INTRODUCTION
Paleosol-loess sequences have a long history of use in paleoenvironmental reconstructions for a broad range of paleoclimate, geology, biology, and anthropology investigations (e.g., Riecken, Reference Riecken1950; Fisk, Reference Fisk1951; Kyuma et al., Reference Kyuma, Wang, Tulaphitak, Yue, Araki and Miao1985; Wu et al., Reference Wu, Chen, Rousseau, Li, Pei and Wu2007; Zech et al., Reference Zech, Rass, Buggle, Loescher and Zoeller2012; Lechler et al., Reference Lechler, Huntington, Breecker, Sweeney and Schauer2018). The worldwide spatial distribution (Haase et al., Reference Haase, Fink, Haase, Ruske, Pécsi, Richter, Altermann and Jäger2007; Muhs, Reference Muhs and Elias2007; Roberts et al., Reference Roberts, Muhs, Bettis, Elias and Mock2013; Ghafarpour et al., Reference Ghafarpour, Khormali, Balsam, Karimi and Ayoubi2016) and high temporal resolution (Frechen et al., Reference Frechen, Oches and Kohfeld2003; Karimi et al., Reference Karimi, Frechen, Khademi, Kehl and Jalalian2011; Sun et al., Reference Sun, Clemens, Morrill, Lin, Wang and An2012; Taheri et al., Reference Taheri, Khormali, Wang, Amini, Wei, Kehl, Frechen and Chen2016; Moine et al., Reference Moine, Antoine, Hatte, Landais, Mathieu, Prud'homme and Rousseau2017) of paleosol-loess sequences make them an important terrestrial archive (Guo et al., Reference Guo, Ruddiman, Hao, Wu, Qiao, Zhu and Peng2002). To this end, investigating isotopic compositions of carbon (δ13Ccarb) and oxygen (δ18Ocarb) of loess materials, and in particular carbonates formed in these sequences, has been a staple of paleoenvironmental reconstructions for decades. Carbonate δ13C values provide information about paleovegetation (Quade and Cerling, Reference Quade and Cerling1995; Liu et al., Reference Liu, Guo, Wu and Lu1996; Bayat et al., Reference Bayat, Karimi and Khademi2016), and δ18O values are related to the temperature and the isotopic composition of oxygen in the soil water (δ18Owater) from which the carbonates precipitated (Cerling, Reference Cerling1984; Bayat et al., Reference Bayat, Karimi and Khademi2016; Lechler et al., Reference Lechler, Huntington, Breecker, Sweeney and Schauer2018).
More recently, carbonate clumped isotope (Δ47) thermometry has been used in terrestrial paleoclimate reconstructions (e.g., Affek, Reference Affek2012; Eiler et al., Reference Eiler, Bergquist, Bourg, Cartigny, Farquhar, Gagnon and Guo2014). Δ47 thermometry is an indicator of carbonate formation temperature independent of δ18Owater through measure of the abundance of “clumped” carbonate molecules containing both 13C and 18O in excess of the abundance that would be expected if heavy isotopes were distributed randomly (Ghosh et al., Reference Ghosh, Adkins, Affek, Balta, Guo, Schauble, Schrag and Eiler2006; Eiler, Reference Eiler2007). Recent applications of clumped isotope thermometry to study carbonates formed in or associated with paleosols and loess show the potential for using independent (paleo)temperature constraints to extract paleoclimate information from these records (Eagle et al., Reference Eagle, Risi, Mitchell, Eiler, Seibt, Neelin, Li and Tripati2013; VanDeVelde et al., Reference VanDeVelde, Bowen, Passey and Bowen2013; Garzione et al., Reference Garzione, Auerbach, Smith, Rosario, Passey, Jordan and Eiler2014; Ji et al., Reference Ji, Nie, Lechler, Huntington, Heitmann and Breecker2018; Lechler et al., Reference Lechler, Huntington, Breecker, Sweeney and Schauer2018; Zhai et al., Reference Zhai, Wang, Qin, Cui, Zhang and Ding2019).
Paleosol-loess sequences contain various carbonate types (e.g., loess dolls, nodules, rhizoliths, land-snail shells, and earthworm biospheroliths) that are formed through a variety of processes that occur over different time periods (Zamanian et al., Reference Zamanian, Pustovoytov and Kuzyakov2016a). All carbonate types that might be found in paleosol-loess sequences can be classified in three groups: (1) geogenic carbonates (i.e., “bulk” loess carbonates), which account for up to 30% of loess material; (2) biogenic carbonates, such as land-snail shells, earthworm biospheroliths, and micro mammal remains such as bones and teeth; and (3) pedogenic carbonates, such as rhizoliths, carbonate pendants, and nodules (Zamanian et al., Reference Zamanian, Pustovoytov and Kuzyakov2016a). Formation of each carbonate type is governed by the same equilibrium equations:


where calcite activity (α CaCO3) varies as a function of the concentration of calcium ions in aqueous solution (m Ca2+), the partial pressure of CO2 in the soil gas (pCO2), and temperature-sensitive equilibrium constants for the dissociation of carbonic acid (K 1), bicarbonate (K 2), and calcite (K cal), and for the hydration of CO2 (K CO2) (Drever, Reference Drever1982).
For each carbonate type, Equation 1 is driven to the left to cause CaCO3 precipitation via distinct processes that operate over different time spans and locations (Zamanian et al., Reference Zamanian, Pustovoytov and Kuzyakov2016a). In the case of biogenic carbonates, environmental conditions that promote (or restrict) biological activity, and growth must be considered. The mean lifespan of land-snails (depending on the species) is 3–5 years (Kerney and Cameron, Reference Kerney and Cameron1979), and snails are more active at temperatures close to their growth optimum (Zaarur et al., Reference Zaarur, Olack and Affek2011; Yanes et al., Reference Yanes, Gutiérrez-Zugasti and Delgado2012). For example, the number of active Arianta arbustorum at 8°C is about five times higher than at 0°C and about two times higher than at 22°C (Cameron, Reference Cameron1970). Pupilla muscorum is active during the whole year, while the activity of Succinella oblonga is limited to the warm seasons of May to July (Pokryszko, Reference Pokryszko2001). In contrast, some pedogenic carbonates (e.g., rhizoliths) may form over a few decades (Gocke et al., Reference Gocke, Pustovoytov, Kühn, Wiesenberg, Löscher and Kuzyakov2011). Pedogenic carbonate nodule and pendant formation takes place over hundreds to thousands of years (Gile et al., Reference Gile, Peterson and Grossman1966; Birkeland, Reference Birkeland1999) and occurs seasonally (Breecker et al., Reference Breecker, Sharp and McFadden2009), leading to clumped isotope temperatures that presumably reflect long-term averaged, and in many cases seasonally biased, environmental temperatures (Peters et al., Reference Peters, Huntington and Hoke2013; Huntington and Lechler, Reference Huntington and Lechler2015; Burgener et al., Reference Burgener, Huntington, Hoke, Schauer, Ringham, Latorre and Díaz2016; Gallagher and Sheldon, Reference Gallagher and Sheldon2016; Gallagher et al., Reference Gallagher, Hren and Sheldon2019; Huth et al., Reference Huth, Cerling, Marchetti, Bowling, Ellwein and Passey2019; Kelson et al., Reference Kelson, Huntington, Breecker, Burgener, Gallagher, Hoke and Petersen2020). The origin and time-integration of geogenic carbonate isotopic compositions may be more difficult to ascertain. Geogenic carbonates found in paleosol-loess sequences may record formation temperatures of minerals in the deflation area from which dust was sourced, where the minerals may have been formed through a variety of geological processes, including metamorphism (Catoni et al., Reference Catoni, Falsone and Bonifacio2012; Baez-Hernandez et al., Reference Baez-Hernandez, Garcia, Menendez, Jaramillo, Sanchez-Perez, Santana, Alonso, Mangas and Hernandez-Leon2019). Therefore, various carbonate types—even located in the same stratigraphic level in a paleosol-loess sequence—may record a mixture, but specific and potentially complementary environmental information based on differences in δ13C, δ18O, and Δ47 values.
We examine paleoenvironmental information recorded by carbonate types from a well-known paleosol-loess sequence, the Upper Pleistocene Nussloch paleosol-loess in the Rhine Valley of southwestern Germany (Zoller et al., Reference Zoller, Stremme and Wagner1988; Hatté et al., Reference Hatté, Antoine, Fontugne, Rousseau, Tisnérat-Laborde and Zöller1999; Rousseau et al., Reference Rousseau, Antoine, Hatté, Lang, Zöller, Fontugne and Othman2002, Reference Rousseau, Boers, Sima, Svensson, Bigler, Lagroix, Taylor and Antoine2017a, Reference Rousseau, Svensson, Bigler, Sima, Steffensen and Boersb; Antoine et al., Reference Antoine, Rousseau, Moine, Kunesch, Hatté, Lang, Tissoux and Zöller2009; Prud'homme et al., Reference Prud'homme, Lécuyer, Antoine, Hatté, Moine, Fourel, Amiot, Martineau and Rousseau2018). Taken together, the δ18O, δ13C, and Δ47 data for multiple carbonate types enable us to investigate the effects of geogenic carbonate input, pedogenesis, and environmental factors on resulting isotopic variations.
MATERIALS AND METHODS
Study area and sampling
The Nussloch paleosol-loess sequence (49°18.82′N, 8°43.01′E) in southwestern Germany (Fig. 1a) is a well-studied type section of the European paleosol-loess. The Upper Pleniglacial sequence in Nussloch, with a total thickness of 18.5 m, has been dated by 14C (Lang et al., Reference Lang, Hatté, Rousseau, Antoine, Fontugne, Zoller and Hambach2003; Rousseau et al., Reference Rousseau, Sima, Antoine, Hatté, Lang and Zoeller2007; Moine et al., Reference Moine, Antoine, Hatte, Landais, Mathieu, Prud'homme and Rousseau2017) and infrared-stimulated luminescence (Antoine et al., Reference Antoine, Rousseau, Zoller, Lang, Munaut, Hatté and Fontugne2001, Reference Antoine, Rousseau, Moine, Kunesch, Hatté, Lang, Tissoux and Zöller2009), indicating formation throughout the last glacial-interglacial cycle since ~130 ka (Zoller et al., Reference Zoller, Stremme and Wagner1988; Hatté et al., Reference Hatté, Fontugne, Rousseau, Antoine, Zoller, Tisnerat-Laborde and Bentaleb1998; Rousseau et al., Reference Rousseau, Sima, Antoine, Hatté, Lang and Zoeller2007, Reference Rousseau, Boers, Sima, Svensson, Bigler, Lagroix, Taylor and Antoine2017a). We sampled the younger part of the Nussloch sequence, which contains various morphologies of carbonates, including rhizoliths, loess dolls, land-snail shells, carbonate coatings, and hypocoatings, as well as concretions and earthworm biospheroliths (Moine et al., Reference Moine, Rousseau and Antoine2008, Reference Moine, Antoine, Hatte, Landais, Mathieu, Prud'homme and Rousseau2017; Gocke et al., Reference Gocke, Pustovoytov, Kühn, Wiesenberg, Löscher and Kuzyakov2011; Prud'homme et al., Reference Prud'homme, Lécuyer, Antoine, Moine, Hatté, Fourel, Martineau and Rousseau2016, Reference Prud'homme, Lécuyer, Antoine, Hatté, Moine, Fourel, Amiot, Martineau and Rousseau2018).

Figure 1. (color online) (a) The location of the Nussloch paleosol-loess sequence (49°18.82′N, 8°43.01′E) in southwestern Germany. (b) The sampling depths (adapted from Antoine et al., Reference Antoine, Rousseau, Moine, Kunesch, Hatté, Lang, Tissoux and Zöller2009) and (c–l) the types of carbonates collected from each depth. The black scale bar on all images is 1 cm, except (f) earthworm biospheroliths, which is 300 μm.
Sampling focused on the most abundant carbonate morphologies (i.e., rhizoliths, loess doll, land-snail shells, and earthworm biospheroliths) from three horizons (Fig. 1b). The sampled horizons are: (1) ca. 20 ka loess unit at 5–6 m below the modern surface (“Upper loess”), (2) the ca. 38 ka paleosol (Lohne soil) at ~12 m below the modern surface (“Paleosol”), and (3) the loess unit directly below the paleosol (“Lower loess”). The sampled layers correspond to units 32 and 31, 20 and 19 described by Antoine et al. (Reference Antoine, Rousseau, Moine, Kunesch, Hatté, Lang, Tissoux and Zöller2009), respectively. Bulk samples (~10 kg each) were collected in the field from ~50 cm deep into 1-m-wide outcrops that were freshly cleaned off by the authors. Bulk samples were taken to the lab and the various carbonate types were separated through wet sieving using a 50 μm screen. The following carbonate types were separated from each layer (Fig. 1c–l):
Upper loess: short and thin rhizoliths, < 1 mm in diameter and up to 1 cm long (“Fine-rhizo A;” Fig. 1c); short and thick rhizoliths, a few mm in diameter and length (“Fine-rhizo B;” Fig. 1d); large rhizoliths, up to 2 cm in diameter and 10 cm long (“Large-rhizo;” Fig. 1e); earthworm biospheroliths, average ~1 mm in diameter (Fig. 1f); loess dolls, a few mm in diameter and < 1 cm long with different shapes (e.g., elongated ovals or globules with rounded edges; Fig. 1g); a few small white shell pieces of unidentified taxa with a maximum length of 5 mm (“Up-shell;” Fig. 1h); and bulk loess (26% calcium carbonate equivalent; CCE).
Paleosol: Land-snail shells of Succinella oblonga (Fig. 1i), Pupilla muscorum (Fig. 1j), Trochulus hispidus (Fig. 1k), Arianta arbustorum (Fig. 1l), and bulk paleosol (21% CCE).
Lower loess: Land-snail shells of Succinella oblonga (Fig. 1i), Pupilla muscorum (Fig. 1j), Trochulus hispidus (Fig. 1k), Arianta arbustorum (Fig. 1l), and bulk loess (25% CCE). The land-snail shells observed and sampled in the Lower loess are the same species as those from the paleosol.
Stable isotope analysis and calculations
Carbonate samples were ground with a mortar and pestle into homogenized powders prior to stable isotope (δ18O, δ13C, Δ47) analyses at the University of Washington IsoLab. Sample preparation and analysis followed methods of Burgener et al. (Reference Burgener, Huntington, Hoke, Schauer, Ringham, Latorre and Díaz2016) and Schauer et al. (Reference Schauer, Kelson, Saenger and Huntington2016). Briefly, 8–20 mg of powdered carbonate was reacted in a common phosphoric acid bath held at 90°C. The released CO2 was purified cryogenically through an automated nickel and stainless steel vacuum line using an ethanol-dry ice slush trap and liquid N2 trap, then chromatographically with a helium carrier through a Porapak Q trap (50/80 mesh, 15 cm long, 4.5 mm ID, 0.635 mm OD, held at -20°C). Preparation was completed when the sample CO2 was frozen into a 6 mm Pyrex tube and flame sealed. The break seal containing purified CO2 was loaded on the autosampler of a Thermo MAT 253 dual inlet mass spectrometer to measure mass to charge ratio (m/z) 44–49. The intra-laboratory carbonate standards of C64, C2, and Coral (referenced to VPDB using NBS19 and LSVEC for δ13C, and NBS19 and NBS18 for δ18O), as well as interlaboratory calcite standards ETH1-4 (Meckler et al., Reference Meckler, Ziegler, Millán, Breitenbach and Bernasconi2014; Bernasconi et al., Reference Bernasconi, Müller, Bergmann, Breitenbach, Fernandez, Hodell, Jaggi, Meckler, Millan and Ziegler2018), were purified and analyzed following the same procedures as Nussloch samples. Two to eight replicates were analyzed per carbonate sample, based on available sample mass.
Δ47, δ13C, and δ18O values from mass spectrometer data were calculated based on Huntington et al. (Reference Huntington, Eiler, Affek, Guo, Bonifacie, Yeung, Thiagarajan, Passey, Tripati, Daëron and Came2009) using the pressure baseline correction of He et al. (Reference He, Olack and Colman2012), with data reduction and 17O correction methods and scripts from Schauer et al. (Reference Schauer, Kelson, Saenger and Huntington2016). Δ47 values were placed in the carbon dioxide equilibrium scale, or absolute reference frame (Dennis et al., Reference Dennis, Affek, Passey, Schrag and Eiler2011), using CO2 gases heated to 1000°C or equilibrated with water at 4°C and 60°C. Peirce outlier tests (Ross, Reference Ross2003) were applied to identify replicate outliers (4 of 75 Δ47 analyses [5%] identified as outliers based on either Δ47 or δ13C value), following which mean Δ47 and standard error (S.E.) of the mean values were calculated. Standard error of the mean Δ47 value is calculated as the standard deviation (taken to be the standard deviation of the sample replicates or the long-term standard deviation of carbonate standards, whichever is larger) divided by the square root of the number of replicates. T(Δ47) was calculated for mean Δ47 using the calibration of Petersen et al. (Reference Petersen, Defliese, Saenger, Daëron, Huntington, John and Kelson2019), which is based on data produced using the same or similar analytical methods and the same 17O correction as our samples. δ13C and δ18O values were placed on the VPDB scale using C64 and C2 for δ13C, and C64 and coral for δ18O.
To calculate the oxygen isotopic composition of the water from which the carbonate precipitated (δ18Owater), the equations in Kim and O'Neil (Reference Kim and O'Neil1997) were used to calculate the temperature-dependent fractionation factor α for calcite and aragonite (Eqs. 3, 4).


To estimate α for shell carbonates comprising both aragonite and calcite (shells contained 62–100% aragonite; determined by the authors), we did a mass balance of the two relationships. The δ18Owater was then calculated based on Eq. 5:

RESULTS
Isotopic results for Upper loess, Paleosol, and Lower loess samples are summarized in Table 1, and full data for samples and standards is provided in Supplementary Table A1.
Table 1. Stable isotopes and clumped isotope data for carbonate types collected from three depths at Nussloch paleosol-loess sequence.

* Outliers are notedin italics
δ13C and δ18O of carbonate types
Carbonate δ13C values ranged from -11 to -0.5‰ and δ18O values from -6.1‰ to -2.2‰ (VPDB), with the average uncertainties of individual measurements of 0.04‰ and 0.03‰ for δ13C and δ18O, respectively.
All rhizoliths, loess dolls, and bulk loess samples in the Upper loess have similar carbonate δ18O values ranging from -6.1 to -5.6‰ (VPDB). δ18O values of shell samples and earthworm biospheroliths from this horizon are significantly higher at -2.3 and -4.7‰, respectively (VPDB) (Table 1, Fig. 2). While all Upper loess rhizoliths have similar δ18O values (-5.9 ± 0.1‰ VPDB, ± 1σ, n = 22), δ13C values depend on rhizolith size. Small rhizoliths (Fine-rhizo A and B) have average δ13C values (-6.1 ± 0.4‰) that are 2‰ higher than Large-rhizo samples from this interval (-7.0‰ and -8.2‰). δ13C values for the shell sample (-6.6‰) and pedogenic loess dolls (~-7.0‰) from the Upper loess fall within the range of rhizoliths from this horizon. Earthworm biospheroliths from the Upper loess are characterized by the lowest δ13C for the interval at -11.5 ‰. Measured δ13C for bulk loess in the Upper loess interval (-0.5‰) is significantly higher than all other carbonates from the same horizon, a relationship consistently observed in the other sampled horizons.

Figure 2. (color online) Isotopic composition of carbon (δ13C) and oxygen (δ18O) in various carbonate types present in the three sampled horizons in the Nussloch paleosol-loess sequence. The δ13C and δ18O in bulk loess carbonates is indicated by the grey-dashed circle. Note the shift (-1‰) in δ13C values of land-snail S. oblonga, P. muscorum, and T. hispidus shells from Lower loess to Paleosol. A similar magnitude δ13C shift is observed between δ13C values of bulk carbonates from Lower loess to Paleosol. Where errors are larger than the size of the symbols, standard errors are shown with horizontal and vertical bars, respectively, for δ13C and δ18O.
The Lower loess and Paleosol samples show similar shifts in δ13C and δ18O values by carbonate type, with the shells having significantly lower δ13C and higher δ18O values than the bulk loess or bulk paleosol. The three sampled shell species S. oblonga, P. muscorum, and T. hispidus in the Lower loess and the Paleosol have a narrow and similar range of δ18O values (-3.8 ± 0.2‰), which is more than 1‰ lower than values for A. arbustorum (-2.3 ± 0.1‰). The δ13C values of S. oblonga, P. muscorum, and T. hispidus sampled in both horizons (-3.1‰ to -6.1‰) are systematically offset relative to δ13C values of the bulk paleosol (-1.9‰) and bulk loess from the Lower loess interval (-0.9‰) (Fig. 2). δ13C values for A. arbustorum are similar across the Paleosol and Lower loess horizons (-8‰). A similar magnitude δ13C offset is observed for bulk loess/paleosol carbonate samples from the Lower loess and Paleosol horizons (Fig. 2).
Formation temperatures [T(Δ47)] of carbonate types
Measured carbonate Δ47 values range from 0.754–0.499‰, corresponding to apparent temperatures of 8–120°C (Table 1). The T(Δ47) values of the bulk carbonate samples range from ~78–120°C, far exceeding Earth-surface temperature conditions, while T(Δ47) values for other carbonate types (i.e., pedogenic and biogenic) range from ~8–38°C and are in the plausible range of Earth-surface temperatures (Fig. 3). The small rhizoliths (Fine-rhizo A and B) in the Upper loess (Fig. 3) yielded temperatures of 24 ± 4°C and 30 ± 2°C, respectively, one of which is indistinguishable from loess doll T(Δ47) (23 ± 3°C). The small rhizolith and loess doll temperatures are distinguishable from the temperatures of two Large-rhizo samples (12 ± 4 and 15 ± 5°C), Up-shell (12 ± 6°C), and earthworm biospherolith (8 ± 3°C) samples. Land-snail shells revealed two distinct temperature ranges in both the Paleosol and the Lower loess horizons (Fig. 3). The clumped isotope temperatures for S. oblonga (38 ± 4 and 36 ± 6°C) and T. hispidus (27 ± 5 and 32 ± 5°C) are consistently warmer than analyzed P. muscorum (16 ± 6 and 21 ± 4°C) and A. arbustorum (12 ± 4 and 15 ± 3°C) samples. Similar land-snail species show similar temperatures in the Paleosol and in the Lower loess (Fig. 3).

Figure 3. (color online) Temperature of carbonate formation calculated based on T(Δ47) values of carbonate types present at three depths in the Nussloch paleosol-loess sequence. The inset graph shows the temperatures of carbonate types in the Upper loess horizon. Horizontal bars show standard errors.
The calculated value of reconstructed δ18Owater
Calculated apparent δ18Owater values vary among carbonate types (Fig. 4). Apparent δ18Owater for bulk loess and paleosol carbonates are positive values (6–12‰ VSMOW). In contrast, the other carbonate types (i.e. pedogenic and biogenic carbonates) have δ18Owater values in the range of possible paleoenvironmental water values. Reconstructed apparent δ18Owater values for rhizoliths of different sizes (-6.4 to -2.1‰), land-snail shells of different species (-3.8 to +0.3‰) and earthworm biospheroliths (-6.0‰) differ significantly, even at a similar depth. However, similar species of land-snails record similar reconstructed δ18Owater values independent of depth (Fig. 4).

Figure 4. (color online) The estimated isotopic composition of water (δ18Owater), from which the carbonate types were formed. The error bars are smaller than the symbol size for all carbonate types. The solid vertical line at δ18Owater = 0 is shown for reference.
DISCUSSION
Stable isotopic compositions vary with carbonate type within a single sedimentological layer within the Nussloch loess-paleosol sequence. T(Δ47) in excess of 78°C indicates bulk loess and bulk paleosol carbonates are not suitable for paleoenvironmental reconstructions. In contrast, the pedogenic and biogenic carbonate types record distinct paleoenvironmental information, with sample δ18O, δ13C, and Δ47 values likely reflecting specific formation mechanisms and time periods over which the carbonates precipitated.
Geogenic carbonates: bulk loess and bulk paleosol samples
δ13C, δ18O, and T(Δ47) values suggest carbonates in bulk loess and bulk paleosols of the Nussloch sequence are detrital rather than pedogenic in origin. Apparent T(Δ47) values of bulk carbonates (~78–120°C) far exceed the range of plausible Earth-surface temperatures (Fig. 3). Additionally, calculated apparent δ18Owater values of bulk carbonates (6.3–12‰; Fig. 4) are inconsistent with formation in situ in the (paleo)soil unit. Instead, the isotopic data point to diagenetic and/or metamorphic sources for these carbonates, which we suggest were likely derived from pre-existing rock through processes of weathering and erosion.
Waters in metamorphic environments are commonly characterized by high δ18O values (potentially > 0‰ VSMOW) as a result of dehydration processes and water-rock isotopic exchange in deep-crustal environments (Sheppard, Reference Sheppard1986), which may explain the positive δ18Owater values calculated for many bulk carbonates in the Nussloch sequence. Diagenetic and metamorphic carbonates are exposed throughout Western Europe, making it difficult to ascertain the exact source(s) of Nussloch detrital carbonate. That said, prevailing westerly and north-westerly winds into the Nussloch region during the last glacial period (Antoine et al., Reference Antoine, Rousseau, Degeai, Moine, Lagroix, Kreutzer, Fuchs, Hatte, Gauthier, Svoboda and Lisa2013; Rousseau et al., Reference Rousseau, Chauvel, Sima, Hatte, Lagroix, Antoine, Balkanski, Fuchs, Mellett, Kageyama, Ramstein and Lang2014) point to source regions to the west of the Nussloch Basin, possibly marbles exhumed in the Scottish Highlands and abundant limestone throughout the Devon Basin in England. Additional geochemical data (e.g., Sr-Nd isotopes) could potentially help decipher source(s) for Nussloch detrital carbonate. Nevertheless, high apparent T(Δ47) and δ18Owater values of bulk carbonates indicate that bulk carbonates do not record the local environmental conditions during or following sedimentation and cannot be used as a direct paleoenvironmental proxy.
Pedogenic carbonates: rhizoliths and loess dolls
Rhizoliths and loess dolls are pedogenic carbonates formed in situ in the soil (e.g., Gocke et al., Reference Gocke, Wiesenberg, Pustovoytov and Kuzyakov2010, Reference Gocke, Pustovoytov, Kühn, Wiesenberg, Löscher and Kuzyakov2011; Li et al., Reference Li, Wang, Cheng, Ning, Zhao, Li, Marković, Catto, O'Hara-Dhand and McLaren2015; Gao et al., Reference Gao, Li, Zhu and Liao2020; Sun et al., Reference Sun, Zamanian, Huguet, Fa and Wang2020). Their δ13C values should largely reflect vegetation type, their δ18Owater mirror the values of local soil water, and T(Δ47) is presumably a measure of the soil temperature during soil drying and CO2 degassing. The pedogenic carbonate isotopic values are generally consistent with formation in soil conditions. In the following paragraphs, we examine the pedogenic carbonate data in the context of the range of δ13C, δ18O, and T(Δ47) values expected for Nussloch site paleoenvironmental conditions and discuss implications of distinct seasonality and mechanisms of pedogenic carbonate formation for paleoenvironmental reconstruction.
Multiple lines of evidence place constraints on Nussloch paleoenvironmental conditions relevant to the period of pedogenic carbonate formation. We lack direct constraints on local terrestrial δ18Owater values during this period; however, Sima et al. (Reference Sima, Rousseau, Kageyama, Ramstein, Schulz, Balkanski, Antoine, Dulac and Hatté2009) assumed that precipitation sources and dominant wind trajectories were similar to modern during the Last Glacial. Such δ18Owater values of precipitation would differ by only ~0.5‰ from modern (-11‰ to -7‰) (Field, Reference Field2010; Terzer et al., Reference Terzer, Wassenaar, Araguás-Araguás and Aggarwal2013) due to the ice volume effect (Prud'homme et al., Reference Prud'homme, Lécuyer, Antoine, Moine, Hatté, Fourel, Martineau and Rousseau2016). Today, minimum and maximum monthly mean air temperatures in the study area range from ~0 to ~25°C, and average cold- and warm-season air temperatures are 1.7 ± 0.9°C (DJF) and 18 ± 2°C (JJA), respectively. The mean annual air temperature is 10°C, and modern mean annual soil temperature is therefore likely to be in the range of 10°C to up to 3–5°C higher due to radiative heating of the soil surface (Roxy et al., Reference Roxy, Sumithranand and Renuka2014). Significant elevation of soil surface temperatures via radiative heating is common when the annual precipitation is < 600 mm (Burgener et al., Reference Burgener, Hyland, Huntington, Kelson and Sewall2019; Gallagher et al., Reference Gallagher, Hren and Sheldon2019), as it is estimated to have been in Nussloch (Prud'homme et al., Reference Prud'homme, Lécuyer, Antoine, Hatté, Moine, Fourel, Amiot, Martineau and Rousseau2018). Recent air and soil temperature estimates from earthworm calcite granules at Nussloch suggest similar-to-modern mean warm season air and soil temperatures of 12 ± 4 and 15 ± 5°C, respectively, during paleosol formation (Prud'homme et al., Reference Prud'homme, Lécuyer, Antoine, Moine, Hatté, Fourel, Martineau and Rousseau2016). These temperature estimates were based on measured carbonate δ18O and assumed δ18Owater values for the Nussloch paleosol-loess sequence. Other paleo-temperature estimates suggest comparable-to-modern mean annual air temperatures of 8.5–10°C during paleosol formation in analogous paleosol-loess sequences in Hungary (Schatz et al., Reference Schatz, Scholten and Kühn2015).
Within these ranges of temperature, the season over which the rhizoliths are formed and the formation mechanisms of rhizoliths should determine the isotopic values and temperatures recorded. Nussloch rhizolith samples have δ13C values that are dependent on rhizolith size: small rhizoliths have higher δ13C values than large rhizoliths and loess dolls from the same horizon (Fig. 2). We interpret the dependence of the δ13C values with rhizolith size to suggest that these carbonates originate from different plant species or groups, and/or form through specific mechanisms (e.g., root water uptake or soil wetting-drying cycles), motivating hypotheses that can be tested using the suite of δ13C, δ18O and T(Δ47) data.
First, it is possible that rhizolith formation mechanisms vary with time. Water uptake by roots and resulting water flow toward the root is accepted as the main formation mechanism for rhizoliths (Klappa, Reference Klappa1980; Lambers et al., Reference Lambers, Mougel, Jaillard and Hinsinger2009; Gocke et al., Reference Gocke, Pustovoytov, Kühn, Wiesenberg, Löscher and Kuzyakov2011). Following root death, water uptake by the root and water flow towards the root is stopped. However, non-biogenic mechanisms (e.g., wetting-drying cycles) can still drive further carbonate dissolution and precipitation. In this way, a rhizolith formed in association with water uptake by roots may subsequently act as a framework for further carbonate precipitation to build on such that increased size of the rhizolith records a longer formation period (Sun et al., Reference Sun, Xue, Zamanian, Colin, Duchamp-Alphonse and Pei2019b, Reference Sun, Zamanian, Li, Wang, Colin and Sunc; Huguet et al., Reference Huguet, Bernard, Khatib, Gocke, Wiesenberg and Derenne2020). In this scenario, specific formation mechanisms operating over different time scales would affect the environmental information recorded by rhizoliths larger than a few millimeters compared to smaller rhizoliths that presumably form over a short period of time associated with active root water uptake.
Vegetation type could also cause differences in the isotopic signals recorded by large and small rhizoliths. Indeed, a variety of vegetation species are associated with rhizolith formation (Rodriguez-Aranda and Calvo, Reference Rodriguez-Aranda and Calvo1998; Alonso-Zarza, Reference Alonso-Zarza1999; Matteucci et al., Reference Matteucci, Belluomini and Manfra2007). If large rhizoliths form along perennial roots that are bigger than the roots of annual grasses, this would lead to continued root-water uptake over many years. In contrast, the morphology of small rhizoliths suggest that they formed along the smaller roots of annual grasses, likely over one growing season. Such a difference in duration of carbonate formation could explain the observed differences in δ13C, δ18O, and T(Δ47) values among large and small rhizoliths. Alternatively, small rhizoliths might have formed when the plant was under water stress, for example at the end of the winter season through the early spring (Tranquillini, Reference Tranquillini, Lange, Nobel, Osmond and Ziegler1982), which is most probably the case in glacial times (Yung et al., Reference Yung, Lee, Wang and Shieh1996; Medeiros and Ward, Reference Medeiros and Ward2013). Under such conditions the plant will close the stomata to reduce water loss, which leads to 13C enrichment by C3 photosynthesis (e.g., Dawson et al., Reference Dawson, Mambelli, Plamboeck, Templer and Tu2002). Observed differences in temperature and δ18Owater values among small versus large rhizoliths could be consistent with small-rhizolith formation under water stress, with fine rhizoliths recording higher formation temperature (Fig. 3) and forming from more evaporative waters (Fig. 4). The δ13C values (Fig. 2) do not show obvious evaporation control, potentially reflecting other factors, such as plant type, that may influence δ13C values.
We suggest that rhizolith formation temperatures (Fig. 3) are consistent with differences in formation duration and mechanisms. We suggest small rhizoliths likely formed over short periods, potentially as short-lived as during a single soil-drying event due to root-water uptake. In contrast, large rhizoliths may have formed over a few years up to a few decades (Gocke et al., Reference Gocke, Pustovoytov, Kühn, Wiesenberg, Löscher and Kuzyakov2011; Sun et al., Reference Sun, Wang and Zamanian2019a) integrating several warm seasons, and therefore recording the mean temperature of those seasons. We therefore interpret the clumped isotope temperature for the large rhizoliths (average T[Δ47] = 14 ± 5°C) to represent warm-season soil conditions averaged over up to a few decades.
The clumped isotope warm-season soil temperature reconstruction is consistent with the average mean warm-season soil temperature estimates (13 ± 4°C and 15 ± 3°C) reported for Nussloch (Prud'homme et al., Reference Prud'homme, Lécuyer, Antoine, Moine, Hatté, Fourel, Martineau and Rousseau2016, Reference Prud'homme, Lécuyer, Antoine, Hatté, Moine, Fourel, Amiot, Martineau and Rousseau2018). Prud'homme et al. (Reference Prud'homme, Lécuyer, Antoine, Moine, Hatté, Fourel, Martineau and Rousseau2016) estimated δ18Owater values during last glacial at Nussloch based on a linear equation among modern values of water δ18O (δ18Omwater) and modern mean annual air temperature of the IAEA dataset, and used the δ18Omwater values to calculate temperature from carbonate δ18O. Assuming measured T(Δ47) values record soil carbonate formation temperatures, we can directly calculate the δ18Owater values during rhizolith formation. The good agreement between temperature results from our study and those of Prud'homme et al. (Reference Prud'homme, Lécuyer, Antoine, Moine, Hatté, Fourel, Martineau and Rousseau2016) suggest that the assumptions by Prud'homme et al. (Reference Prud'homme, Lécuyer, Antoine, Moine, Hatté, Fourel, Martineau and Rousseau2016) about paleo δ18Owater values at Nussloch during the last glacial interval were reasonable.
Loess doll formation may occur over centuries or longer depending on size (Zamanian et al., Reference Zamanian, Pustovoytov and Kuzyakov2016a), rather than during a single vegetation period like small rhizoliths. However, the loess dolls and one of the small rhizolith samples are similar in both formation temperatures and size (Figs. 1, 3). Thus, it is possible that the small loess dolls might also be formed over short periods, although more evidence than just formation temperature would be necessary to substantiate such an argument.
Taken together with the δ13C and δ18O data, T(Δ47) values further support interpretation of differences in duration of carbonate growth by carbonate type (Fig. 5). We suggest that small rhizoliths (a few millimeters in diameter) and loess dolls exhibiting large variability in T(Δ47) (19–32°C) record the temperature of one growing season and/or soil-drying event. Such transient growth during peak warm-season events can explain why observed T(Δ47) values are significantly warmer than expected for growth during glacial conditions. In contrast, we interpret average T(Δ47) of ~14°C for large rhizoliths (a few centimeters in diameter) as a more reliable measure of average paleo-temperatures across multiple growing seasons, or over decades (Table 1, Fig. 3).
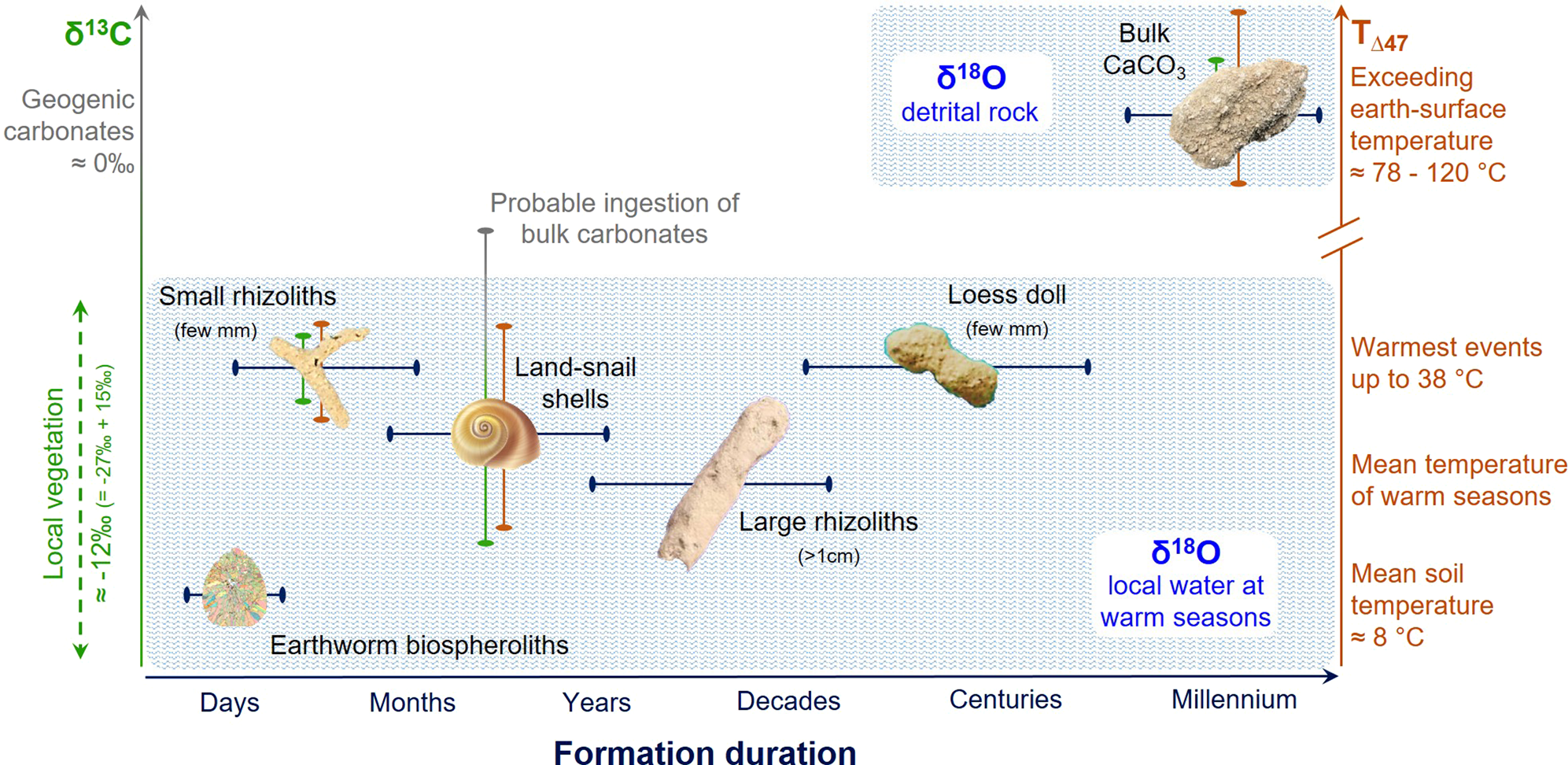
Figure 5. (color online) Dependency and resolution of paleoenvironmental interpretations based on carbonate types on formation duration and formation mechanisms. While δ13C and T(Δ47) are plotted on the left and right y-axes, respectively, δ18O is lumped into the two pools and shown as the two blue shaded regions. While bulk carbonates show more positive δ13C values of geogenic origins (~0‰), pedogenic and biogenic carbonates record δ13C values of local vegetation. Note that the δ13C values of local vegetation (~ -27‰ for C3 species) are enriched by about 15‰ during carbonate precipitation process (e.g., -12‰ for earthworm biospheroliths). Some error bars are smaller than the symbols.
Biogenic carbonates: land-snail shells and earthworm biospheroliths
The δ13C and δ18O values of distinct land-snail species (Fig. 2) reflect the specifics in the diet of these organisms (i.e., ingested plants and, to a lesser extent, geogenic carbonate). δ13C values in shell carbonate depend mainly on the land-snail diet regime (Metref et al., Reference Metref, Rousseau, Bentaleb, Labonne and Vianey-Liaud2003; Balakrishnan et al., Reference Balakrishnan, Yapp, Theler, Carter and Wyckoff2005; Zhang et al., Reference Zhang, Yamada, Suzuki and Yoshida2014). The similar shift (~ -1‰) to lower δ13C values from Lower loess to paleosol in both land-snail shells and bulk carbonates reveals that the land snails S. oblonga, P. muscorum, and T. hispidus were ingesting bulk carbonate (Pigati et al., Reference Pigati, Rech and Nekola2010; Yanes et al., Reference Yanes, Gutiérrez-Zugasti and Delgado2012) (Fig. 2). These identified species are all able to ingest carbonates (Fechter et al., Reference Fechter, Falkner, Steinbach and Wendler1990; Boschi et al., Reference Boschi, Kappeler and Tanner2011), which comprise ~5–25% of their diet (Pigati et al., Reference Pigati, Rech and Nekola2010; Yanes et al., Reference Yanes, Gutiérrez-Zugasti and Delgado2012). We can estimate the δ13C of vegetation during paleosol formation and Lower loess deposition using the equation of Yanes et al. (Reference Yanes, Delgado, Castillo, Alonso, Ibáñez, De la Nuez and Kowalewski2008) based on shell carbonate δ13C values. Using this equation, and assuming 0% carbonate ingestion, the range of δ13C of vegetation is calculated as -22 to -17‰ VPDB; but considering 5–25% carbonate ingestion, the estimated δ13C values of vegetation range from -25‰ to -20‰ VPDB. The latter calculated δ13C range of vegetation is consistent with the reported δ13C values of organic carbon (-24.9 to -23.7‰) for the Nussloch paleosol-loess sequence (Hatté et al., Reference Hatté, Antoine, Fontugne, Rousseau, Tisnérat-Laborde and Zöller1999; Prud'homme et al., Reference Prud'homme, Lécuyer, Antoine, Hatté, Moine, Fourel, Amiot, Martineau and Rousseau2018), supporting our interpretation of significant carbonate ingestion by analyzed snail species. Similar calculations using A. arbustorum, which we assume did not ingest carbonate due to the similarity of δ13C values for co-occurring shell and non-biogenic carbonate in paleosol and Lower loess, also gives a δ13C value of -26‰ for vegetation. Lastly, earthworm biospheroliths also can be used to reconstruct plant δ13C (Prud-hommme et al., Reference Prud'homme, Lécuyer, Antoine, Hatté, Moine, Fourel, Amiot, Martineau and Rousseau2018). Using an epsilon calculated from Canti (Reference Canti2009) and typical diet estimation equations (Passey et al., Reference Passey, Robinson, Ayliffe, Cerling, Sponheimer, Dearing, Roeder and Ehleringer2005), we calculate a δ13Cplant of -23‰ from the earthworm biospheroliths δ13C values.
The clumped isotope data and calculated δ18Owater values are broadly consistent with the hypothesis that land-snail individuals record seasonal and local changes in atmospheric temperature (Eagle et al., Reference Eagle, Risi, Mitchell, Eiler, Seibt, Neelin, Li and Tripati2013; Wang et al., Reference Wang, Cui, Zhai and Ding2016; Zhang et al., Reference Zhang, Yamada, Kano, Matsumoto and Yoshida2018; Zhai et al., Reference Zhai, Wang, Qin, Cui, Zhang and Ding2019) and the isotopic composition of local precipitation modified by evaporation (Zaarur et al., Reference Zaarur, Olack and Affek2011; Dong et al., Reference Dong, Eiler, An, Wu, Liu, Li, Kitchen and Lu2020). Different species grow in seasons with different temperatures (Wang et al., Reference Wang, Cui, Zhai and Ding2016; Zhai et al., Reference Zhai, Wang, Qin, Cui, Zhang and Ding2019), precipitation δ18Owater values, and relative humidity. Furthermore, because individual snails are short lived, considerable variation in the temperatures and δ18Owater values recorded by land-snail individuals is expected (Eagle et al., Reference Eagle, Risi, Mitchell, Eiler, Seibt, Neelin, Li and Tripati2013). This variability is further exacerbated because the recorded clumped isotope temperatures depend on shell properties such as shell color and morphology, and the ecological behavior of the snail (Zaarur et al., Reference Zaarur, Olack and Affek2011). Accordingly, the observed variability among individual snail-shell T(Δ47) and reconstructed δ18Owater values within a single horizon is expected; however, this natural variability can mask and complicate interpretation of environmental shifts in the proxy records.
Land-snail shells, following embedding in loess and paleosol, might also have undergone recrystallization and associated re-equilibration of shell carbonate isotopic values due to dissolution and re-precipitation (Zamanian et al., Reference Zamanian, Pustovoytov and Kuzyakov2016b, Reference Zamanian, Pustovoytov and Kuzyakovc). Hence, it is possible that recrystallization might have biased the recorded temperatures toward warmer, post-burial environmental conditions. However, we are unable to determine the extent of such effects. Large T(Δ47) uncertainties for some analyzed biogenic carbonate samples (Table 1) further limit our ability to test the hypothesis of land-snail variability rigorously. Future studies, where it is possible to sample a large number of individual shells of each species, could achieve sufficient precision (Eagle et al., Reference Eagle, Risi, Mitchell, Eiler, Seibt, Neelin, Li and Tripati2013).
In contrast to land-snail shells, earthworms seem to not incorporate the ingested carbonates in their biospheroliths (Moine et al., Reference Moine, Antoine, Hatte, Landais, Mathieu, Prud'homme and Rousseau2017). Thus, the T(Δ47) values (8 ± 2°C) of earthworm biospheroliths likely record the temperature at the soil surface during earthworm activity. Our direct biospheroliths T(Δ47) measurement is within the range, but on the lower side of our average warm-season soil temperature estimate based on large rhizolith clumped isotope measurements (14 ± 5°C), and the warm-season soil (13 ± 4°C to 15 ± 3°C) temperature ranges inferred by Prud´homme et al. (Reference Prud'homme, Lécuyer, Antoine, Moine, Hatté, Fourel, Martineau and Rousseau2016) for the Nussloch paleosol-loess sequence. The uncertainties are large enough to permit significant variation in biospherolith-based temperature estimates, which would not be surprising given that the carbonate samples here and in the study of Prud´homme et al. (Reference Prud'homme, Lécuyer, Antoine, Moine, Hatté, Fourel, Martineau and Rousseau2016) homogenize a large number of individual earthworm granules, each of which formed in a single day. Soil carbonate formation and earthworm activity were likely both restricted to the warmest periods of year in the Nussloch permafrost environment, so we do not suggest that the low resolution of these thermometry techniques masks a seasonal difference in rhizoliths and biospheroliths formation.
Implications for paleoenvironmental reconstructions from stable isotopes of carbonates in loess and paleosol deposits
Our observations of the δ13C, δ18O, and clumped isotope temperatures of carbonate types associated with the Nussloch paleosol-loess sequence suggest several broader implications for paleoenvironmental reconstructions from these and similar deposits (Table 2, Fig. 5).
Table 2. The suitability of δ13C, δ18O, and T(Δ47) values of various carbonate types at Nussloch paleosol-loess sequence for local paleoenvironmental reconstructions.

First, the contribution of detrital carbonate to bulk loess and paleosol carbonate makes these materials unsuitable for paleoenvironmental reconstruction (e.g., interpreting mean annual or seasonal air temperatures), as observed in other recent T(Δ47) investigations of loess-paleosol sequences (Lechler et al., Reference Lechler, Huntington, Breecker, Sweeney and Schauer2018). The contribution of geogenic detrital carbonate was made obvious by the clumped isotope apparent temperatures well in excess of plausible Earth surface conditions. However, it is possible that in the absence of clumped isotope data, the contribution of bulk geogenic carbonate may not be recognized. Geogenic carbonate contribution may be particularly difficult to recognize in deposits where pedogenic grain coatings of carbonate formed in situ also contribute significantly to the isotopic composition of the bulk carbonate samples. In such cases, it may be possible to interpret variations in carbonate δ13C and δ18O to document temporal variation in the seasonality of precipitation, in vegetation or in other paleoenvironmental variables, when in reality the carbonate δ13C and δ18O variations may document variations in the degree of pedogenic overprinting of detrital carbonate in bulk loess or paleosol deposits. Clumped isotope data would provide a simple test of the interpretation. In addition, isotopic analysis of bulk carbonate in paleosol-loess deposits can be valuable in interpreting isotopic compositions of land-snail shells, given that many of these organisms ingest this carbonate as a significant proportion of their diet.
Second, our findings contribute to a growing body of literature concerning seasonal bias in pedogenic carbonate formation, and the implications of this bias for paleoenvironmental reconstruction (e.g., Burgener et al., Reference Burgener, Huntington, Hoke, Schauer, Ringham, Latorre and Díaz2016; Kelson et al., Reference Kelson, Huntington, Breecker, Burgener, Gallagher, Hoke and Petersen2020). Most previous work on this topic has focused on carbonate pendant (clast coating) and nodule formation in modern and Holocene soils (Breecker et al., Reference Breecker, Sharp and McFadden2009; Quade et al., Reference Quade, Breecker, Daeron and Eiler2011; Peters et al., Reference Peters, Huntington and Hoke2013; Burgener et al., Reference Burgener, Huntington, Hoke, Schauer, Ringham, Latorre and Díaz2016; Gallagher and Sheldon, Reference Gallagher and Sheldon2016; Ringham et al., Reference Ringham, Hoke, Huntington and Aranibar2016). We complement these approaches by examining isotopic values in carbonates of different types in the same temporal horizon. The variations in carbonate δ13C, δ18O (Fig. 2), and clumped isotope temperatures (Fig. 3) are consistent with specific mechanisms of carbonate formation that integrate environmental information over time periods, which we suggest may range from a single vegetation growth event in small rhizoliths, to decades and centuries of summers in larger rhizoliths. Together, our interpretations provide guidance for sampling strategies, to consider time resolution in future studies. Such studies may target carbonates that grow over short periods to examine seasonal variability and extremes, and carbonates that grow over longer periods to document seasonal average temperatures and representative vegetation and precipitation isotopic values (Burgener et al., Reference Burgener, Huntington, Hoke, Schauer, Ringham, Latorre and Díaz2016; Ringham et al., Reference Ringham, Hoke, Huntington and Aranibar2016; Ji et al., Reference Ji, Nie, Lechler, Huntington, Heitmann and Breecker2018; Lechler et al., Reference Lechler, Huntington, Breecker, Sweeney and Schauer2018).
Finally, our results and those of other studies in the Nussloch remind us that pedogenic/biogenic processes as well as seasonal biases must be considered in paleoenvironmental interpretations of paleosol archives. In European paleosol-loess archives alone, reconstructions of Last Glacial paleo-temperatures depend significantly on both the proxy method and the type of deposit to which the method is applied. Specific proxies may provide estimates for a particular month or average warm season air temperature (Rousseau, Reference Rousseau1991; Moine et al., Reference Moine, Rousseau, Jolly and Vianey-Liaud2002), for mean annual air temperature (Renssen and Vandenberghe, Reference Renssen and Vandenberghe2003; Vandenberghe et al., Reference Vandenberghe, French, Gorbunov, Marchenko, Velichko, Jin, Cui, Zhang and Wan2014; Schatz et al., Reference Schatz, Scholten and Kühn2015), for winter air temperature (Rousseau, Reference Rousseau1991), or for seasonally biased or mean annual soil temperature (Prud'homme et al., Reference Prud'homme, Lécuyer, Antoine, Moine, Hatté, Fourel, Martineau and Rousseau2016). Mean annual temperature estimates for periods of loess formation and paleosol formation also differ from one another (Schatz et al., Reference Schatz, Scholten and Kühn2015), with paleosol formation representing milder climate conditions during interstadial events (Prud'homme et al., Reference Prud'homme, Lécuyer, Antoine, Moine, Hatté, Fourel, Martineau and Rousseau2016). Better understanding of the processes responsible for differences in proxy records as a function of the periods over which proxy materials form is needed to integrate multiple records into more complete paleoenvironmental reconstructions.
CONCLUSIONS
Various carbonate types deposited in the same paleo-horizon or layer of the Nussloch paleosol-loess sequence record specific environmental information because of differences in formation mechanisms and duration. Bulk carbonates in the Nussloch paleosol-loess sequence are detrital materials that record the diagenetic conditions of carbonates in the deflation area instead of local environmental conditions. Therefore, bulk carbonates should be excluded from samples taken to reconstruct the local paleoenvironment. We interpret small rhizoliths, loess dolls, land-snail shells, and earthworm biospheroliths as records of temperatures and soil water content that reflect environmental conditions over the short time periods over which they form. In contrast, large rhizoliths (centimeters in thickness) record mainly summer temperatures averaged over decades. This finding suggests that rhizolith size should be considered for paleoenvironmental reconstructions, with larger rhizoliths being more reliable indicators of average warm season conditions. Earthworm biospheroliths and land-snail shells are suitable materials for paleoenvironment and paleovegetation reconstructions. In the case of land snails, their ecological habit, including the proportion of ingested bulk/geogenic carbonate and the isotopic composition of bulk soil carbonates should be considered. This study highlights that with proper consideration of proxy carbonate type and associated formation mechanisms, integrated stable (δ13C, δ18O) and clumped (Δ47) isotopic values of pedogenic and biogenic carbonates can yield robust paleoenvironment reconstructions with decadal and even seasonal temporal resolution.
ACKNOWLEDGMENTS
Our sincere thanks go to Manfred Löscher for kind discussion in the field and recognizing the sampled sediment layers. Special thanks to Landon Burgener for X-ray analyses of land-snail shells and Casey Saenger for suggestions regarding calculation of δ18Owater from shell carbonate δ18O. We highly appreciate comments and suggestions of associate editor Jeff Pigati and reviewers Denis-Didier Rousseau and Christophe Lécuyer. The study was supported by funding from the German Academic Exchange Service (DAAD) (PPP with USA, application contract: 57211165), German Research Foundation (DFG) (KU 1184/34-1), U.S. National Science Foundation (EAR-1156134 to KWH), Quaternary Research Center (QRC) of the University of Washington, the Government Program of Competitive Growth of Kazan Federal University, and with the support of the RUDN University program 5-100.
SUPPLEMENTARY MATERIAL
The supplementary material for this article can be found at https://doi.org/10.1017/qua.2020.109