The liver plays a critical role in the defence against the entry of bacteria and bacterial products, such as lipopolysaccharide (LPS), in animals. It is the final barrier that prevents the entry of exogenous toxic substances into the systemic blood stream( Reference Nakao, Taki and Yasui 1 ). Human and animal hepatic diseases are usually accompanied by elevated levels of endotoxins in the blood. LPS is a component of the outer membrane of Gram-negative bacteria, and LPS-induced effects in vivo are initiated by the stimulation of Kupffer cells, followed by the subsequent release of inflammatory mediators (such as IL-1, IL-6 and TNF-α)( Reference He, Noda and Sugiyama 2 ) and radical oxygen intermediates( Reference Su 3 ). These mediators further stimulate the immune system to protect the body from the effects of LPS. However, when they are produced in large amounts, the LPS-induced mediators may result in liver injury( Reference Masaki, Chiba and Tatsukawa 4 ). In many models of liver injury, elevated levels of pro-inflammatory cytokines are correlated with the extent of damage( Reference Czaja, Flanders and Biempica 5 , Reference Kamimura and Tsukamoto 6 ). The inhibition of the actions of these cytokines can improve liver function( Reference Su 3 ). In addition, hepatic reactive oxygen species (ROS) have been shown to play an important pathogenic role in liver disease( Reference Bellezzo, Leingang and Bulla 7 ). ROS can aggravate LPS-induced production of TNF-α( Reference Chaudhri and Clark 8 ). Therefore, antioxidants may beneficially alleviate liver injury by scavenging ROS and indirectly reducing the release of pro-inflammatory cytokines.
N-Acetylcysteine (NAC) is a precursor of l-cysteine, which is utilised for the synthesis of reduced glutathione (GSH)( Reference Wu, Fang and Yang 9 , Reference Wu 10 ). NAC can directly react with oxidative metabolites and protect cells from oxidative damage and can indirectly exert antioxidative effects via the synthesis of glutathione, which is an essential substance for cellular defence against oxidative injury( Reference Wu, Fang and Yang 9 , Reference Fishbane, Durham and Marzo 11 ). Therefore, NAC has positive effects on detoxification and prevents oxidative stress and diseases, including acetaminophen-induced liver toxicity, metal toxicity, cancer, heart disorders and immunodeficiency virus infection( Reference Yu 12 ). We have recently reported that dietary NAC suppresses LPS-induced inflammation, oxidative stress and mucosal damage in the small intestine by regulating redox, epidermal growth factor and Toll-like receptor signalling( Reference Hou, Wang and Zhang 13 , 14 ). To extend the findings of the previous study, the present study was carried out to determine whether NAC had beneficial effects on the hepatic injury induced by a LPS challenge in piglets.
Materials and methods
Experimental design
The animal use protocol for the present study was approved by the Animal Care and Use Committee of Hubei Province. A total of eighteen cross-bred healthy female piglets (Duroc × Landrace × Yorkshire) were reared by sows and then weaned at 28 (sd 2) d of age. After a 7 d adaptation period, piglets aged 35 (sd 2) d with an average body weight of 11·58 (sd 0·26) kg were housed individually in stainless-steel metabolism cages (1·20 × 1·10 m2) and maintained at an ambient temperature of 22–25°C( 14 – Reference Hou, Yao and Wang 16 ). The piglets had free access to food and water. A maize- and soyabean meal-based diet (Table 1) was formulated to meet the National Research Council (1998)-recommended requirements for all nutrients( Reference Hou, Wang and Ding 17 ). The dietary contents of DM, fat, crude fibre, ash, crude protein, Ca and total P were determined according to the Weende method of the feed proximate analysis as described by Henneberg & Stohmann( 18 ). The dietary contents of total lysine, methionine, cystine, threonine and tryptophan were determined by HPLC( Reference Li, Rezaei and Li 19 ).
Table 1 Composition and nutrient contents of the basal diet (air-dried basis)

* Steam-dried fishmeal was produced in the USA and contained 66 % crude protein.
† Premix provided the following amounts of vitamins and trace minerals per kg of the complete diet: Fe, 100 mg (FeSO4·H2O); Cu, 150 mg (CuSO4·5H2O); Mn, 40 mg (MnSO4·5H2O); Zn, 100 mg (ZnSO4·7H2O); I, 0·5 mg (KI); Se, 0·3 mg (Na2SeO3·5H2O); retinol acetate, 3·66 mg; cholecalciferol, 0·10 mg; dl-α-tocopheryl acetate, 36·4 mg; menadione, 4 mg; thiamin, 6 mg; riboflavin, 12 mg; pyridoxine, 6 mg; cyanocobalamin, 0·05 mg; biotin, 0·2 mg; folic acid, 2 mg; niacin, 50 mg; d-calcium pantothenate, 25 mg.
‡ Analysed value.
§ Calculated value.
The experimental design of the present study is outlined in Fig. 1. All the piglets had free access to the basal diet during the 7 d adaptation period. At 35 d of age, the piglets were randomly assigned to one of the three groups: (1) control group (non-challenged control, piglets that were fed the basal diet and intraperitoneally administered sterile saline); (2) LPS group (LPS-challenged control, piglets that were fed the basal diet and intraperitoneally administered Escherichia coli LPS); (3) NAC group (LPS+500 mg/kg NAC, piglets that were fed the basal diet supplemented with 500 mg/kg NAC and intraperitoneally administered E. coli LPS). Each group consisted of six piglets. LPS was dissolved in sterile saline. NAC (powder) was mixed well with the basal diet in one-batch mixing. The diets for piglets in the control and LPS groups were supplemented with 500 mg/kg maize starch to obtain approximately isoenergetic diets( Reference Hou, Wang and Zhang 13 ). The dosage of 500 mg/kg NAC was chosen according to our previous studies that dietary supplementation with 500 mg/kg NAC exerted positive effects on the small intestine in the LPS-challenged piglets( Reference Hou, Wang and Zhang 13 , 14 ). Due to a negligible increase of 0·0042 % N, we deemed it not necessary to use non-essential amino acids as an isonitrogenous control. On days 10, 13 and 20 of the trial, overnight fasted piglets of the LPS and NAC groups were intraperitoneally administered LPS (E. coli serotype 055: B5; Sigma Chemical, Inc.) at a dose of 100 μg/kg body weight, whereas piglets in the control group were intraperitoneally administered the same volume of sterile saline( 14 ). Piglets in the NAC group were fed the basal diet supplemented with 500 mg/kg NAC during the entire 20 d period of the trial and administered LPS on days 10, 13 and 20 of the trial. Therefore, the present study was designed as a prevention strategy( Reference Haynes, Li and Li 20 – Reference Uehara, Takahashi and Fujii 23 ) rather than as a curative therapy( Reference Boutry, Matsumoto and Bos 24 ). The present study was based on the previous studies of Haynes et al. ( Reference Haynes, Li and Li 20 ) and Kessel et al. ( Reference Kessel, Toube and Pavlotzky 21 ). During the pre-challenge period (days 0–10 of the trial), all the piglets had free access to food and drinking-water. To exclude a possible effect of LPS-induced reduction in food intake on liver function, the control and NAC piglets were pair-fed the same amount of feed per kg body weight as the LPS-treated piglets during the post-challenge period (days 10–16 of the trial). All the piglets were killed under anaesthesia with an intravenous injection of pentobarbital sodium (50 mg/kg body weight) 24 h after the administration of LPS or saline (day 21 of the trial) for collecting liver samples( Reference Hou, Wang and Zhang 13 , 14 ).

Fig. 1 Scheme of the experimental design. After a 7 d adaptation period, eighteen piglets were randomly assigned to one of the three groups: control group (non-challenged control, piglets that were fed the basal diet and intraperitoneally administered sterile saline); LPS group (lipopolysaccharide (LPS)-challenged control, piglets that were fed the basal diet and intraperitoneally administered Escherichia coli LPS); NAC group (LPS+500 mg/kg N-acetylcysteine (NAC), piglets that were fed the basal diet supplemented with 500 mg/kg NAC and intraperitoneally administered E. coli LPS). On days (D) 10, 13 and 20 of the trial, piglets in the LPS and NAC groups were intraperitoneally administered a LPS at a dose of 100 μg/kg body weight, whereas piglets in the control group were administered the same volume of sterile saline. On D20 of the trial, 3 h after LPS or saline administration, blood samples were collected. On D21 of the trial, all the piglets were killed and liver samples were collected.
Collection of blood samples
On day 20 of the trial, 3 h after LPS or saline injection, blood samples were collected from the anterior vena cava into heparinised vacuum tubes (Becton-Dickinson Vacutainer System) as described previously( 14 ). Blood samples (7 ml) were centrifuged at 3000 rpm for 10 min at 4°C to obtain plasma, which was stored at − 80°C until analysis.
Collection and morphological analysis of liver samples
The abdomens of the piglets were surgically opened immediately from the sternum to the pubis and livers were collected without the cholecyst. Liver samples (approximately 5 g) were collected from the left lobe of the livers and rinsed thoroughly with ice-cold PBS (pH 7·4) to remove blood contamination. The liver samples were then rapidly frozen in liquid N2 and stored at − 80°C for further analysis. All the samples were collected within 15 min of killing.
For histomorphological analysis of the liver samples, 0·5 cm3 segments were cut off the livers and flushed with ice-cold PBS. Liver segments were fixed in fresh 4 % paraformaldehyde/PBS and embedded in paraffin, sectioned at 5 μm and stained with haematoxylin and eosin( Reference Li, Liu and Che 25 ). Histomorphological examination was done using a light microscope (American Optical Company, Scientific Instrument Division).
Determination of the activities of aminotransferase, antioxidative enzymes and related products in the plasma and liver
The activities of alanine aminotransferase (ALT) and aspartate aminotransferase (AST) in the plasma were determined using commercially available kits (Nanjing Jiancheng Bioengineering Institute). Plasma and liver samples were used for the analysis of the activities of antioxidative enzymes and related products. Specifically, the activity of superoxide dismutase (SOD) and the concentration of malondialdehyde in the plasma and liver were determined as described previously( Reference Yi, Zeng and Guo 26 , Reference Yi, Zeng and Guo 27 ). The activities of GSH and oxidised glutathione were determined using commercially available kits (Beyotime Institute of Biotechnology). The activities of catalase (CAT) and glutathione peroxidase (GSH-Px) and the concentration of H2O2 were measured using commercially available kits (Nanjing Jiancheng Bioengineering Institute) according to the manufacturer's recommendations. The assays were performed in triplicate( 14 ).
Concentrations of pro-inflammatory cytokines in the plasma and liver
The concentrations of pro-inflammatory cytokines in the plasma and liver were determined as described previously( 14 ). Briefly, frozen liver samples were homogenised, and the homogenates were centrifuged to obtain the supernatant fluid. The concentrations of TNF-α, IL-6 and PGE2 in the plasma and liver supernatant fluid were determined using commercially available 125I kits (Beijing North Institute of Biological Technology). The detection limit for the TNF-α analysis was 0·3 ng/ml, and the intra- and inter-assay CV were 5 and 8 %. The detection limits for the IL-6 and PGE2 analyses were 5·0 and 0·12 pg/ml, respectively. The CV for intra- and inter-assays were less than 7 and 15 % for the IL-6 analysis, respectively, and less than 7·5 and 10·5 % for the PGE2 analysis.
Determination of the concentrations of ATP, ADP and AMP in the liver
The concentrations of ATP, ADP and AMP in the liver were determined using HPLC as described previously( Reference Hou, Yao and Wang 16 ). Briefly, liver samples were homogenised with 1·5 m-perchloric acid in an ice bath and then centrifuged to obtain a supernatant fluid. An aliquot of the supernatant fluid was neutralised with 2 m-potassium carbonate and then centrifuged to obtain a supernatant fluid for analysis using the Waters Breeze HPLC system (Waters Corporation) and an analytical column (Waters XBridge C18, 5 μm, 4·6 mm × 150 mm; Waters Corporation). The detection wavelength was 260 nm, the pump flow rate was 1·0 ml/min and the column temperature was 35°C. Each thawed sample was filtered through a 0·20 μm filter membrane and the injection volume used was 10 μl. Peaks were identified by their retention time using authentic standards (Sigma Chemical, Inc.). Total adenine nucleotide and adenylate energy charges (AEC) were calculated according to the following equation( Reference Atkinson 28 ):

Western blot analysis
The protein contents of heat shock protein 70 (HSP70), NF-κB, AMP-activated protein kinase (AMPK) and phosphorylated AMPK (p-AMPK) in the liver were determined using Western blot analysis as described by Hou( 14 , Reference Hou, Yao and Wang 16 ). Briefly, frozen liver samples were powdered and homogenised in 1 ml lysis buffer (20 mm-HEPES, pH 7·4, 2 mm-EGTA, 50 mm-NaF, 100 mm-KCl, 0·2 mm-EDTA, 50 mm-β-glycerophosphate, 1 mm-dithiothreitol, 0·1 mm-phenylmethylsulfonyl fluoride, 1 mm-benzamidine and 0·5 mm-sodium vanadate) with a homogeniser. After centrifugation (12 000 g , 15 min and 4°C), the supernatant fluid was aliquoted into micro-centrifuge tubes, to which a 2 × SDS sample buffer was added at a 1:1 ratio. The samples were boiled and cooled on ice before being used for Western blot analysis. Proteins (50 μg/sample for HSP70, 60 μg/sample for NF-κB and 150 μg/sample for both AMPK and p-AMPK) were separated by electrophoresis on a 10 % polyacrylamide gel and then electrophoretically transferred onto polyvinylidene difluoride membranes. Skimmed milk powder in TBST buffer (1 × Tris-buffered saline including 0·1 % Tween-20) was used to block the membranes for 1 h at room temperature. The membranes were incubated with primary antibodies overnight at 4°C: HSP70 (mouse monoclonal antibodies from Stressgen Bioreagents; dilution 1:2000 in a primary antibody dilution buffer), NF-κB p65 (mouse polyclonal antibodies from Cell Signaling Technology, Inc.; dilution 1:1000 in a primary antibody dilution buffer), AMPKα and p-AMPKα (Thr172) (rabbit monoclonal antibodies from Cell Signaling Technology, Inc.; dilution 1:1000 in a primary antibody dilution buffer) or β-actin (mouse monoclonal antibodies from Sigma Chemicals; dilution 1:5000 in a primary antibody dilution buffer). The membranes were washed with TBST and incubated for 1 h at room temperature with anti-goat (mouse) IgG horseradish peroxidase-conjugated secondary antibodies (Beijing ZhongShan Golden Bridge Biological Technology Company, Limited; 1:5000 dilution). After washing with TBST, blots on the membranes were developed using an enhanced chemiluminescence Western blotting kit (ECL-plus; Amersham Biosciences), visualised and quantified using an imaging system (Alpha Innotech FluorChem FC2).
Statistical analyses
All results are expressed as means and standard deviations and analysed using one-way ANOVA. The normality and constant variance for data were tested using Levene's test( Reference Wei, Carroll and Harden 29 ). Differences among treatment means were determined using Duncan's post hoc tests. All statistical analyses were performed using the SPSS 13.0 software (SPSS, Inc.). Possibility values < 0·05 were considered to indicate statistical significance( Reference Fu, Stromberg and Viele 30 ).
Results
Liver morphology
The morphological structure of the liver is shown in Fig. 2. The livers of piglets in the control group (Fig. 2(a)) were normal. However, in the LPS group, histopathological changes associated with liver injury were observed, which included infiltration of inflammatory leucocytes; karyolysis, karyopyknosis, vacuolation and haemorrhage of hepatocytes, and a moderately disordered arrangement of hepatic cell cords (Fig. 2(b)). The LPS-induced liver injury was attenuated by dietary NAC supplementation, as indicated by normal liver cell cords, mild vacuolation of hepatic cells, limited infiltration of inflammatory leucocytes and common structure of the liver (Fig. 2(c)).

Fig. 2 Histological examination of the liver samples of piglets obtained 24 h after lipopolysaccharide (LPS) or saline administration. Sections were stained with haematoxylin and eosin ( × 400). (a) Control group (piglets that were fed the basal diet and administered sterile saline). No obvious pathological changes were found. (b) LPS group (piglets that were fed the basal diet and challenged with LPS). Significant morphological changes were infiltration of inflammatory leucocytes (A) and karyolysis (B) and karyopyknosis (C) of hepatocytes. Vacuolation and haemorrhage of hepatocytes, as well as a moderately disordered arrangement of hepatic cell cords, also occurred. (c) NAC group (piglets that were fed the basal diet supplemented with 500 mg/kg N-acetylcysteine (NAC) and also challenged with LPS). Normal liver cell cords, mild vacuolation of hepatocytes, limited infiltration of inflammatory leucocytes and normal structure of hepatocytes were observed in the NAC group.
Aminotransferase activities in the plasma
The activities of ALT and AST in the plasma are summarised in Table 2. The LPS-challenged piglets exhibited an increase (P< 0·05) in the activities of plasma ALT and AST compared with the control group. In contrast, dietary NAC supplementation attenuated the LPS-induced increase in the activity of AST (P< 0·05) to the level observed in the control group.
Table 2 Effects of dietary N-acetylcysteine (NAC) supplementation on the activities of alanine aminotransferase (ALT) and aspartate aminotransferase (AST) in the plasma of piglets (Mean values and standard deviations, n 6)
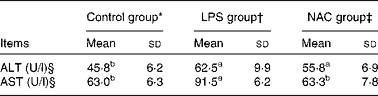
LPS, lipopolysaccharide.
a,bMean values within a row with unlike superscript letters were significantly different (P< 0·05).
* Non-challenged control, piglets that were fed the basal diet and administered saline.
† LPS-challenged control, piglets that were fed the basal diet and challenged with Escherichia coli LPS.
‡ LPS+500 mg/kg NAC, piglets that were fed the basal diet supplemented with 500 mg/kg NAC and challenged with LPS.
§ 1 U/l is defined as the amount of ALT or AST required for catalysing 1 μmol of α-ketoglutarate/min per litre plasma at 25°C.
Concentrations of inflammatory mediators in the plasma and liver
The results obtained for the concentrations of inflammatory mediators in the plasma and liver are presented in Table 3. Piglets challenged with LPS had higher (P< 0·05) concentrations of TNF-α, IL-6 and PGE2 in the plasma and liver than those in the control group. The concentrations of inflammatory mediators were decreased (P< 0·05) in the plasma and liver of piglets fed the NAC-supplemented diet.
Table 3 Effects of dietary N-acetylcysteine (NAC) supplementation on the concentrations of pro-inflammatory mediators in the plasma and liver of piglets (Mean values and standard deviations, n 6)
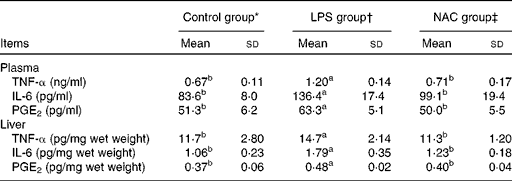
LPS, lipopolysaccharide.
a,bMean values within a row with unlike superscript letters were significantly different (P< 0·05).
* Non-challenged control, piglets that were fed the basal diet and administered saline.
† LPS-challenged control, piglets that were fed the basal diet and challenged with Escherichia coli LPS.
‡ LPS+500 mg/kg NAC, piglets that were fed the basal diet supplemented with 500 mg/kg NAC and challenged with LPS.
Antioxidative enzyme and related product activities in the plasma and liver
Data on the activities of antioxidative enzymes and related products in the plasma and liver are summarised in Table 4. Compared with the control group, the LPS-challenged piglets exhibited a decrease (P< 0·05) in the activity of SOD in the plasma and of GSH-Px in the liver, but an increase (P< 0·05) in the concentration of H2O2 in both the plasma and liver. Dietary NAC supplementation increased (P< 0·05) the activities of antioxidative enzymes (those of SOD and CAT in the plasma and liver and that of GSH-Px in the plasma) and reduced (P< 0·05) the concentrations of malondialdehyde (in the plasma and liver) and H2O2 (in the liver) in comparison with the LPS challenge. The ratio of oxidised glutathione:GSH in the plasma or liver did not differ among the three groups of piglets (P>0·05).
Table 4 Effects of dietary N-acetylcysteine (NAC) supplementation on hepatic redox status in piglets (Mean values and standard deviations, n 6)

LPS, lipopolysaccharide; SOD, superoxide dismutase; CAT, catalase; GSH-Px, glutathione peroxidase; GSSG, oxidised glutathione; GSH, reduced glutathione; MDA, malondialdehyde.
a,bMean values within a row with unlike superscript letters were significantly different (P< 0·05).
* Non-challenged control, piglets that were fed the basal diet and administered saline.
† LPS-challenged control, piglets that were fed the basal diet and challenged with Escherichia coli LPS.
‡ LPS+500 mg/kg NAC, piglets that were fed the basal diet supplemented with 500 mg/kg NAC and challenged with LPS.
§ 1 U/ml is defined as the amount of SOD, CAT or GSH-Px required for catalysing 1 μmol of superoxide (O2 −), hydrogen peroxide (H2O2) or GSH/min per ml plasma at 25°C.
∥ 1 U/mg (liver SOD) is defined as the amount of SOD required for catalysing 1 μmol of O2 −/min per mg liver protein at 25°C.
¶ 1 U/g (liver CAT and GSH-Px) is defined as the amount of CAT or GSH-Px required for catalysing 1 mol of H2O2 or GSH/min per g liver protein at 25°C.
Concentrations of ATP, ADP and AMP in the liver
In the liver, the concentrations of ATP and ADP were reduced (P< 0·05) and that of AMP was elevated (P< 0·05) in the LPS group compared with those in the control and NAC groups (Table 5). LPS treatment also increased the ratio of AMP:ATP in the liver of the LPS group than in that of the other two groups. Compared with the control group, piglets challenged with LPS exhibited a lower AEC in the liver, which was restored by dietary NAC supplementation to the level observed in the control group.
Table 5 Effects of dietary N-acetylcysteine (NAC) on the hepatic concentrations of adenylate purines in piglets (Mean values and standard deviations, n 6)
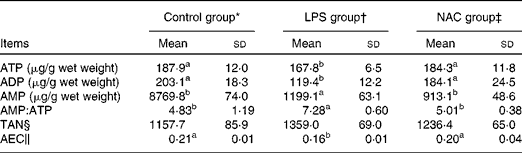
LPS, lipopolysaccharide; TAN, total adenine nucleotide; AEC, adenylate energy charge.
a,bMean values within a row with unlike superscript letters were significantly different (P< 0·05).
* Non-challenged control, piglets that were fed the basal diet and administered saline.
† LPS-challenged control, piglets that were fed the basal diet and challenged with Escherichia coli LPS.
‡ LPS+500 mg/kg NAC, piglets that were fed the basal diet supplemented with 500 mg/kg NAC and challenged with LPS.
§ TAN = ATP+ADP+AMP.
∥ AEC = (ATP+0·5 ADP)/(ATP+ADP+AMP).
Abundance of heat shock protein 70 and NF-κB p65 protein and the ratio of AMP-activated protein kinase:phosphorylated AMP-activated protein kinase in the liver
Data on the abundance of hepatic HSP70 and NF-κB p65 protein and the ratio of AMPK:p-AMPK are summarised in Fig. 3. Compared with the control group, the LPS-challenged piglets had higher (P< 0·05) concentrations of HSP70 and NF-κB p65 in the liver. However, the concentrations of these proteins were attenuated (P< 0·05) in the liver of piglets given the dietary NAC supplementation. The ratio of p-AMPK:AMPK in the liver was not altered by either LPS treatment or NAC supplementation (P>0·05).

Fig. 3 Relative levels of heat shock protein 70 (HSP70), NF-κB and phosphorylation (p) state of AMP-activated protein kinase (AMPK)-α in the liver of piglets. Liver extracts were separated by 10 % SDS–PAGE for the determination of the concentrations of HSP70, NF-κB, AMPK, p-AMPK and β-actin. Values for relative HSP70 and NF-κB abundance were normalised for β-actin. Values for p-AMPK-α abundance were normalised for total AMPK-α. Values are means (n 6), with standard deviations represented by vertical bars. Control (, non-challenged control), piglets that were fed the basal diet and administered sterile saline; LPS (
, LPS-challenged control), piglets that were fed the same control diet and challenged with Escherichia coli LPS; NAC (
, LPS+500 mg/kg NAC), piglets that were fed the basal diet supplemented with 500 mg/kg NAC and challenged with LPS. a,b,cMean values with unlike letters within the same intestinal segment were significantly different (P< 0·05).
Discussion
A well-established porcine model of liver injury induced by a LPS challenge( Reference Li, Liu and Che 25 ) was employed in the present study to investigate the potential protective effects of NAC on inflammation, antioxidative capacity and energy metabolism. We also determined the activities of plasma AST and ALT, which are non-specific and specific markers for hepatic injury, respectively( Reference He, Noda and Sugiyama 2 , Reference Matsuzaki, Kuwamura and Yamaji 31 ). The results of the present study show that dietary NAC supplementation attenuated LPS-induced increases in the activities of AST and ALT, indicating a beneficial effect of NAC in ameliorating liver injury.
The liver responds to LPS with an elevated production of cytokines and reactive oxygen intermediates, which are primarily produced by Kupffer cells( Reference Luster, Germolec and Yoshida 32 ). In many models of liver injury, a large body of evidence has indicated that increases in the concentrations of TNF-α, IL-1 and IL-6 occur and are correlated with hepatic abnormalities( Reference Kamimura and Tsukamoto 6 , Reference McClain, Hill and Schmidt 33 ). The present study showed that a LPS challenge significantly increased the concentrations of TNF-α, IL-6 and PGE2 in both the liver and blood of piglets (Table 3), probably indicating that their synthesis and release by activated immunocytes were enhanced by the LPS. The occurrence of LPS-induced liver injury was substantiated by histopathological changes including infiltration of inflammatory leucocytes; karyolysis, karyopyknosis, vacuolation and haemorrhage of hepatocytes; and a moderately disordered arrangement of hepatic cell cords in the LPS group (Fig. 2(b)). However, the inhibition of the production of pro-inflammatory cytokines can effectively modulate liver damage( Reference Su 3 ). We found that piglets fed the diet containing 500 mg/kg NAC had reduced concentrations of TNF-α and IL-6 in the blood and liver (Table 3) and reduced infiltration of inflammatory leucocytes (Fig. 2(c)) in comparison with the LPS-challenged piglets. Thus, NAC could alleviate liver injury by inhibiting the release of pro-inflammatory cytokines. This is in line with our previous studies on the small intestine( 14 ).
A critical step in the release of cytokines after a LPS challenge is the activation of the transcription factor NF-κB( Reference Muller, Ziegler-Heitbrock and Baeuerle 34 ). NF-κB is normally inactivated in the cytoplasm by binding to inhibitory κB, but it is translocated into the nucleus in response to infections, oxidants or pro-inflammatory cytokines( Reference Finco and Baldwin 35 ). This results in the transcription of target genes encoding inflammatory cytokines and mediators, which in turn further enhances the expression of NF-κB( 14 ). Therefore, the elevated abundance of liver NF-κB (Fig. 3) plays an important role in the mediation of a stimulatory effect of LPS on the production of inflammatory cytokines (IL-6 and TNF-α) in piglets. Importantly, dietary NAC supplementation reduced the concentration of NF-κB protein in the LPS-treated piglets, indicating that the decreases in the concentrations of IL-6 and TNF-α may be attributed to the inhibitory effects of NAC on the expression of NF-κB. Bellezzo et al. ( Reference Bellezzo, Leingang and Bulla 7 ) have also reported that antioxidants (NAC and α-tocopherol succinate) could suppress the activation of Kupffer cells and inhibit that of NF-κB during hepatic injury.
In addition to the release of inflammatory cytokines, the resident macrophages in the liver also produce large amounts of ROS when animals are challenged by bacterial endotoxins or pro-inflammatory cytokines( Reference Finco and Baldwin 35 ). ROS are important cytotoxic and signalling mediators in the pathophysiology of inflammatory liver disease( Reference Jaeschke 36 ). Therefore, LPS stimulates the release of inflammatory cytokines, which consequently induces the formation of ROS and related peroxides and ultimately results in the aggravation of the liver injury. This was supported by the results of the present study, demonstrating that the LPS challenge increased the concentration of H2O2 in the liver and blood of piglets (Table 4). However, the liver possesses defence mechanisms against ROS through the actions of radical scavengers, such as SOD, CAT and GSH-Px( Reference Wu, Fang and Yang 9 ). These antioxidative enzymes can cooperatively convert ROS into water and O2 ( Reference Yu 12 , Reference Zafarullah, Li and Sylvester 37 ). In the present study, the activity of SOD in the plasma and that of GSH-Px in the liver were substantially lower in the LPS group than in the control group, probably due to the reduced synthesis and enhanced degradation of these proteins. Although the activity of SOD in the liver and that of GSH-Px in the plasma tended to be lower, while the concentration of malondialdehyde tended to be higher in the LPS group than in the control group, these numerical differences did not reach statistical significance (P>0·05). Note that previous studies have reported that the hepatic levels of SOD, CAT and GSH are significantly decreased 6 or 7 h or 18 h after the administration of LPS in rats( Reference Jaeschke 36 , Reference Hsu, Chiang and Chien 38 , Reference Ben-Shaul, Sofer and Bergman 39 ). However, no difference in the activity of CAT was observed 24 or 48 h after the administration of LPS( Reference Ravikumar, Shivashangari and Devaki 40 ). Thus, the values of measured variables are likely to be affected by the sampling time. Nonetheless, dietary NAC supplementation increased the activities of SOD, CAT and GSH-Px while reducing the concentrations of H2O2 and malondialdehyde in the liver and blood of piglets. NAC can directly react with oxidants and protect cells from oxidative damage, which may be associated with its sulphydryl group (–SH). By reacting with hydroxyl radicals, NAC is converted into NAC thiol radical intermediates and finally into NAC disulphide( Reference Zafarullah, Li and Sylvester 37 ). NAC can also indirectly exert antioxidative effects by increasing the synthesis of glutathione, which is essential for cellular defence against oxidative damage( Reference Wu, Fang and Yang 9 , Reference Fishbane, Durham and Marzo 11 ). Therefore, NAC can protect the liver from oxidative damage by sparing antioxidative enzymes and also possibly by providing the cysteine precursor for the biosynthesis of GSH. We have recently reported that dietary NAC supplementation also increased the activities of SOD and CAT in the small intestines of piglets( 14 ). Taken together, these findings support the use of NAC as an effective agent to improve antioxidative capacity in animals.
In response to stress, HSP70 is expressed at elevated levels to promote refolding and prevent the aggregation of partially denatured proteins, thereby protecting cells from injury( Reference Beckmann, Mizzen and Welch 41 , Reference Kregel 42 ). This is an adaptive mechanism for allowing organisms to survive heat shock stress( Reference Hou, Wang and Ding 15 ). Therefore, a high level of HSP70 is employed as an indicator of oxidative stress in tissues( Reference Sepponen and Poso 43 ). In the present study, the protein content of HSP70 was dramatically increased in the liver of the LPS-challenged piglets, but it was restored to the normal value when the diet contained 500 mg/kg NAC, further supporting an important role of NAC in the amelioration of hepatic oxidative stress.
Another important finding of the present study is the modulation of liver energy status by dietary NAC supplementation. Previous evidence has shown that LPS causes mitochondrial dysfunction and impairs oxidative metabolism in the liver and the whole body( Reference Menguy 44 , Reference Sugino, Dohi and Yamada 45 ). Specifically, LPS administration reduces the hepatic concentration of ATP and increases the levels of lipid peroxide in rodents( Reference Lancaster, Laster and Gooding 46 ). These observations are supported by the results of the present study in which the concentrations of both ATP and ADP were decreased, but that of AMP was elevated, in the liver of the LPS-challenged piglets (Table 5). Because the energy charge of the adenyl pool is considered a more sensitive index of the energy state in a tissue than the level of a single nucleotide( Reference Atkinson 28 ), we determined the energy charge in the liver of piglets. We observed that LPS treatment reduced AEC and increased the ratio of AMP:ATP, indicating LPS-induced alterations in the cellular energy status in the porcine liver. Thus, NAC could modulate the adenine nucleotide pool and improve the liver mitochondrial function. The underlying mechanism(s) of these beneficial effects may not be associated with AMPK signalling, since the ratio of p-AMPK:AMPK did not differ among the three groups of piglets (Fig. 3). Lancaster et al. ( Reference Lancaster, Laster and Gooding 46 ) reported that LPS directly or indirectly (by inducing the release of secondary factor TNF-α) inhibits the mitochondrial function of hepatocytes. TNF-α further induces these cells to synthesise ROS, which is able to inhibit the enzyme complexes of the mitochondrial electron transport chain( Reference Zhang, Marcillat and Giulivi 47 ). Therefore, oxygen radical scavengers (e.g. antioxidants) could prevent the inhibitory effects of LPS on mitochondrial function and maintain energy metabolism in the liver( Reference Sugino, Dohi and Yamada 45 ). These beneficial effects of NAC on hepatic energy metabolism may be related to its capacity of scavenging ROS( Reference Wu, Fang and Yang 9 ).
In conclusion, dietary supplementation of 500 mg/kg NAC alleviated liver injury in the LPS-challenged piglets. NAC supplementation reduced liver inflammation (demonstrated by the reduced levels of inflammatory mediators and reduced abundance of the NF-κB p65 protein), mitigated hepatic oxidative stress (indicated by the increased activities of antioxidant enzymes, decreased production of ROS and reduced expression of HSP70) and maintained hepatic energy metabolism (based on the increases in the hepatic levels of ATP, ADP and AEC). These results have important implications for the development of new therapeutic approaches to ameliorate hepatic injury and dysfunction in animals and humans.
Acknowledgements
The present study was jointly supported by the National Basic Research Program of China (no. 2012CB126305), the Hubei Provincial Research and Development Program (no. 2010BB023), the Natural Science Foundation of Hubei Province (no. 2012FFB04805 and 2011CDA131), the Thousand-People Talent Program at China Agricultural University, Chinese Universities Scientific Fund (2012RC024), the National Research Initiative Competitive Grants from the Animal Growth and Nutrient Utilization Program (2008-35206-18764) of the USDA National Institute of Food and Agriculture and Texas AgriLife Research (H-82000). All these funders had no role in the design, analysis or writing of this article.
The authors' contributions are as follows: Y. H. and D. Y. designed the study and wrote the manuscript; L. W., B. D., Z. Y., J. L. and M. L. collected and analysed the experimental results; Y. L. and G. W. participated in the experiments and revision of the paper. All authors contributed to the data interpretation and approved the final version of the manuscript.
The authors declare that they have no conflicts of interest.