (Côme et al., Reference Côme, Perino and Ralambosoa1985); at harvest, dormant apple embryos do not germinate in air at 15°C, but their germination is improved at lower oxygen concentration. Release of dormancy enables them to germinate in a large range of oxygenation (Côme et al., Reference Côme, Perino and Ralambosoa1985).
Table 2. Effects of oxygen tensions on the germination of dormant and non-dormant seeds of Oldenlandia corymbosa incubated for 7 d at 40°C in continuous light

Means of 3 replicates.
Modified from Corbineau and Côme (Reference Corbineau and Côme1980a, Reference Corbineau and Côme1980/81).
However, in the majority of species, seeds are more sensitive to oxygen deprivation when they are dormant than the non-dormant ones. This influence of dormancy on oxygen sensitivity has been demonstrated with cereals like barley (Corbineau and Côme, Reference Corbineau and Côme1980b; Benech-Arnold et al., Reference Benech-Arnold, Gualano, Leymarie, Côme and Corbineau2006), wheat (Corbineau et al., Reference Corbineau, Sanchez, Côme and Chaussat1981) and oat (Lecat et al., Reference Lecat, Corbineau and Côme1992), sunflower (Gay et al., Reference Gay, Corbineau and Côme1991; Corbineau and Côme, Reference Corbineau, Côme, Jiarui and Khan1992, Reference Corbineau, Côme, Kigel and Galili1995) and Douglas fir (Pseudotsuga menziesii) (Corbineau et al., Reference Corbineau, Bianco, Garello and Côme2002). Figure 3 shows, in barley, the changes in oxygen sensitivity of the intact grains (Fig. 3A) and isolated embryos (Fig. 3B) incubated on water at 20°C at harvest (i.e. dormant grains) and during afterripening (grains dry-stored for 5 and 9.5 months at 25°C). At harvest in 2000, less than 50% of the grain population can germinate at 20°C in air (21% oxygen) and no germination occurred in atmosphere containing less than 3% oxygen (Fig. 3A). Dry storage of dormant grains for 5 and 9.5 months at 25°C results in breaking of dormancy allowing germination of all the grain population in air and reducing the oxygen concentration at which 50% of the population germinate to 4.8% and 0.4% (Fig. 3A). By contrast, isolated embryos from dormant grains at harvest germinate in atmosphere containing at least 5% oxygen (Fig. 3B, curve 1) demonstrating that the covering structures are involved in oxygen diffusion (c.f. Oxygen requirement for germination of non-dormant seeds). Afterripening also resulted in an improvement of embryo germination in hypoxia: 100% of the population germinate in 4 and 0.5% oxygen, respectively, after 5 and 9.5 months of dry storage at 25°C (Fig. 3B, curves 2 and 3).
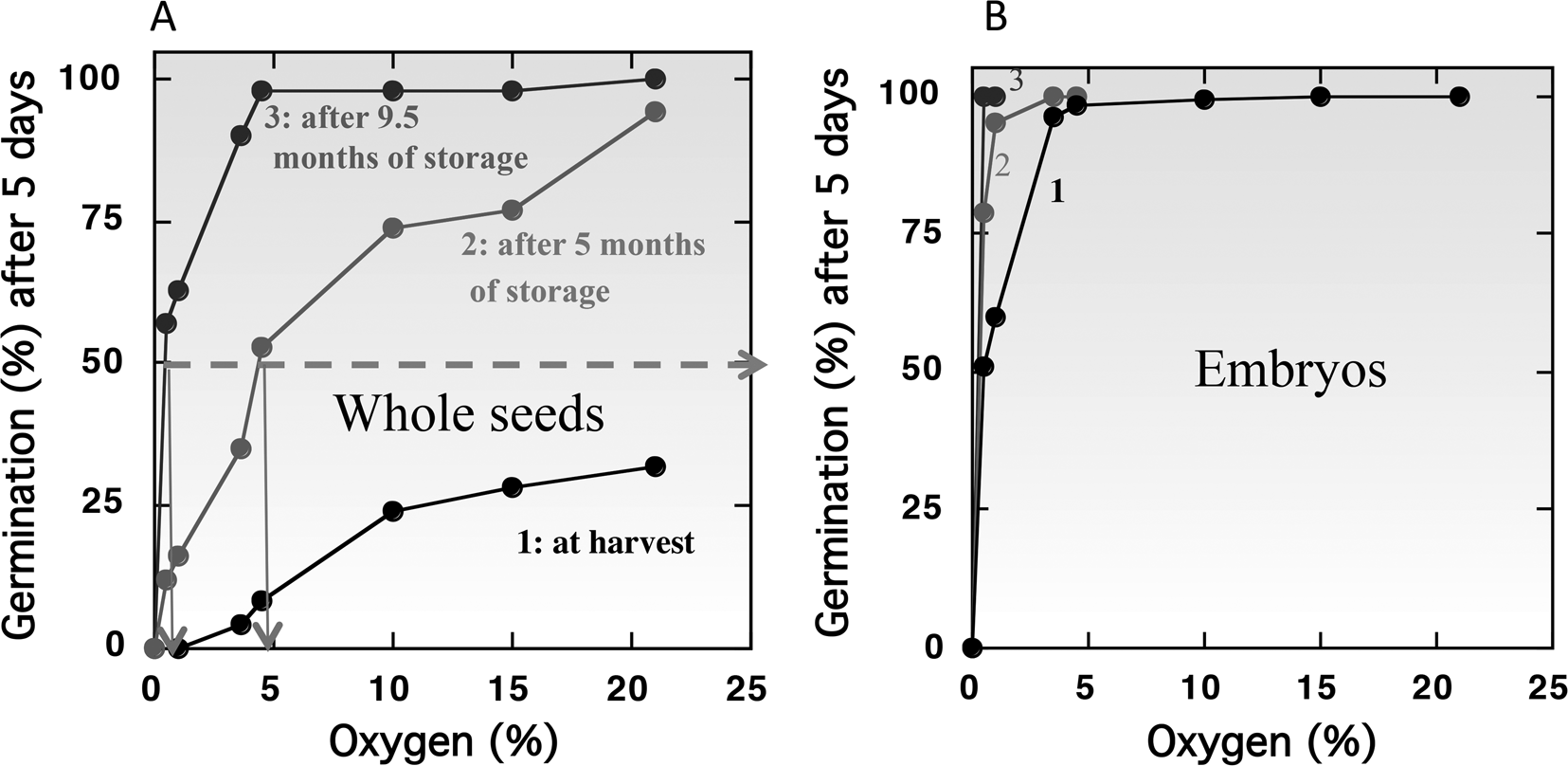
Fig. 3. Effects of oxygen concentration in the atmosphere on the germination percentages obtained after 5 d at 20°C with barley caryopses (A) and embryos (B) at harvest (1) and after 5 months (2) and 9.5 months (3) of afterripening at 25°C. Seeds harvested in 2000 and stored in the open air for 5 and 9.5 months at 25°C. Modified from Bradford et al. (Reference Bradford, Benech-Arnold, Côme and Corbineau2008).
Using a population-based threshold model, Bradford et al. (Reference Bradford, Benech-Arnold, Côme and Corbineau2008) have calculated a median base (or threshold) O2 [(Ox(b)(50)]. The model predicted that 36.3% oxygen would be required for 50% germination of intact dormant grains at harvest in 2000, while the germination of isolated embryos was predicted to require only 0.123% oxygen to achieve 50% germination. For the intact grains, the Ox(b)(50) value was 2.87% and 0.30% after 5 and 9.5 months of afterripening, respectively (Bradford et al., Reference Bradford, Benech-Arnold, Côme and Corbineau2008). Environmental conditions during seed production influence their sensitivity to oxygen. Hence, the Ox(b)(50) is dependent of the year of harvest and was decreased to 15.7% for grains obtained in 2002 compared to 36.3% for those obtained in 2000 (Fig. 3A and Table 3).
Table 3. Effects of ABA or GA3 concentration on oxygen tensions on the median O2 threshold Oxb(50) calculated using a population-based threshold model in dormant seeds and isolated embryos in barley
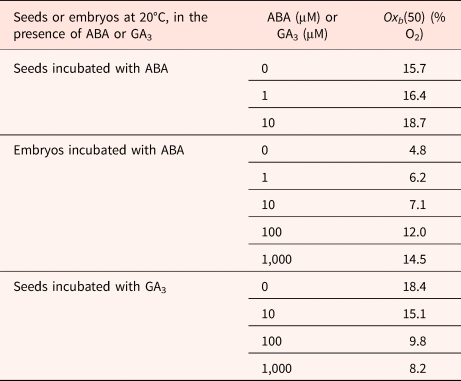
Seeds harvested in 2002.
Modified from Bradford et al. (Reference Bradford, Benech-Arnold, Côme and Corbineau2008).
Specific effect of hypoxia and anoxia on breaking of dormancy and induction of secondary dormancy
Oxygen is required for germination, however partial or total oxygen deprivation is not always harmful at least if it is not too prolonged. Temporary anaerobiosis applied to imbibed seeds may promote germination in dormant seeds of some species (Lonchamp and Gora, Reference Lonchamp and Gora1979; Côme et al., Reference Côme, Perino and Ralambosoa1985) and may substitute for cold stratification (Côme et al., Reference Côme, Corbineau, Soudain, Jackson, Davies and Lambers1991; Corbineau and Côme, Reference Corbineau, Côme, Kigel and Galili1995). For example, incubation in anoxia (pure nitrogen) for 2 or 3 weeks breaks dormancy in apple embryos (Tissaoui and Côme, Reference Tissaoui and Côme1973), Oldenlandia corymbosa seeds (Corbineau and Côme, Reference Corbineau and Côme1988), sunflower achenes (Corbineau and Côme, Reference Corbineau, Côme, Kigel and Galili1995) and Xanthium pennsylvanicum (Esashi et al., Reference Esashi, Kotaki and Ohhara1976). In the case of apple embryos, this beneficial effect of anoxia increases with temperature (Tissaoui and Côme, Reference Tissaoui and Côme1973).
In contrast, incubation of seeds in anoxia or hypoxia, conditions which inhibit seed germination, induces secondary dormancy in barley (Hoang et al., Reference Hoang, Bailly, Corbineau and Leymarie2013), Xanthium pennsylvanicum (Esashi et al., Reference Esashi, Okazaki, Yanai and Hishinuma1978), Viola spp., Veronica hederofolia and Veronica persica (Lonchamp and Gora, Reference Lonchamp and Gora1979), lettuce (Vidaver and Hsiao, Reference Vidaver and Hsiao1975) and Brassica napus (Pekrun et al., Reference Pekrun, Lutman and Baeumer1997). In Oldenlandia corymbosa seeds, responsiveness to light depends also on the temperature and on the oxygen tension of the atmosphere. Seeds remain responsive to white light at 10 and 20°C, but lose their responsiveness during incubation in darkness at temperature of at least 30°C; this phenomenon has been observed in many photoblastic seeds (Karssen, Reference Karssen1967; Rollin, Reference Rollin1970) and is accelerated at low oxygen concentration in Oldenlandia corymbosa (Corbineau and Côme, Reference Corbineau and Côme1985).
Effects of hypoxia on ABA and GA metabolism and sensitivity
The hormonal regulation of dormancy and germination is well established (Bewley, Reference Bewley1997; Finch-Savage and Leubner-Metzger, Reference Finch-Savage and Leubner-Metzger2006; Finkelstein et al., Reference Finkelstein, Reeves, Ariizumi and Steber2008; Holdsworth et al., Reference Holdsworth, Bentsink and Soppe2008; Graeber et al., Reference Graeber, Nakabayashi, Miatton, Leubner-Metzger and Soppe2012; Shu et al., Reference Shu, Liu, Xie and He2016; Nonogaki, Reference Nonogaki2017). Oxygen uptake by the glumellae during the first 12–14 h of imbibition appears to be related to ABA metabolism in the embryo, as a transient increase in ABA content occurs in dormant grains and not in non-dormant ones (Benech-Arnold et al., Reference Benech-Arnold, Gualano, Leymarie, Côme and Corbineau2006). The presence of glumellae alters the expression of genes involved in ABA synthesis (HvNCED1, NCED2), ABA catabolism (HvABA8’OH1) and signalling (HvABI5, HvVP1 and HvPKABA) (Mendiondo et al., Reference Mendiondo, Leymarie, Farrant, Corbineau and Benech-Arnold2010). During imbibition, the decrease in embryonic ABA level is slower in hypoxia than in air, but this effect is independent of the regulation of HvABA8’OH1 expression (Mendiondo et al., Reference Mendiondo, Leymarie, Farrant, Corbineau and Benech-Arnold2010; Hoang et al., Reference Hoang, Bailly, Corbineau and Leymarie2013). Incubation of embryos of primary dormant grains in the presence of exogenous ABA at various concentrations shows that hypoxia increased embryo sensitivity to ABA by twofold (Fig. 4), and this effect was more pronounced at 30°C than at 20°C (Benech-Arnold et al., Reference Benech-Arnold, Gualano, Leymarie, Côme and Corbineau2006), suggesting that hypoxia imposed by glumellae increases embryo sensitivity to ABA.
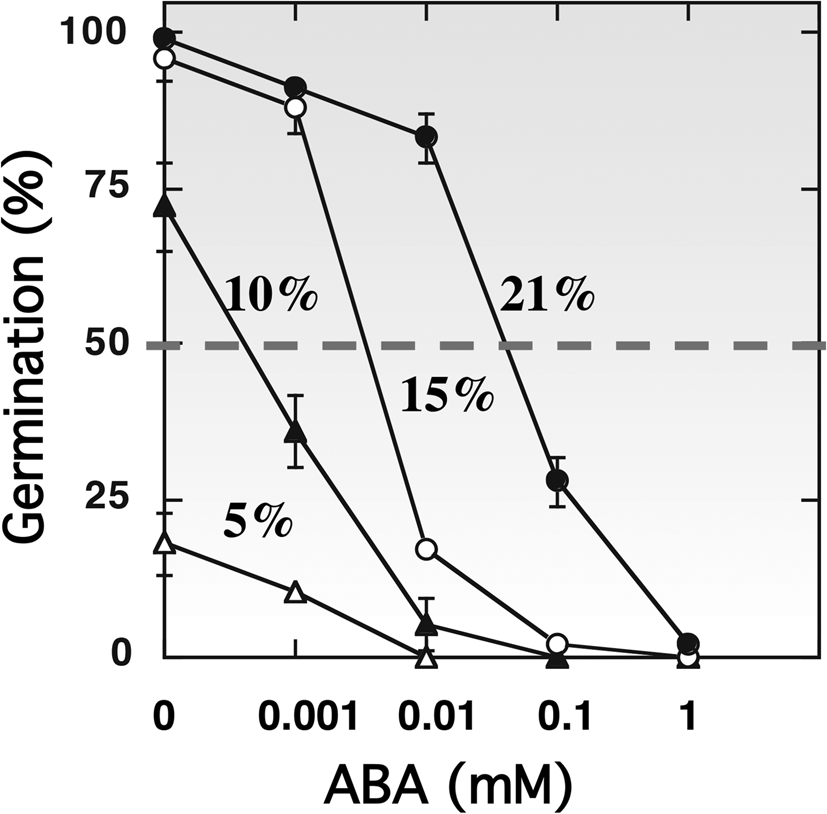
Fig. 4. Effects of ABA concentration on the germination percentages obtained after 7 d with embryos isolated from dormant barley grains placed at 30°C in 21% (filled circles), 15% (open circles), 10% (filled triangles) and 5% (open triangles) oxygen. Seeds harvested in 2000. Means of 2 measurements ± arithmetical spread. From Benech-Arnold et al. (Reference Benech-Arnold, Gualano, Leymarie, Côme and Corbineau2006).
ABA delayed and reduced seed and embryo germination; using a population-based threshold model, Bradford et al. (Reference Bradford, Benech-Arnold, Côme and Corbineau2008) show that ABA concentration up to 10 μM had relatively little effect on O2 sensitivity of seeds. However, at higher concentration, the value of Oxb50 increased from 4.8% in the absence of ABA to 12 and 14.5% in the presence of 100 and 1000 μM ABA, respectively (Table 3). Incubation of embryos in the presence of GA3 results in a decrease in Oxb50 (Table 3) from 18.4% on water down to 8.2% in the presence of 1000 μM GA.
Hypoxia at 15°C which induced secondary dormancy in barley was related to an increased expression of ABA synthesis genes (HvNCED2) only after 3 d and a strong regulation of gibberellin (GA) metabolism genes with promotion in HvGA2ox3 (64-fold compared with grains in air) and inhibition of HvGA3ox2 and HvGA20ox1 after 1 d (Hoang et al., Reference Hoang, Bailly, Corbineau and Leymarie2013). In addition, embryo sensitivity to ABA increases in hypoxia and seeds became insensitive to GA at low oxygen (Benech-Arnold et al., Reference Benech-Arnold, Gualano, Leymarie, Côme and Corbineau2006). However, hypoxia did not alter the embryo sensitivity to ABA and GA which was similar in both primary and hypoxia-induced secondary dormant grains (Hoang et al., Reference Hoang, Bailly, Corbineau and Leymarie2013).
Effect of oxygen on energy metabolism and protein turnover
Effect on fermentation
Although EC has been cited as being essential in the regulation of germination (Raymond and Pradet, Reference Raymond and Pradet1980; Raymond et al., Reference Raymond, Al-Ani and Pradet1985), dormancy in oat does not result from an inability to synthesize ATP from ADP and AMP (Côme and Corbineau, Reference Côme, Corbineau and Taylorson1989). During seed incubation at 30°C, a temperature at which dormant grains cannot germinate, embryos from dormant and non-dormant grains exhibited a similar increase in the ATP/ADP ratio and in the EC [(ATP + 0.5 ADP)/(ATP + ADP + AMP)] as long as seeds had not germinated (Côme et al., Reference Côme, Corbineau and Lecat1988). In anoxia (nitrogen) or hypoxia (1% oxygen), the immediate response at the level of mitochondria corresponded to a decrease in ATP synthesis and in the EC within 10 min (Lecat, Reference Lecat1987; Table 4), the secondary responses resulted in an increase of EC that was stabilized after 1 to 3 h due to fermentation and accumulation of ethanol in starchy seeds (Tables 4 and 5) (Pradet and Raymond, Reference Pradet and Raymond1983; Raymond et al., Reference Raymond, Al-Ani and Pradet1985; Lecat, Reference Lecat1987). Ethanol is the major product of fermentation in higher plants whether they are tolerant to anoxia or not (Raymond et al., Reference Raymond, Al-Ani and Pradet1983, Reference Raymond, Al-Ani and Pradet1985; Ricard et al., Reference Ricard, Couée, Raymond, Saglio, Saint-Ges and Pradet1994). Late responses leading to tolerance to oxygen deprivation were associated with a decrease in ADH activity and a change in synthesis of malate, succinate, lactate and shikimate resulting from amino-acid metabolism instead of ethanol (Raymond et al., Reference Raymond, Al-Ani and Pradet1985; Bui et al., Reference Bui, Novi, Lombardi, Lannuzzi, Rossi, Santaniello, Mensuali, Corbineau, Giuntoli, Perata, Zaffagnini and Licausi2019). In higher plants, l-lactate is often produced prior to ethanol in the first min after the transfer to anoxia, and alanine is the third major fermentation product in plants. The adenylate EC under anoxia depends on the seed reserves; in group I (lipid seeds including lettuce, sunflower, radish, turnip, cabbage, flax and soybean), germination does not occur in oxygen pressure below 1 kPa and the EC is below 0.4 while it remains at a higher value (0.70–0.82) in seeds from group II (i.e. starch seeds as rice, wheat, maize, sorghum and pea) for which germination still occurs at 0.1 kPa (Table 5; Al-Ani et al., Reference Al-Ani, Bruzau, Raymond, Saint-Ges, Leblanc and Pradet1985).
Table 4. Change in energy charge (EC) and metabolic responses in oat embryos placed in anoxia (0% oxygen) or hypoxia (1% oxygen) after 8 h incubation of oat grains on water at 30°C and in air
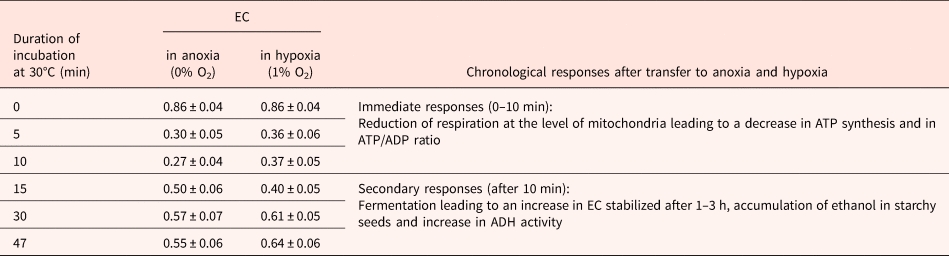
Grains harvested in 1984. Mean of 3 measurements ± SD.
ADH, alcohol dehydrogenase.
Modified from Lecat (Reference Lecat1987).
Table 5. Effects of a 3-h incubation in anoxia at 20°C on the energy charge (EC) and ethanol accumulated in seeds
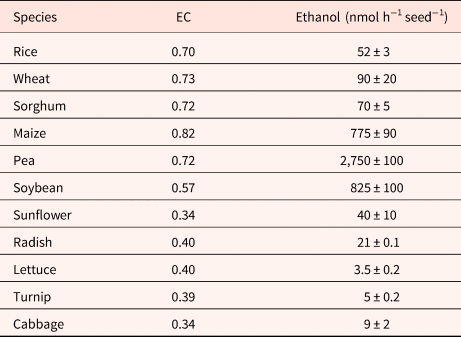
Modified from Pradet and Raymond (Reference Pradet and Raymond1983) and Raymond et al. (Reference Raymond, Al-Ani and Pradet1985).
In contrast, change in non-adenylic triphosphate nucleotides (NTP) content within the embryo during seed incubation at 30°C depended on the dormancy state (Corbineau et al., Reference Corbineau, Vinel, Leymarie, Côme, Adkins, Ashmore and Navie2007). Thermo-dormant grains lost their ability to synthesize NTP from adenine after transfer from 30 to 20°C; the capacity of synthesis of NTPs seems then to be a good marker of dormancy in oat seeds, as in buds of Helianthus tuberosus L. tubers (Le Floc'h and Lafleuriel, Reference Le Floc'h and Lafleuriel1981), of Fraxinus excelsior L. (Lavarenne et al., Reference Lavarenne, Champciaux, Barnola and Gendraud1982), Quercus spp. (Barnola et al., Reference Barnola, Crochet, Payan, Gendraud and Lavarenne1986) and Prunus persica L. (Balandier et al., Reference Balandier, Gendraud, Rageau, Bonhomme, Richard and Parisot1993).
In addition, modulation of EC using NaN3 and polyethylene-glycol (PEG) has demonstrated that the induction of thermodormancy in oat seeds is an active phenomenon requiring an EC higher than 0.5 (Corbineau et al., Reference Corbineau, Vinel, Leymarie, Côme, Adkins, Ashmore and Navie2007). Exogenous GA strongly stimulated germination of oat seeds at high temperature and in hypoxia (Lecat et al., Reference Lecat, Corbineau and Côme1992) but has no effect on EC.
Involvement of the N-end rule pathway
The ubiquitin-proteasome system (UPS) degrades the proteins by targeting specific signals known as degrons. Among the degradation signals, N-terminus is the one targeted at the N-terminal residues rather than the sequence elements of the protein (Bachmair et al., Reference Bachmair, Finley and Varshavsky1986). The N-end rule pathway has three branches: the Arg/N-end rule pathway, the Ac/N-end rule pathway and the Pro/N-end rule pathway (Lee et al., Reference Lee, Heo, Kim and Hwang2016; Nguyen et al., Reference Nguyen, Mun, Lee and Hwang2018, Reference Nguyen, Lee, Mun, Truong, Park and Hwang2019; Dong et al., Reference Dong, Zhang, Li, Tempel, Loppnau and Min2018, Zhang et al., Reference Zhang, Gannon, Hassall, Deery, Gibbs, Holdsworth, van der Hoorn, Lilley and Theodoulou2018a,Reference Zhang, Gannon, Jones, Rundle, Hassall, Gibbs, Holdsworth and Theodouloub). The Arg/N-end rule pathway identifies specific N-degrons such as aromatic residues (Phe, Trp, Tyr) by PROTEOLYSIS 1 (PRT1) and basic residues (Arg, His, Lys) by PRT6 in plant. Tertiary destabilizing residues (Asn, Gln and Cys) are first modified, either enzymatically (by deamidation of Asn or Gln) or chemically (by oxidation of Cys), to generate a secondary destabilizing residue (Asp, Glu and oxidized Cys). Studies reviewed by Licausi (Reference Licausi2011, Reference Licausi2013), Voesenek and Bailey-Serres (Reference Voesenek and Bailey-Serres2015) and Loreti and Perata (Reference Loreti and Perata2020) indicate that the sensory system to hypoxia relies on an oxygen-mediated branch of the N-end rule pathway for protein degradation acting on a specific clade of ethylene-responsive transcription factor (ERF-VII). Both the N-end rule pathway and ERF-VII are components of the oxygen signalling pathway, in particular involved in responses to low oxygen stress (Gibbs et al., Reference Gibbs, Lee, Isa, Gramuglia, Fukao, Bassel, Correia, Corbineau, Theodoulou, Bailey-Serres and Holdsworth2011, Reference Gibbs, Conde, Berckhan, Prasad, Mendiondo and Holdsworth2015; Licausi, Reference Licausi2011, Reference Licausi2013; Licausi et al., Reference Licausi, Kosmacz, Weits, Giuntoli, Giorgi, Voesenek, Perata and Van Dongen2011, Reference Licausi, Pucciariello and Perata2013; Hartman et al., Reference Hartman, Sasidharan and Voesenek2021). Group VII ERF transcription factors are either constitutively expressed and/or differentially transcriptionally regulated in response to different signals including low oxygen (Bailey-Serres et al., Reference Bailey-Serres, Fukao, Gibbs, Holdsworth, Lee, Licausi, Perata, Voesenek and Van Dongen2012; Gibbs et al., Reference Gibbs, Bacardit, Bachmair and Holdsworth2014a,Reference Gibbs, Isa, Movahedi, Lozano-Juste, Mendiondo and Berckhanb, Reference Gibbs, Conde, Berckhan, Prasad, Mendiondo and Holdsworth2015; Voesenek and Bailey-Serres, Reference Voesenek and Bailey-Serres2015; Loreti and Perata, Reference Loreti and Perata2020; Hartman et al., Reference Hartman, Sasidharan and Voesenek2021). Four of the five ERFs, HYPOXIA RESPONSIVE1 (HRE1 and HRE2) and RELATED TO APETALA 2.2 (RAP 2.2 and RAP 2.12) are implicated in the regulation of hypoxia-responsive genes. Under normoxia, ERFs are degraded via the N-end rule pathway of proteolysis. When oxygen becomes limiting, the degradation of the ERFs by the N-end rule pathway is inhibited because of the reduction of oxygen-mediated Cys oxidation (Bailey-Serres et al., Reference Bailey-Serres, Fukao, Gibbs, Holdsworth, Lee, Licausi, Perata, Voesenek and Van Dongen2012; Gibbs et al., Reference Gibbs, Conde, Berckhan, Prasad, Mendiondo and Holdsworth2015; Loreti and Perata, Reference Loreti and Perata2020; Hartman et al., Reference Hartman, Sasidharan and Voesenek2021).
Concluding comments
From 1960 to 1990, seed research took the form of descriptive physiology that highlights the sensitivity of seeds to the environment. The main research topics were developed at that time in France by the team of Dr A. Pradet and Dr P. Raymond at INRA (Bordeaux) and the team of Prof D. Côme at CNRS (Meudon) and the University Pierre et Marie Curie (Paris) were on (1) the oxygen requirement for the seed germination as a function of dormancy (Figs 2 and 3; Tables 1 and 2), (2) the involvement of the seed coat in dormancy due to the interference with oxygen diffusion to the embryo through phenolic compounds oxidation (Fig. 1) and (3) the seed/seedling metabolism in response to oxygen deprivation (Tables 4 and 5). In some ways, physiological and biochemical mechanisms have become clearer, but these data do not allow to explain the signalling mechanisms involved between oxygen, ROS and hormones in the regulation of seed germination and dormancy. It was then necessary to consolidate the interfaces among physiology, biochemistry, eco-physiology, cell and molecular biology, and genetics in order to better understand the seed behaviour and its plasticity in various environmental constraints. Access to ‘omic’ tools, genetics and micro-measurements with microsensors have allowed an enormous advancement over the last 35–60 years. Figure 5 summarizes the emerging mechanisms involved in tolerance to hypoxia (reviewed by Voesenek and Bailey-Serres (Reference Voesenek and Bailey-Serres2015), Pucciariello and Perata (Reference Pucciariello and Perata2017), Bui et al. (Reference Bui, Novi, Lombardi, Lannuzzi, Rossi, Santaniello, Mensuali, Corbineau, Giuntoli, Perata, Zaffagnini and Licausi2019), Loreti and Perata (Reference Loreti and Perata2020) and Hartman et al. (Reference Hartman, Sasidharan and Voesenek2021)), regulation of dormancy by hormone synthesis and sensitivity, NO and ROS production and communication between organelles and cell compartments (Feurtado and Kermode, Reference Feurtado, Kermode, Bradford and Nonogaki2007; Footitt et al., Reference Footitt, Douterelo-Soler, Clay and Finch-Savage2011; Nonogaki, Reference Nonogaki2017, Reference Nonogaki2019; Bailly and Merendino, Reference Bailly and Merendino2021; Jurdak et al., Reference Jurdak, Launay-Avon, Paysant-le Roux and Bailly2021). In addition, ethylene which breaks dormancy (Corbineau et al., Reference Corbineau, Xia, Bailly and El-Maarouf-Bouteau2014; Wang et al., Reference Wang, Yesbergenova-Cuny, Biniek, Bailly, El-Maarouf-Bouteau and Corbineau2018, Reference Wang, Gomes, Bailly, Nambara and Corbineau2021; Wang, Reference Wang2019) was shown to increase the response to hypoxia through enhanced stability of specific transcription factors (ERFs) and is involved in mitochondria retrograde regulation (MRR) or signalling (MRS) in which alternative oxidase (AOX) is likely to have an essential role (Nonogaki, Reference Nonogaki2014; Bailly and Merendino, Reference Bailly and Merendino2021; Hartman et al., Reference Hartman, Sasidharan and Voesenek2021; Jurdak et al., Reference Jurdak, Launay-Avon, Paysant-le Roux and Bailly2021). These recent data point out the role of mitochondria as a sensor organelle that regulates ROS production, and the control of oxygen sensing by the Cys branch of the N-degron pathway. Under hypoxia ATP synthesis is reduced but ERF-VII are stabilized and migrated to the nucleus and then activated the transcription of hypoxia-responsive genes (Gibbs et al., Reference Gibbs, Lee, Isa, Gramuglia, Fukao, Bassel, Correia, Corbineau, Theodoulou, Bailey-Serres and Holdsworth2011, Reference Gibbs, Isa, Movahedi, Lozano-Juste, Mendiondo and Berckhan2014b; Licausi et al., Reference Licausi, Kosmacz, Weits, Giuntoli, Giorgi, Voesenek, Perata and Van Dongen2011, Reference Licausi, Pucciariello and Perata2013; Pucciariello and Perata, Reference Pucciariello and Perata2017; Loreti and Perata, Reference Loreti and Perata2020; Hartman et al., Reference Hartman, Sasidharan and Voesenek2021). Better understanding of the ROS trafficking and interorganelle communication will bring novel insights in the regulation of germination and seedling emergence by the environmental conditions, and in particular by oxygen (Nonogaki, Reference Nonogaki2014, Reference Nonogaki2017, Reference Nonogaki2019; Bailly and Merendino, Reference Bailly and Merendino2021). Despite the progress of the last 30 years, numerous questions remain under debate. For example, it would be essential to characterize the ROS targets and the oxidative modifications using proteomic and/or transcriptomic approaches. The better understanding of the role of ROS in seed germination and dormancy also requires to determine the dynamics of ROS generation at the subcellular level, where ROS are produced and sensed, and how ROS level is regulated through detoxication systems in which the covering structure could be involved. It would be of particular interest to investigate how nuclear ROS may regulate seed dormancy by inducing specific transcriptomic effect.
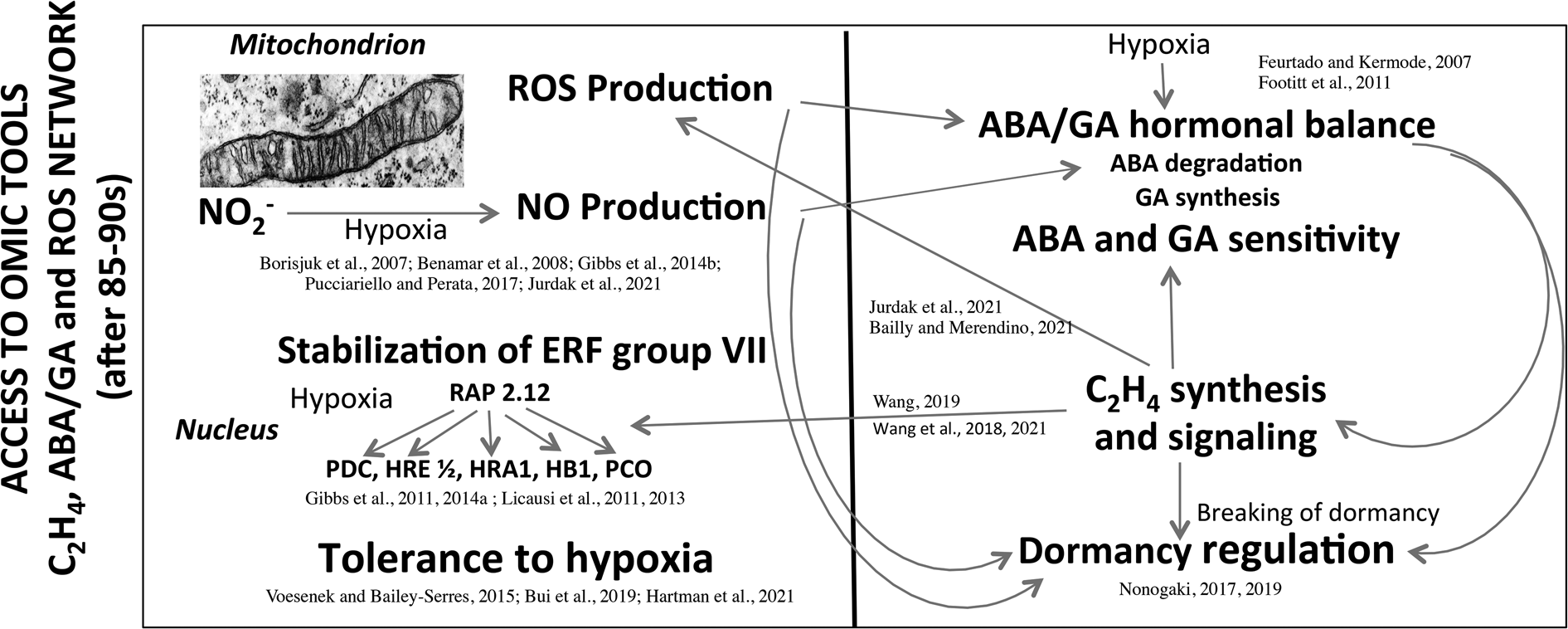
Fig. 5. Main data obtained after the 85–90s concerning the network between hormones and ROS production involved in the dormancy regulation, and the involvement of hypoxia in stabilization of ERF group VII and tolerance to oxygen deprivation. The left part of the panel highlights the involvement of hypoxia in ROS and NO production in mitochondria and the stabilization of ERFs because of the absence of oxygen. ERFs migrate then to the nucleus and activate the transcription of hypoxia-response genes important for the tolerance to hypoxia. The right part of the panel indicates the effects of hypoxia on ABA and GA balance (ABA degradation and GA synthesis) and sensitivity, and the regulation of dormancy. The relative level of ABA to GAs is a major determinant of the depth of dormancy, in addition C2H4 which improves germination of dormant seeds contributes to a decrease in ABA level, in modification in ABA and GA sensitivity, and on ROS production at the level of mitochondrion, and the stabilization of ERFs. NO induces a decrease in ABA level correlated with the regulation of ABA 8′-hydroxylase expression. ABA, abscisic acid; GA, gibberellins; ERF, ethylene response factor; RAP2.12, related to Apetala 2 12; ROS, reactive oxygen species; PDC, pyruvate decarboxylase; HRE, hypoxia responsive; HRA, hypoxia-response attenuator1; HB1, haemoglobin 1 ; PCO, plant cysteine oxidases. Modified from Borisjuk et al. (Reference Borisjuk, Macherel, Benamar, Wobus and Rolletschek2007), Feurtado and Kermode (Reference Feurtado, Kermode, Bradford and Nonogaki2007), Benamar et al. (Reference Benamar, Rolletschek, Borisjuk, Avelange-Macherel, Curien, Mostefai, Andriantsitohaina and Macherel2008), Footitt et al. (Reference Footitt, Douterelo-Soler, Clay and Finch-Savage2011), Gibbs et al. (Reference Gibbs, Lee, Isa, Gramuglia, Fukao, Bassel, Correia, Corbineau, Theodoulou, Bailey-Serres and Holdsworth2011, Reference Gibbs, Bacardit, Bachmair and Holdsworth2014a,Reference Gibbs, Isa, Movahedi, Lozano-Juste, Mendiondo and Berckhanb), Licausi et al. (Reference Licausi, Kosmacz, Weits, Giuntoli, Giorgi, Voesenek, Perata and Van Dongen2011, Reference Licausi, Pucciariello and Perata2013), Voesenek and Bailey-Serres (Reference Voesenek and Bailey-Serres2015), Pucciariello and Perata (Reference Pucciariello and Perata2017), Nonogaki (Reference Nonogaki2017, Reference Nonogaki2019), Bui et al. (Reference Bui, Novi, Lombardi, Lannuzzi, Rossi, Santaniello, Mensuali, Corbineau, Giuntoli, Perata, Zaffagnini and Licausi2019), Wang et al. (Reference Wang, Yesbergenova-Cuny, Biniek, Bailly, El-Maarouf-Bouteau and Corbineau2018, Reference Wang, Gomes, Bailly, Nambara and Corbineau2021), Wang (Reference Wang2019), Bailly and Merendino (Reference Bailly and Merendino2021), Hartman et al. (Reference Hartman, Sasidharan and Voesenek2021) and Jurdak et al. (Reference Jurdak, Launay-Avon, Paysant-le Roux and Bailly2021).