INTRODUCTION
In several European countries, intensive pig production systems produce high quantities of organic waste in limited and specific geographic areas. In Italy, the 6th Italian National Census of Agriculture (ISTAT 2012) indicates that the regions of Piedmont, Lombardy and Emilia-Romagna account for 0·90 of all pig breeding in the country (ISTAT 2012). In both Europe and Italy, slurry storage for subsequent land application is the predominant manure management practice, probably due to its simplicity, low cost and potential to reduce the total cost of crop production as a chemical fertilizer replacement (Kunz et al. Reference Kunz, Miele and Steinmetz2009). However, the technique carries several environmental pollution risks: ammonia (NH3) and greenhouse gas (GHG) emissions into the atmosphere, nitrate (NO3 −) leaching into groundwater and phosphorous (P) runoff into surface waters (Salazar et al. Reference Salazar, Chadwick, Pain, Hatch and Owen2005; Rao et al. Reference Rao, Watabe, Stewart, Millar and Moore2007; Troy et al. Reference Troy, Lawlor, O'Flynn and Healy2013; Zhu et al. Reference Zhu, Christel, Bruun and Jensen2014; Vazquez et al. Reference Vazquez, De La Varga, Plana and Soto2015). Consequently, the European Union and local authorities enforce regulations on application timings, distribution volumes and proper techniques to manage the potential environment fallout of high volumes of pig excreta generated in areas of its member countries (Berruto et al. Reference Berruto, Busato, Bochtis and Sorensen2013). At times, these rules have unintended consequences, as does the Nitrates Directive (EEC 1991) that restricts the animal manure nitrogen (N) application rate to 170 kg N/ha/year within defined Nitrate Vulnerable Zones. In this case, the mandate fails to permit manure disposal in many intensive livestock regions where cultivation occurs near farm facilities, increasing costs for storage and transportation.
Several techniques have been developed to better manage livestock slurries (Jørgensen & Jensen Reference Jørgensen and Jensen2009). The separation of solid and liquid fractions (LFs) simplifies handling by decreasing its volume. The LF, which is rich in soluble N (Fangueiro et al. Reference Fangueiro, Lopes, Surgy and Vasconcelos2012), is generally applied in areas adjacent to the farm, while the solid fraction (SF), rich in nutrients and organic matter (OM) (Fangueiro et al. Reference Fangueiro, Lopes, Surgy and Vasconcelos2012) and containing less water, can be applied to land at greater distances. According to recent investigations (unpublished data), the SF can be transported economically to fields up to 25 km from the livestock farm.
A promising approach to increase the benefits of pig slurry SF, as well as to create a potential new market for pig slurry-derived fertilizer, is to pelletize it. The densification process that occurs after composting increases the bulk density of SF from <500 to >1000 kg/m3 (Pampuro et al. Reference Pampuro, Facello and Cavallo2013), which reduces transport, handling and storage costs (Kaliyan & Vance Morey Reference Kaliyan and Vance Morey2009). Furthermore, Alemi et al. (Reference Alemi, Kianmehr and Borghaee2010) and Romano et al. (Reference Romano, Brambilla, Bisaglia, Pampuro, Foppa Pedretti and Cavallo2014) showed that pelletizing homogenizes and further concentrates SF nutrients, thereby improving its fertilizing and amending actions.
However, the high moisture content (75–80%) of fresh SF does not make it suitable for pelletizing. In previous studies (Pampuro et al. Reference Pampuro, Dinuccio, Balsari and Cavallo2014, Reference Pampuro, Dinuccio, Balsari and Cavallo2016), turning windrow composting has been revealed as a simple and cheap method to reduce the moisture content of SF. As a consequence of the heat generated by composting, after only 72 days moisture can be lowered by 40%, hence the material is suitable for pelletizing.
For optimizing the composting, a bulking agent is added to SF. This makes it possible to adjust substrate properties such as air space, moisture content, carbon-to-nitrogen ratio (C/N), particle density, pH and mechanical structure, positively affecting the decomposition rate and, therefore, development of the temperature (Bernal et al. Reference Bernal, Alburquerque and Moral2009). Lignocellulosic agricultural and forestry by-products are typical bulking agents when composting N-rich wastes such as animal manures (Bernal et al. Reference Bernal, Alburquerque and Moral2009). Their low moisture and high C/N ratios can improve the benefits of animal manures (Nolan et al. Reference Nolan, Troy, Healy, Kwapinski, Leahy and Lawlor2011). The most commonly used materials are cereal straw, cotton waste and wood by-products (Ros et al. Reference Ros, Garcia and Hernández2006; Bernal et al. Reference Bernal, Alburquerque and Moral2009; Nolan et al. Reference Nolan, Troy, Healy, Kwapinski, Leahy and Lawlor2011; Santos et al. Reference Santos, Bustamante, Tortosa, Moral and Bernal2016).
The current work aimed to determine the fertilizer properties, as well as the potential benefit to improve soil properties and to reduce NH3 volatilization and GHG emissions of pig slurry SF pellets. Different techniques for SF-based pellet fertilizer production, including addition of a bulking agent for composting, preparation of different pellet sizes and use of different soil application methods, were investigated and tested within two separate mesocosm experiments to control environmental conditions.
Several hypotheses have been formed: (1) compost derived from pig slurry SF can have a significant short-term benefit as a fertilizer (not as an amendment only); (2) fertilizer properties of SF-based pellets are not compromised by the addition of a bulking agent for composting; (3) reducing pellet diameter increases the availability of nitrogen: phosphorus: potassium (NPK), and NH3 volatilization and GHG emissions simultaneously; (4) soil-incorporated pellets, as opposed to those applied superficially, reduce NH3 volatilization and GHG emissions while increasing nutrient availability.
MATERIALS AND METHODS
Pellet preparation and characterization
Two different composts were produced from the same SF obtained from a screw press separator. The pig SF compost (SSFC) was obtained by composting 6000 kg of pig SF, while the wood chip compost (WCC) resulted after composting 8000 kg of the same pig SF with 2400 kg of wood chips processed from urban garden pruning residues. During WCC windrow preparation, materials were mixed thoroughly to achieve a theoretical C/N ratio equal to 30 (Bishop & Godfrey Reference Bishop and Godfrey1983), so as to optimize composting performance (Bernal et al. Reference Bernal, Alburquerque and Moral2009). After the set-up, windrows were placed on a concrete floor and the process was monitored for 130 days. The temperature of each SF windrow was recorded continuously with three sets of thermocouple sensors (type K) connected to a multi-channel acquisition system (Grant, mod. SQ 1600). Each set consisted of two thermocouples placed at depths of 0·2 m (T1) and 0·6 m (T2) from the windrow surface. Daily air temperatures were monitored and recorded (Fig. 1).

Fig. 1. Temperature trends (°C) recorded during the composting trial (daily average).
During the experimental period, windrows were turned six times (on days 7, 16, 28, 35, 50 and 71).
The two composts were pelletized to two different diameters ([Ø] 6 and 8 mm) by a mechanical pelletizer (CLM200E, La Meccanica Srl, Padua, Italy).
A number of analyses were performed to characterize the four pellet types (two diameters of two compost types): pH, moisture content, dry matter content (DM), total organic carbon (TOC), total nitrogen (TN), ammonium nitrogen (NH4 +-N), nitric nitrogen (NO3 −-N), C/N, OM, cation exchange capacity (CEC), total phosphorous (expressed as P2O5) and total potassium (expressed as K2O). The pH value was determined in a water-soluble extract 1 : 10 (w/w) using a Hanna HI 9026 portable pH meter fitted with a glass electrode combined with a thermal automatic compensation system. Dry matter was calculated after drying at 105 °C for 12 h and OM content by loss on ignition at 430 °C for 24 h (Navarro et al. Reference Navarro, Cegarra, Roig and Garcia1993). Samples for TOC analysis were prepared by drying the samples at 105 °C for 24 h, followed by treatment with sulphuric acid to eliminate any inorganic C, with subsequent analysis on an elemental analyser (Carlo Erba Instruments). Total N and NH4 +-N were determined using the Kjeldahl standard method. Nitric-N was determined by ion chromatography in a 1 : 20 (w/v) water extract (Garcia-Gomez et al. Reference Garcia-Gomez, Bernal and Roig2002); CEC was determined by sodium chloride adsorption followed by the potassium nitrate displacement method (Silber et al. Reference Silber, Bar-Yosef, Levkovitch and Soryano2010). After HNO3/HClO4 digestion, P2O5 was analysed by colourimetry and K2O by flame photometry (Garcia-Gomez et al. Reference Garcia-Gomez, Bernal and Roig2002). Table 1 reports the main chemical characteristics (mean value of three replicates) for the pellets investigated.
Table 1. Main properties of the two pellet types included in the experiment

SSFC, pig slurry solid fraction (SF) compost without a bulking agent; WCC, pig SF compost with wood chips as the bulking agent; TN, total nitrogen; NH4 +-N, ammonium nitrogen; NO3 −-N, nitric nitrogen; TOC, total organic carbon; C/N, carbon to nitrogen ratio; OM, organic matter; CEC, cation exchange capacity; P2O5, total phosphorous; K2O, total potassium.
Number of replicates = 3.
Fertilizer value experiment
A mesocosm experiment was set up in a controlled environment (22 °C) glasshouse to test the fertilizer value of the different pig SF-based pellets in a randomized complete block design with four replicates. The experiment included a total of ten treatments: (1) SSFC Ø 6 mm superficially distributed [SSFC 6 SUP]; (2) SSFC Ø 8 mm superficially distributed [SSFC 8 SUP]; (3) SSFC Ø 6 mm mixed with the soil [SSFC 6 MIX]; (4) SSFC Ø 8 mm mixed with the soil [SSFC 8 MIX]; (5) WCC Ø 6 mm superficially distributed [WCC 6 SUP]; (6) WCC Ø 8 mm superficially distributed [WCC 8 SUP]; (7) WCC Ø 6 mm mixed with the soil [WCC 6 MIX]; (8) WCC Ø 8 mm mixed with the soil [WCC 8 MIX]; (9) Conventional mineral fertilization with NPK fertilizer (15–15–15) [NPK]; (10) unfertilized Control [CON].
Each experimental unit consisted of a plastic mesocosm pot (volume = 3·015 litre, diameter = 160 mm, height = 150 mm) with small holes in the bottom for excess water drainage containing clay–silty soil collected from the top 20 cm of the CEBAS–CSIC experimental fields located in Santomera, Murcia Region (Spain). The soil was air-dried for 5–6 days and sieved to <5 mm for the mesocosm experiment. For the soil characterization analyses described above, soil was further sieved to <2 mm; results are reported in Table 2.
Table 2. Chemical properties of the soil used in the experiment
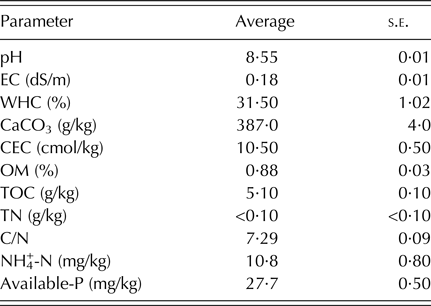
EC, electrical conductivity; WHC, water holding capacity; CaCO3, calcium carbonate; CEC, cation exchange capacity; OM, organic matter; TOC, total organic carbon; TN, total nitrogen; C/N, NH4 +-N, ammonium nitrogen; P, phosphorus.
Number of replicates = 2.
Each mesocosm was uniformly packed with 3 l of soil at a bulk density of 1350 kg/m3 (Wu et al. Reference Wu, Huang and Warrington2011). Initially, all pots were moistened with deionized water to attain a 60% water-filled pore space (WFPS). The water added to each mesocosm was calculated to supply 70% of the water holding capacity (WHC), which corresponded to 670 ml per pot. Thereafter, soil water content was adjusted via a drip irrigation system (4 litre/min for 10 min) every 2–5 days as required for the crop. Mesocosms were fertilized manually (with SSFC or WCC or NPK mineral fertilizer) at a consistent N application rate (equivalent to 200 kg/ha). Depending on pellet composition, P and K were supplied as follows to the soil: 240 kg P2O5/ha and 60 kg K2O/ha for SSFC; 255 kg P2O5/ha and 110 kg K2O/ha for WCC; and 200 kg P2O5/ha and 200 kg K2O/ha for NPK fertilizer. Maize (Zea mays L.) FAO 500 seeds were then sown into the mesocosm pots at a density of two plants per pot. Plants grew for 65 days.
At the end of the trial, the above-ground biomass was harvested, roots were separated from the soil and the soil was sampled. After washing both the above- and below-ground biomass with tap and distilled water (two times each), all were dried at 60 °C for 72 h and sub-samples were milled to 0·5 mm for analysis and moisture content determination.
Soil pore water was sampled three times during the experiment (days 30, 60, 65) in the MIX, NPK fertilizer and unfertilized control treatments using FLEX-type ‘Rhizon’ soil pore water samplers (Rhizosphere Research Products, The Netherlands) inserted at the surface of each pot at approximately 45°. Soils were wetted to saturation (100% of their WHC) with deionized water 24 h prior to each pore water extraction to ensure soil solution equilibrium.
Nitrogen concentration was assessed by automatic microanalysis. After HNO3–H2O2 microwave-assistant digestion, P composition of the aerial parts was determined by colourimetry (Kitson & Mellon Reference Kitson and Mellon1944) and K by flame photometry. Soil samples were analysed for nitrate (NO3) by ion chromatography in a 1 : 20 (w/v) water extract, while electrical conductivity (EC) and pH were evaluated in a water-soluble extract 1 : 10 (w/v). An automatic liquid sample analyser (TOC-V CSN + TNM-1 Analyser, Shimadzu, Tokyo, Japan) was used to measure soluble N in pore water. All chemical determinations were performed in duplicate.
Plant N utilization efficiency was calculated on the basis of the apparent recovery fraction (ARF) approach (Gunnarsson et al. Reference Gunnarsson, Bengtsson and Caspersen2010), according to the following equation:

in which N uptake treatment is the total N uptake (mg/pot) of a fertilizer treatment at harvesting, N uptake control is the total N uptake (mg/pot) of the unfertilized control and TN added is the total N added to each pot (mg/pot). A similar calculation was done for P, but without subtracting P uptake of the control (Syers et al. Reference Syers, Johnston and Curtin2008).
Ammonia and greenhouse gas experiment
A second mesocosm experiment, also of a randomized complete block design with four replicates, was set up to measure NH3 volatilization and GHG emissions. Nine of the ten treatments described for the first experiment were included in this investigation; the ‘NPK treatment’ was omitted.
The experiment was carried out in glass jars (3·2 litre capacity). To mimic the plough layer (0–30 cm) of the soil, all jars were filled with 1·5 kg of the same soil used in the first mesocosm experiment; they were also moistened with deionized water to reach 60% of WFPS (Subedi et al. Reference Subedi, Kammann, Pelissetti, Sacco, Grignani, Monaco, Vallez, Cambier, Bacheley, Cheviron, Formisano, Lepeuple, Revallier and Houot2013). Next, the soil was brought back to field density (1·35 g/cm3; Wu et al. Reference Wu, Huang and Warrington2011), at which the headspace volume equalled 2000 cm3. The jars were then pre-incubated at 20 °C until the initial CO2 flux from soil re-wetting had subsided (10 days). After pre-incubation, jars were manually fertilized with either SSFC or WCC pellets with the same nutrient amounts as described in the first experiment. Thereafter, all jars were maintained in a climate-controlled room at a constant 25 °C and air humidity of about 55%. The soil moisture content of each jar was maintained at 60% WFPS for 57 days via gravimetric adjustment every 2–3 days as required. No gas measurement was taken <12 h after an adjustment.
Ammonia volatilization was measured for 48 h following pellet application at 20 °C and at an air-flow rate of 2 litre/min (Subedi et al. Reference Subedi, Kammann, Pelissetti, Sacco, Grignani, Monaco, Vallez, Cambier, Bacheley, Cheviron, Formisano, Lepeuple, Revallier and Houot2013) with a dynamic chamber system coupled with a photo-acoustic trace gas analyser (PTGA, INNOVA 1412, LumaSense Tech).
Emissions of the main GHG produced from agricultural soils (i.e., CO2, CH4 and N2O) were measured from the jars three times weekly for the first 2 weeks after fertilization, then twice weekly for the following 3 weeks and once weekly for the last 4 weeks, for a total of 16 times during the 57-day period. Greenhouse gas fluxes were measured for each sealed jar using a gas-tight polyethylene lid equipped with two Teflon tubes (each 5 cm long) punctured by several small holes (0·5 mm diameter) to sample air from the entire headspace volume. Thirty mililitres of air was withdrawn by plastic syringe from the jar headspace at 0, 9 and 18 min after jar closure. All samples were stored in airtight glass vials (12 ml Exetainer® vials) and analysed for CO2, CH4 and N2O concentrations within 24 h by gas chromatography (Agilent 7890). The gas chromatograph (GC) was equipped with thermal conductivity, flame ionization and electron capture detectors for determination of CO2, CH4 and N2O concentrations, respectively. For each jar closure, concentrations of the three GHG were plotted over time and fluxes were calculated with a linear or polynomial model, depending on their specific accumulation pattern (Subedi et al. Reference Subedi, Taupe, Pelissetti, Petruzzelli, Bertora, Leahy and Grignani2016). Cumulative emissions were estimated assuming a linear change in fluxes between adjacent sampling points.
Total gaseous losses were expressed in CO2-eq using conversion factors of 1, 28, 265 and 2·65 for CO2, CH4, N2O and NH3 (IPCC Reference Stocker, Qin, Plattner, Tignor, Allen, Boschung, Nauels, Xia, Bex and Midgley2013), respectively.
Statistical analyses
One-way analysis of variance (ANOVA) was performed to evaluate all investigated variables concerning plant, root, soil pore water, soil and cumulative NH3 and GHG emissions. A Kolmogorov–Smirnov test was used to test normality of distribution; homoscedasticity was verified with Levene's test. For each variable, if treatment effect was statistically significant, the ANOVA was followed by the planned contrasts test. Nine contrasts were planned; first, the unfertilized control against all the fertilized treatments (all pellets + NPK); then NPK against all pellet-fertilized treatments (SSFC + WCC); subsequently, SSFC pellets against WCC pellets; afterwards, within each type of pellet (both SSFC and WCC) 6 mm diameter against 8 mm diameter; finally, (within each type of pellet and each diameter) surface application against soil mixed application. For apparent recovery (AR), only eight contrasts were realized, excluding the unfertilized control.
Statistical analyses were performed by SPSS software (IBM SPSS Statistics for Windows, Version 21·0. Armonk, NY: IBM Corp.).
RESULTS
Maize biomass and nutrient concentrations
All plants in all treatments appeared healthy throughout the growing period and did not show any sign of nutrient deficiency or toxicity at any time. Fertilizer treatments significantly affected above-ground yield (P ⩽ 0·010) and NPK concentrations (P < 0·001), as well as root production (P < 0·001) and N concentration (P < 0·001) (Table 3).
Table 3. Results of analysis of variance (ANOVA) of all measured variables

DM, dry matter; N, nitrogen; P, phosphorus; K, potassium; NO3, nitrate; EC, electrical conductivity; CO2, carbon dioxide; N2O, nitrous oxide; CH4, methane; C, carbon; NS, not significant.
Significance of the treatment and standard error of the mean (s.e.m.) of the treatments. Degrees of freedom of s.e.m. = 3
Table 4 shows that after 65 days all fertilized treatments produced significantly greater yields (P < 0·001) and NPK concentrations (P < 0·001) relative to the unfertilized control, while no other difference was significant for maize yield. Pellet-fertilized maize exhibited lower N (−11%) and K (−9%) concentrations as opposed to maize fertilized with NPK mineral fertilizer, probably resulting from the lower K2O amount provided by the pellets v. the NPK fertilizer (60, 110 and 200 by SSFC, WCC and NPK fertilizer, respectively). All treatments produced similar TN levels.
Table 4. Effects of fertilization treatment on maize production and nutrient content

DM, dry matter; N, nitrogen; P, phosphorus; K, potassium; SSFC, pig slurry solid fraction (SF) compost without a bulking agent; WCC, pig SF compost with wood chips as the bulking agent; NS, not significant.
Maize N and P concentrations were significantly (P = 0·009 and P ⩽ 0·001 for N and P, respectively) influenced by characteristics of the pellet applied, as demonstrated by increased N concentrations in WCC relative to SSFC. In the case of P, plants fertilized with SSFC had the highest concentrations. A significant (P < 0·001) rise in N concentration was induced in WCC with smaller- as opposed to larger-sized pellets (6 v. 8 mm), although no such effect was detected in SSFC. Application method significantly (P ⩽ 0·001, < 0·001 and ⩽ 0·001 for SSFC 8, WCC 6 and WCC 8, respectively) influenced N concentration, as evidenced by increased N concentrations when pellets were mixed into the soil as opposed to surface-applied.
With regards to P and K, the higher P content in SSFC played a key role in increasing maize P concentration, while the high K content in WCC did not produce such an effect on the plant. Neither pellet application method nor dimension produced any important P or K effect. Alternatively, if an effect was indeed produced, it might have been countered by different interactions.
Apparent recovery of both N and P were affected by treatment (Table 5). No significant effect of pellet type relative to NPK fertilizer or of SSFC relative to WCC was detected. However, soil incorporation improved the AR of N in every tested situation and the same was observed for the small diameter relative to the large one. The AR of P was lowered by the use of pellets compared with mineral fertilizer and by WCC compared with SSFC. Soil incorporation affected the AR of P, but in dissimilar ways for pellet type and diameter. Small-sized pellets improved the AR of P only for SSFC, but not for WCC.
Table 5. Effects of fertilization treatment on apparent recovery of nitrogen (N) and phosphorus (P)

N, nitrogen; P, phosphorus; SSFC, pig slurry solid fraction (SF) compost without a bulking agent; WCC, pig SF compost with wood chips as the bulking agent; NS, not significant.
Root production (P < 0·001) and N concentration (P < 0·001) were affected significantly by treatments (Table 3), with all fertilized treatments producing a significantly greater root biomass (P < 0·001) (Table 6) compared with the control. No significant differences were observed between mineral fertilizer and pellets. Root production was stimulated when no bulking agent was used in the composting process, an effect that was significantly greater when SSFC was mixed into the soil. Smaller diameter pellets also positively affected root production.
Table 6. Effects of fertilization treatment on root production and its nitrogen content

DM, dry matter; N, nitrogen; SSFC, pig slurry solid fraction (SF) compost without a bulking agent; WCC, pig SF compost with wood chips as the bulking agent; NS, not significant.
After 65 days, highly significant differences in root N concentration were observed comparing the unfertilized control with respect of all the other treatments (P ⩽ 0·001). After the same period, root N concentrations were lower for pellet-fertilized treatments than for NPK treatment (P = 0·004).
Soil properties
No significant differences were found in soil pH, while significant treatment effects were detected for NO3 − (P < 0·001) and EC (P < 0·001) (Table 3). In particular, NO3 − and EC increased after the application of NPK mineral fertilizer with respect to pellets (Table 7). Both soil properties were affected by the type of pellet supplied and, specifically, they increased in WCC treatment. In addition, pellet diameter had an important effect on NO3 − and EC: in general, the highest values were observed with 6 mm pellets. Statistical analysis highlighted that the superficial distribution promotes the increase of NO3 − and EC. In all treatments investigated (Table 7), EC values were well below the limit for saline soil and, furthermore, soil in all the treatments can be considered non-saline (Bernal et al. Reference Bernal, Roig, Madrid and Navarro1992).
Table 7. Effects of fertilization treatment on residual soil quality
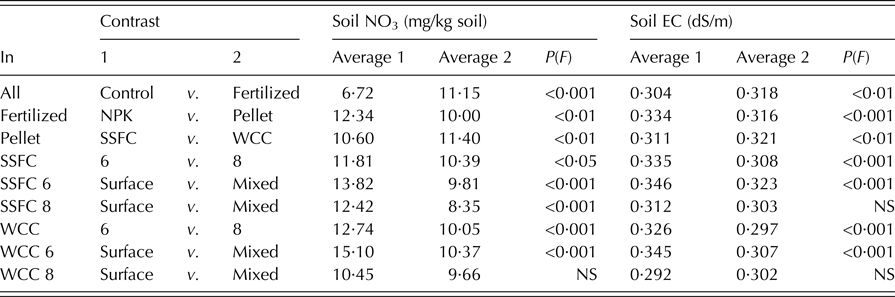
NO3, nitrate; EC, electrical conductivity; N, nitrogen; P, phosphorus; K, potassium; SSFC, pig slurry solid fraction (SF) compost without a bulking agent; WCC, pig SF compost with wood chips as the bulking agent; NS, not significant.
Soluble-N analysed in soil pore water indicated an important effect of sampling time, with the lowest concentration at the end of the experiment in all treatments (Fig. 2). With respect to the treatments, results of NPK fertilizer at the first sampling was statistically greater (P < 0·001) that the rest of the treatments, including unfertilized control.
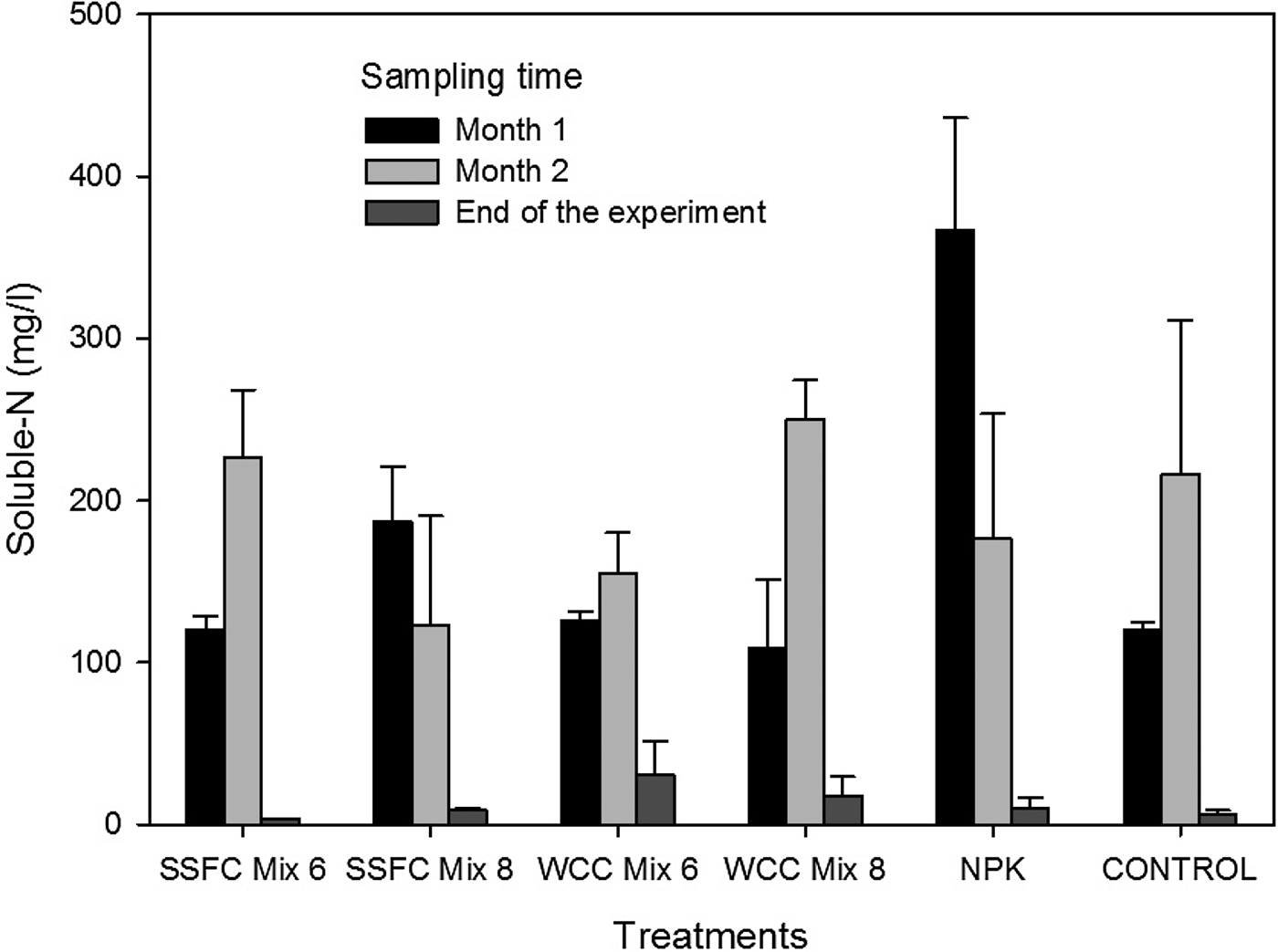
Fig. 2. Concentration of soluble nitrogen in pore water soil at different treatment with pellets mixed with the soil, nitrogen: phosphorus: potassium (NPK) fertilizer and unfertilized control during the mesocosm experiment.
Ammonia volatilization and greenhouse gas emissions
Ammonia volatilization was not detected from any of the treatments investigated (data not shown). Methane emission measurements were low and unaffected by the various treatments (data not shown), while the various treatments showed significant influence on CO2 (P < 0·001) and N2O (P = 0·002) emissions (Table 3).
During the 57-day incubation period, the unfertilized control showed the lowest CO2 emission (Table 8). No significant differences were found between SSFC and WCC treatments. However, across the SSFC treatments, significant differences (P = 0·007) were observed between the two pellets diameter sizes, with higher CO2 emissions recorded for the smaller diameter pellet. Other differences were not significant.
Table 8. Effects of fertilization treatment on carbon dioxide (CO2) and nitrous oxide (N2O) emissions

CO2, carbon dioxide; N2O, nitrous oxide; N, nitrogen; C, carbon; SSFC, pig slurry solid fraction (SF) compost without a bulking agent; WCC, pig SF compost with wood chips as the bulking agent; NS, not significant.
Number of replicates = 3.
All fertilized treatments exhibited cumulative N2O emissions significantly higher than the control (P = 0·002) (Table 8). Cumulative N2O emissions were not significantly affected by pellet type or pellet diameter; however, the statistical analysis revealed that superficial distribution reduced N2O emissions.
DISCUSSION
Pig slurry SF has been investigated in its pelletized form after composting as a fertilizer for maize crop, a technique proposed to add agronomic value while mitigating the environmental risk of conventional SF pig slurry. A set of follow-on trials tested compost type, pellet size and application method to identify optimizations of the technique.
To this end, four hypotheses were developed.
The first hypothesis tested whether compost derived from SF pig manure possessed a short-term significant fertilizer effect beyond that of its value as an amendment. The current investigation verified that the SF pig slurry pelletized compost fertilizers considered effectively increased maize biomass, NPK concentration and root N content, as well as residual soil nitrates and EC in all treatments fertilized with compost pellets, compared with the unfertilized control. The results obtained are consistent with the acknowledgement that composted SF pig slurry is an improved fertilizer product, mainly due to its large contribution of nutrients to plants, especially N and P (Pinamonti et al. Reference Pinamonti, Stringari and Zorzi1997; Atiyeh et al. Reference Atiyeh, Edwards, Subler and Metzger2001; Garcia-Gomez et al. Reference Garcia-Gomez, Bernal and Roig2002; Perez-Murcia et al. Reference Perez-Murcia, Moral, Moreno-Caselles, Perez-Espinosa and Paredes2006).
However, lower N concentrations of aerial and root biomasses, K concentrations in maize plants and residual soil nitrates in all pellet-fertilized treatments v. the NPK mineral fertilizer treatment were observed. The results obtained highlighted that pelletized treatments provided lower – possibly even inadequate – amounts of K during the growing season relative to mineral fertilizer, a finding consistent with the lower yields produced in maize fertilized with compost, compared with mineral fertilizer (Businelli et al. Reference Businelli, Gigliotti and Giusquiani1990; Bazzoffi et al. Reference Bazzoffi, Pellegrini, Rocchini, Morandi and Grasselli1998; Loecke et al. Reference Loecke, Liebman, Cambardella and Richard2004).
The results of the current investigation also confirmed that pig manure compost pellet fertilizers released N slowly when compared with standard NPK-soluble mineral fertilizer. In fact, the analysis of soil pore water during the experiment indicated a similar behaviour of soluble N (readily plant-available) in all pellet treatments, but the greater concentration found in the first sampling of NPK-fertilizer demonstrated the high solubility of the mineral fertilizer with respect to pelletized compost. At the end of the experiment, the results showed that soluble N was taken up by the crop in all treatments. As Ball et al. (Reference Ball, McTaggart and Scott2004) pointed out, the slower nutrient release of pelletized compost over time can act to reduce the risk of nutrient losses significantly. Efficiency of added N, as estimated through apparent recovery (NAR), was not statistically lower for pellets than for NPK. This difference was not determined by different yield rather than by different N concentration in plant, determining an improvement of uptake in NPK treatment. Small diameter (6 mm) pellets v. large (8 mm) pellets were shown to improve NAR values, which advances the notion that pellets did not threaten maize yield performances relative to mineral fertilizer, only that they may reduce the nutritional value of maize destined for feed purposes. It is also possible that the added N not used by plants and not present in the soil in mineral form at the end of the cropping cycle remained in the soil in stable pools as organic-N to improve soil fertility over time (Zavattaro et al. Reference Zavattaro, Assandri and Grignani2016), or was lost through leaching or gaseous emissions. Nonetheless, the fact that mixing pellets (6 mm diameter) into the soil resulted in improved NAR values relative to surface application make the second hypothesis feasible for NH3 volatilization.
For P, the AR of applied fertilizer is usually low in the first cropping year following application, when as much 0·90 of added inorganic P has been shown to become unavailable for crop nutrition due to adsorption and precipitation (Malik et al. Reference Malik, Marschner and Khan2012). The results obtained in the current study followed this trend also, with a range of ‘very low’ AR values (from 0·10 to 0·18). Even though statistical effects were identified, differences failed to permit conclusions on the fertilizer value of using pelletized composts for P nutrition.
The second hypothesis tested whether adding a bulking agent before composting failed to limit the fertilizer properties of SF-based pelletized composts. It too was verified. The results of the planned contrast test between SSFC and WCC highlighted that indeed no differences were found in plant yields, plant K concentrations, root N concentrations, or NAR values. Moreover, plant N concentration increased when WCC was applied, which suggested an improvement in availability of mineral N to plants. Increased maize root biomass was measured in SSFC relative to WCC, a result that might have been induced by lower nutrient availability and a subsequent increase in root allocation (Müller et al. Reference Müller, Schmid and Weiner2000). The supposition of high availability of nutrients in WCC is further corroborated by increased residual soil nitrates found after application of WCC instead of SSFC. These results demonstrate that the addition of a bulking agent during composting fails to reduce the fertilizer value.
The third hypothesis of the current study tested whether reduced pellet diameter resulted in increased NPK availability, as well as concurrently NH3 volatilization and GHG emissions. The hypothesis was partially verified. In the WCC treatment, the results of the planned contrast test of 6 v. 8 mm indicated that smaller diameter pellets induced increased plant N concentration and root production, in addition to soil EC, residual soil nitrates and NAR. The larger diameter increased plant P concentration alone. In SSFC treatment, the smaller diameter resulted in increased root production, soil EC and residual soil nitrates. Carbon dioxide emissions also increased, which others (Rochette et al. Reference Rochette, Angers and Côte2000; Balota et al. Reference Balota, Machineski and Truber2010) ascribe to the raised soil microbial activity when pellet diameter is smaller and the applied OM more degradable.
The last hypothesis postulated that incorporating compost pellets into the soil reduces NH3 volatilization and GHG emissions and simultaneously increases nutrient availability. This hypothesis was partially verified. The planned contrast test of mixed v. surface application highlighted that incorporating pellets into the soil greatly affected plant N concentration, root production, NAR and soil residual nitrates (reduction). Following application, each of these measures demonstrated that plant N uptake was improved except in the case of root production. Considering GHG emissions, soil mixing did not affect CO2 emissions, but induced an increase in N2O emissions as expected from the higher contact of fertilizer with soil particles and enhanced microbial degradation (Velthof et al. Reference Velthof, Kuikman and Oenema2003). Surface application played a different role in nutrient release dynamics by reducing nutrient availability to the plant, while simultaneously, increasing residual nitrates in the soil. This behaviour may be explained by late transfer of added N from the surface toward the soil (retarded or reduced solubilization of pellets) that was unmatched by plant requirements. Soil incorporation is the best technique to take advantage of the nutrients available from pellets.
CONCLUSIONS
Pelletized composted manure was shown to be an effective slow-release fertilizer for maize. The best technical options for its production include addition of a bulking agent before composting, using small diameter pellets and application with incorporation into the soil. The adoption of all these techniques results in the best availability of nutrients from pelletized composted pig manure for plant nutrition.
This work was carried out within the framework of the ‘FITRAREF’ project, funded by the Italian Ministry of Agriculture and Forestry (GRANT NUMBER, DM29638/7818/10). The authors thank CEBAS-CSIC for the infrastructure and materials made available for the mesocosm experiments run during the stay of Dr Pampuro at the Department of Soil and Water Conservation and Organic Wastes Management of CEBAS-CSIC (financed by the Spanish Ministry of Economy and Competitiveness and the European Union through FEDER funds; CTM2013-48697-C2-1-R).