1.1 Anatomical Planes and Orientation of the Brain
The neuroaxis of humans and other bipedal orthograde animals is different from that of quadruped animals. Example axes include the anteroposterior axis, rostrocaudal axis and the dorsoventral axis (Figure 1.1). The brain is usually visualized in sections cut through three orthogonal planes: sagittal (longitudinal), coronal, and transverse (axial) planes.
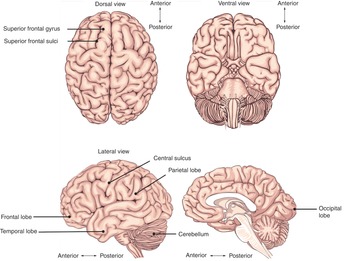
Figure 1.1 Planes and orientations of the brain.

Figure 1.2 Planes and orientations of the body.
1.2 Vascular Supply to the Brain
The brain requires 15–20% of the resting cardiac output and is exquisitely sensitive to oxygen deprivation. Two main pairs of arteries supply blood to the brain: the internal carotid arteries and the vertebral arteries. Within the cranial vault, an anastomotic circle, called the Circle of Willis (Figure 1.3), forms from the terminal branches of these arteries.

Figure 1.3 Circle of Willis.
The brachiocephalic trunk arises from the aorta and bifurcates into the right common carotid and right subclavian artery. The left common carotid artery and subclavian artery branches off directly from the aortic arch. The common carotid arteries divide at the level of the thyroid cartilage (C4) into external and internal carotid arteries. The carotid sinus, a dilation at the base of the internal carotid artery, is a baroreceptor (stretch receptor) that detects changes in systemic blood pressure. Intimately related to it, is the carotid body, made up of glomus type I chemoreceptor cells and glomus type II supporting cells. The chemoreceptors are primarily sensors of partial pressure of oxygen (PaO2). The central chemoreceptors in the medulla oblongata are the primary sensors of the partial pressure of carbon dioxide (PaCO2) and pH, however the carotid bodies also play a secondary role.
The external carotid artery divides into the superficial temporal artery and the maxillary artery within the parotid gland and gives rise to six branches (superior thyroid artery, lingual artery, facial artery, ascending pharyngeal artery, occipital artery, and posterior auricular artery). The maxillary artery gives rise to the middle meningeal artery, which passes through the foramen spinosum to enter the cranial cavity.
The internal carotid artery enters the cranial cavity via the carotid canal in the petrous part of the temporal bone, passes through the cavernous sinus and penetrates the dura into the subarachnoid space. Distal to the cavernous sinus, it gives rise to the following branches: ophthalmic artery, posterior communicating artery, anterior choroidal artery, and anterior cerebral artery. It then continues as the middle cerebral artery. The anterior cerebral artery supplies the frontal lobes and medial aspects of the parietal and occipital lobes (Figure 1.4).

Figure 1.4 Arterial supply to the brain and venous drainage.
The middle cerebral artery is the largest cerebral artery and supplies the somatosensory and motor cortex, basal ganglia, and the cerebral white matter. It can be divided into four main surgical segments, denominated M1 (sphenoidal/horizontal), M2 (insular), M3 (opercular), and M4 (cortical) segments. M1 gives rise to the lenticulostriate perforating end arteries supplying the basal ganglia and internal capsule. Occlusion of these vessels can cause lacunar infarcts, the most common type of ischemic stroke. Hypertensive lipohyalinosis of these vessels can lead to the formation of Charcot-Bouchard aneurysms and these are a principal cause of intracerebral hemorrhage. The cortical branches of MCA arise from all of its segments and supply most of the lateral surface of the brain and include the anterior temporal arteries from M1, lateral frontobasal artery from M2, and parietal branches from M4. The vertebral arteries arise from the subclavian artery and pass though the foramen transversarium in the cervical vertebrae. The vertebral arteries enter the cranium via the foramen magnum and merge to form the basilar artery. Branches of this artery include the anterior and posterior inferior cerebellar arteries supplying the brainstem and the cerebellum alongside the superior cerebellar arteries. The basilar artery bifurcates into the posterior cerebral arteries and supplies the occipital lobes.
1.2.1 Circle of Willis
An anastomotic circle is formed around the optic chiasm between the anterior cerebral arteries (via the anterior communicating artery) and the posterior cerebral arteries (via the posterior communicating arteries).
1.2.2 Cerebral Venous Drainage
The superficial system of veins draining the cerebral cortex include the superior cerebral veins, middle cerebral veins, inferior cerebral veins, superior and inferior anastomotic veins. The superficial veins drain into the superior and inferior sagittal sinuses (Figure 1.4).
The deep veins include subependymal veins, the great cerebral vein, and medullary veins. The deep veins drain into the great cerebral vein and then to the straight or transverse sinuses.
The dural venous sinuses are valveless structures found between the periosteal and meningeal layer of the dura mater and drain into the internal jugular vein. The straight, superior, and inferior sagittal sinuses are found in the falx cerebri of the dura mater and converge at the confluence of sinuses. From here the transverse sinus, located bilaterally in the tentorium cerebelli, curves into the sigmoid sinus and then to the internal jugular vein, which exits at the jugular foramen. The great cerebral vein and the inferior sagittal sinus continue as the straight sinus.
The cavernous sinus is clinically relevant due to its vulnerability as a site of infection. It contains the internal carotid artery, abducens nerve, oculomotor nerve, trochlear nerve, and the ophthalmic and maxillary branches of the trigeminal nerve. The sinus is connected to the valveless facial vein via the superior ophthalmic vein, allowing reverse blood flow to the sinus, and is therefore a potential route of intracranial infection. The cavernous sinus also receives venous drainage from the central vein of the retina, the sphenoparietal sinus, the superficial middle cerebral vein, and the pterygoid plexus. These empty into the superior and inferior petrosal sinuses and, ultimately, into the internal jugular vein. The left and right cavernous sinuses are connected in the midline by the anterior and posterior intercavernous sinuses.
1.2.3 Lymphatics of the Brain
It has been a longstanding belief that the vertebrate brain does not contain a classic lymphatic drainage system. Instead, it was thought the brain has a “glymphatic system,” a perivascular pathway that allows cerebrospinal fluid (CSF) to recirculate through the brain interstitium along perivascular spaces near the cerebral arteries. This pathway was also thought to be responsible for the clearance of interstitial solutes along perivascular channels near the veins, mediated by astroglial water channels. However, recent findings have provided evidence of a functional lymphatic system in the brain, which acts to transport interstitial fluid (ISF) and solutes from the parenchyma to the deep cervical lymph nodes. The lymphatic system also helps maintain the water and ion balance of the ISF and allows communication with the immune system. The lymphatic vessels are associated with the superior sagittal and transverse sinus and the dural middle meningeal arteries. It is likely that the lymphatic system works in conjunction with other efflux pathways, such as via the dural arachnoid granulations.
1.3 Bones of the Skull
The skull is a supportive protection for the brain and is formed by intramembranous ossification of cranial and facial bones. The calvarium is the roof of the cranium and is comprised of the frontal, occipital, and parietal bones (Figure 1.5). The base of the cranium is comprised of the ethmoid, occipital, temporal, parietal, frontal, and sphenoid bones. The junction between the latter four bones is known as the pterion and is of clinical relevance because it overlies the middle meningeal artery, which can rupture following fractures to this region, leading to the formation of extradural hematomas.





Figure 1.5 The skull and facial skeleton.
Cranial fractures may be accompanied by facial bone fractures and should be sought for when assessing the trauma patient. The most common facial fractures include those of the nasal bone, maxilla, mandible, and zygomatic arch. Other areas prone to damage are the sutures, and these include the coronal, sagittal, and lambdoid sutures (Figure 1.6). In neonates, incompletely fused sutures give rise to fontanelles – the frontal fontanelle between the coronal and sagittal sutures and the occipital fontanelle between the sagittal and lambdoid sutures.

Figure 1.6 Sutures of the brain.
1.4 The Cranial Fossae
The cranial cavity is divided into three regions known as fossae. The anterior cranial fossa overlies the nasal and orbital regions and accommodates parts of the frontal lobe. It is made up of the frontal, ethmoid, and sphenoid bones. The frontal crest on the frontal bone and the crista galli of the ethmoid bone are the sites of attachment for a part of the dura mater that divides the cerebral hemispheres, known as the falx cerebri. The cribriform plate supporting the olfactory bulb is lateral to the crista galli, and contains a foramen transmitting the olfactory nerve and two ethmoidal foramina (anterior and posterior, transmitting the anterior and posterior ethmoidal vessels and nerves, respectively). The plate is very thin and can fracture following facial trauma, resulting in CSF rhinorrhea and anosmia.
The anterior fossa is separated from the middle fossa by the lesser wing of the sphenoid bone. The anterior clinoid processes of these bones are the site of attachment for the tentorium cerebelli (dura mater dividing the cerebrum and cerebellum). The middle cranial fossa consists of the sphenoid and temporal bones. Within the central part of the fossa is the sella turcica, a bony prominence which supports the pituitary gland within the hypophysial fossa. The posterior wall of the sella turcica is formed by the dorsal sellae, which separate the middle cranial fossa from the posterior cranial fossa. The lateral parts of the middle cranial fossa are formed by the greater wings of the sphenoid bone and the squamous and petrous parts of the temporal bones and provide structural support to the temporal lobes. Both the sphenoid and temporal bones contain numerous foramina for transmitting vessels and nerves. The posterior cranial fossa is comprised of the occipital bone and the temporal bones and contains the brainstem and cerebellum. The foramina of the skull are most considered in the context of the cranial nerves and are shown in Figure 1.7.

Figure 1.7 Superior view of the skull base showing the foramina.
1.5 Layers of the Scalp
The scalp contains several layers, and these include skin, dense connective tissue, epicranial aponeurosis, loose areolar connective tissue, and the periosteum (Figure 1.8). The scalp is supplied by branches of the external carotid artery (superficial temporal, posterior auricular, and occipital arteries) and the ophthalmic artery (supraorbital and supratrochlear arteries). Venous drainage includes a superficial system following the arterial supply (superficial temporal, occipital, posterior auricular, supraorbital, and supratrochlear veins). The temporal region of the skull is drained by the pterygoid venous plexus, which drains into the maxillary vein. The scalp veins connect to the diploic veins of the skull via valveless emissary veins, allowing a connection between the scalp and the dural venous sinuses. The nerves innervating the scalp include the trigeminal nerve branches (supratrochlear, supraorbital, zygomaticotemporal, and auriculotemporal nerves). The cervical nerve branches supplying the scalp include the lesser occipital nerve, the greater occipital nerve, the great auricular nerve, and the third occipital nerve.

Figure 1.8 Layers of the scalp.
1.6 Meninges
The brain and spinal cord are covered with membranous layers called the meninges. From outer to inner these are the dura, arachnoid, and pia mater.
1.6.1 Dura Mater
This layer is found underneath the bones and consists of the outer periosteal layer and the inner meningeal layer and in between houses the dural venous sinuses. The meningeal layer folds inward to form the dural reflections and forms compartments. These compartments are the falx cerebri, the tentorium cerebelli, the falx cerebelli (separates the cerebellar hemispheres), and the diaphragma sellae (allows the passage of the pituitary stalk). Blood collecting in between the skull and the outer periosteal layer is known as an extradural hematoma and usually occurs due to damage to the middle meningeal artery. A subdural hematoma occurs due to damage of the cerebral veins between the dura and the arachnoid mater.
1.6.2 Arachnoid Mater
The arachnoid mater consists of avascular connective tissue. Below it lies the subarachnoid space containing CSF, which re-enters the circulation via the dural venous sinuses through small projections called arachnoid granulations.
1.6.3 Pia Mater
This is a highly vascularized layer that adheres to the brain surface and follows the contours of the brain into the gyri of the cerebral hemispheres and the folia of the cerebellum. The pia mater and arachnoid mater are joined by connective tissue in the subarachnoid space and together are known as leptomeninges. There are compartments where the two are not in close approximation, resulting in naturally enlarged CSF filled pools called the subarachnoid cisterns.
1.7 Organization of the Sympathetic and Parasympathetic Nervous Systems
The autonomic nervous system is composed of the sympathetic nervous system (SNS) and the parasympathetic nervous system (PNS) and acts to regulate the body’s unconscious actions. The SNS stimulates the so-called “fight or flight” response and the PNS is involved in “rest and digest” responses (Box 1.1).
Box 1.1
Organ | Parasympathetic Response | Sympathetic Response |
---|---|---|
Iris | Miosis and accommodation due to constriction of the sphincter muscles via the short ciliary nerves originating from the Edinger–Westphal nucleus of cranial nerve III | Pupil dilation (adrenergic innervation to the dilator pupillae muscle via the long ciliary nerves, arising from the superior cervical ganglion) |
Salivary glands | Increased watery secretion via cranial nerves IX (parotid gland) and chorda tympani of VII (submandibular and sublingual glands) leading to acetylcholine release onto M3 muscarinic receptors | Reduced saliva secretion (innervation via fibers arising from the superior cervical ganglion resulting in norepinephrine release acting on alpha- and beta-adrenergic receptors) |
Lacrimal glands | Increased secretion (preganglionic fibers reach the pterygopalatine ganglion via the greater petrosal nerve and the nerve of the pterygoid canal to synapse with postganglionic fibers) | Reduced secretion (innervation via fibers originating in the superior cervical ganglion, which reach the pterygopalatine ganglion via the internal carotid plexus and the deep petrosal nerve) |
Heart | Negative chronotopy, inotropy and reduced conduction velocity and coronary artery vasoconstriction via the vagus nerve | Positive chronotropy, inotropy and increased conduction velocity via the cardiac nerves from the lower cervical and upper thoracic ganglia |
Lung | Bronchial muscle contraction (vagus nerve) | Bronchial muscle relaxation (thoracic sympathetic ganglia) |
Stomach | Increased peristalsis and motility and pyloric sphincter relaxation allowing gastric emptying via the vagus nerve | Reduced gastric motility and peristalsis and pyloric sphincter constriction preventing gastric emptying via the celiac plexus (T5–T12) |
Gallbladder | Contraction (vagus nerve) | Relaxation (T7–T9 through the celiac plexus) |
Internal urethral sphincter | Relaxation | Constriction |
Detrusor muscle of bladder | Contraction (via pelvic splanchnic nerves to allow bladder emptying) | Relaxation (via sympathetic branches from the inferior hypogastric plexus to allow bladder filling) |
Penis | Erection (pelvic nerve) | Ejaculation (peristaltic contraction of vas deferens, seminal vesicles, and prostatic smooth muscles via the hypogastric nerve and ejaculation via the pudendal nerve) |
Adrenal medulla | Norepinephrine and epinephrine secretion |
A major anatomical component of the SNS are a pair of nerve fibers that span the skull to coccyx, known as the sympathetic chains. There are two types of neurons involved in sympathetic signal transmission and these are the short preganglionic neurons that originate from T1 to L2–L3, which synapse with postganglionic neurons that extends to the rest of the body. At the synapses, the preganglionic neurons release acetylcholine, which activates the nicotinic acetylcholine receptors in the postganglionic neurons to release norepinephrine, and these subsequently bind to adrenergic receptors in the target tissue leading to sympathetic effects (Figure 1.9). The postganglionic neurons of sweat glands release acetylcholine to activate muscarinic receptors. The chromaffin cells of the adrenal medulla act as a postganglionic neuron and release norepinephrine and epinephrine.
Sympathetic nerves arise in the spinal cord in the intermediolateral nucleus of the lateral gray column. Axons leave the spinal cord through the anterior root and pass near the sensory ganglion to enter the anterior rami of spinal nerves. The axons terminate at the paravertebral or prevertebral ganglia. The main prevertebral ganglia (celiac, mesenteric and aorticorenal ganglia) are located anterior to the aorta and vertebral column and receive preganglionic axons via the splanchnic nerves. The paravertebral ganglia are located bilaterally ventrolateral to the vertebral column. There are three paravertebral ganglia and these are the superior cervical ganglion, middle cervical ganglion and inferior cervical ganglion.
1.7.1 Sympathetic Ganglia Supplying the Head and Neck: Cervical Ganglia
There are three cervical ganglia that supply the head and neck (the superior, middle, and inferior cervical ganglia). The superior ganglion is found posterior to the carotid artery and gives rise to a number of postganglionic nerves: the internal and external carotid nerves, the nerve to the pharyngeal plexus, the superior cardiac branch, and the gray rami communicantes. The middle ganglion may be absent, but when present is found anterior to the inferior thyroid artery. Its postganglionic fibers are the gray rami communicantes, the thyroid branches, and the middle cardiac branch. The inferior cervical ganglion is situated anteriorly to the C7 vertebra. Its branches are the gray rami communicantes, branches to the subclavian and vertebral arteries, and the inferior cardiac nerve. Damage to sympathetic fibers en route to the head and neck can lead to Horner’s syndrome, a condition presenting with partial ptosis of the upper eyelid, miosis (constricted pupil) and hemi-facial anhidrosis (absence of sweating).
1.7.2 Parasympathetic Ganglia Supplying the Head and Neck
The parasympathetic fibers supplying the head and neck are found in four brainstem nuclei associated with a cranial nerve. They synapse in a peripheral ganglion near the target viscera. There are four parasympathetic ganglia located within the head –ciliary, otic, pterygopalatine, and submandibular. They receive fibers from the oculomotor, facial, and glossopharyngeal nerves (the vagus nerve only innervates structures in the thorax and abdomen).
The ciliary ganglion is located within the bony orbit. Its preganglionic fibers are from the Edinger–Westphal nucleus, associated with the oculomotor nerve. Its postganglionic fibers leave the ganglion via the short ciliary nerves to innervate the sphincter pupillae and the ciliary muscles. Sympathetic nerves from the internal carotid plexus and sensory fibers from the nasocilary nerve pass through the ganglion without synapsing.
The pterygopalatine ganglion is located within the pterygopalatine fossa and is supplied by fibers from the superior salivatory nucleus (associated with the facial nerve). Its postganglionic fibers join branches of the maxillary nerve to supply the lacrimal gland, the nasopharynx, and the palate.
Sympathetic fibers from the internal carotid plexus and sensory branches from the maxillary nerve pass through the pterygopalatine ganglion without synapsing.
The submandibular ganglion is located inferiorly to the lingual nerve and is supplied by fibers from the superior salivatory nucleus. These fibers are carried within a branch of the facial nerve, the chorda tympani. This nerve travels along the lingual branch of the mandibular nerve to reach the ganglion and leaves the ganglion to the submandibular and sublingual glands. Sympathetic fibers from the facial artery plexus pass through the submandibular ganglion. They are thought to innervate glands in the base of the oral cavity.
The otic ganglion is located inferiorly to the foramen ovale within the infratemporal fossa. It is medial to the mandibular branch of the trigeminal nerve. The ganglion is supplied by fibers from the inferior salivatory nucleus (associated with the glossopharyngeal nerve). Parasympathetic fibers travel within the lesser petrosal nerve, a branch of the glossopharyngeal nerve, to reach the otic ganglion. The parasympathetic fibers travel along the auriculotemporal nerve (a branch of the mandibular division of the trigeminal nerve) to provide secretomotor innervation to the parotid gland. Sympathetic fibers from the superior cervical chain pass through the otic ganglion, where they travel with the middle meningeal artery to innervate the parotid gland.
1.8 Structures of the Brain
The nervous system forms during the third week of development. At the cranial end of the neural tube, three expansions (vesicles) develop: the forebrain (prosencephalon), the midbrain (mesencephalon), and the hindbrain (rhombencephalon). Further division separates the prosencephalon into the diencephalon (thalamus and hypothalamus) and the telencephalon (cerebrum). The mesencephalon consists of the tectum, cerebral aqueduct, tegmentum, and the cerebral peduncles. The rhomboencephalon consists of the pons, the medulla, and the cerebellum. The cavities within the primary brain vesicles are precursors of the ventricular system. The caudal parts of the neural tube form the spinal cord.
1.9 Forebrain
The structures in the forebrain include the cerebral cortex and subcortical structures of the limbic system including the amygdala, hypothalamus, thalamus, hippocampus, basal ganglia, and cingulate gyrus.
1.10 Cerebrum
The largest part of the brain is the cerebrum, containing two hemispheres separated by the falx cerebri of the dura mater. The visible surface of the cerebral hemisphere is a folded sheet of neural tissue called the cerebral cortex, characterized by sulci (depressions) and gyri (elevations). Some of the larger folds include the lateral sulcus (also known as the Sylvian fissure), which separates the temporal lobe from the frontal and parietal lobes, the central sulcus (dividing the frontal and parietal lobes), and the parieto-occipital sulcus, which separates the occipital and parietal lobes (Figure 1.10). The two cerebral hemispheres are connected by a white-matter tract called the corpus callosum. The tissue separating the two hemispheres is called the longitudinal fissure. The septum pellucidum continues from the corpus callosum to the fornix and separates the anterior horns of the lateral ventricles. The calcarine fissure separates the occipital lobe into the inferior lingual gyrus and the superior cuneus.
The cerebrum is made up of gray matter (containing cell bodies and dendrites) and white matter (consisting of glial cells and myelinated axons). The cerebrum can be divided into four lobes. The frontal lobe subserve decision-making and executive control. The parietal lobe is vital for sensory perception and integration. The occipital lobe is the visuospatial processing area of the brain for color, form and motion. The temporal lobe contains cortical areas that process auditory stimuli, encoding of memory and language comprehension.
1.10.1 Brodmann’s Map
The cerebral cortex can also be subdivided into 52 functional regions, numbered by the neuroanatomist Korbinian Brodmann based on cytological structure. The primary motor cortex (Brodmann area 4) is anterior to the central sulcus (Figure 1.10). It contains large neurons called Betz cells, which send axons to the spinal cord and is important for planning and execution of movements. The topographic map of the motor cortex is arranged with an “overrepresentation” of neurons responsible for complex motor behaviors (Figure 1.11). The premotor cortex (Brodmann area 6) also plays a role in planning movement; however, its function is less well understood. The supplementary motor area (Brodmann area 6) contributes to the control of movement. The primary somatosensory cortex (Brodmann areas 3, 2, and 1) is found in the parietal lobe, posterior to the central sulcus. It processes afferent somatosensory input and helps integrate sensory and motor information required for skilled movement. The primary visual cortex (Brodmann area 17) is at the occipital pole. The extra striate cortex is adjacent to the visual cortex and processes specific features of visual information. It can be separated into two streams. The ventral stream is from the primary visual cortex to the temporal lobe and is important for pattern and object recognition. The dorsal stream from the striate cortex into the parietal lobe is responsible for spatial recognition of motion and location. The primary auditory cortex is responsible for recognition of auditory stimuli and is in the lateral temporal lobe. Wernicke’s area (Brodmann area 22) is located in the superior temporal gyrus of the dominant cerebral hemi- sphere. It is important for comprehension of written and spoken language, and therefore any damage to this area leads to fluent but nonsensical speech. Broca’s area (Brodmann areas 44 and 45) is located in the dominant prefrontal cortex and is involved in language processing and speech production. Lesions in this region lead to expressive aphasia, where the patient retains comprehension but cannot create fluent speech.
1.10.2 Layers of The Cerebral Cortex
The cerebral neocortex is arranged in six layers. The outermost layer is the molecular layer (layer I), containing fibers that run parallel to the cortical surface with very few neurons. Layer II is the outer granular layer, containing small, rounded neurons. Below this is the outer pyramidal layer (layer III), containing pyramidal neurons. Next is the inner granular layer (layer IV), containing spiny stellate and pyramidal cells. It is a major input layer and receives specific sensory inputs from thalamocortical afferent fibers. The primary sensory cortex has a well-developed layer IV, but the output layers are less well developed. Layer IV is well developed in the primary visual cortex and contains the stria of Gennari, a band of myelinated axons that runs parallel to the surface of the cerebral cortex. The inner pyramidal later (layer V) contains many output neurons. The primary motor cortex has a very enlarged layer V containing large Betz cells, which send axons to the contralateral motor nuclei of cranial nerves and to the lower motor neurons in the ventral horn of the spinal cord. Finally, layer VI is the innermost, multiform layer containing morphologically heterogenous population of neurons and sends efferent fibers to the thalamus.
Certain parts of the cortex are arranged differently: the hippocampus has three layers and the cingulate gyrus has four to five layers.
1.10.3 Vascular Supply
The branches of the anterior, middle, and posterior cerebral arteries are responsible for the blood supply to the cerebrum. The venous drainage of the cerebrum is via cerebral veins that empty into the dural venous sinuses (Figure 1.4).
1.11 Ventricles
The ventricles (Figure 1.12) are lined by ependymal cells, ciliated glial cells, which form the choroid plexus, a structure where CSF is produced (Figure 1.13). This provides hydromechanical protection by acting as a shock absorber and providing buoyancy.

Figure 1.12 The ventricular system

Figure 1.13 Histology of the choroid plexus
The ventricular system is composed of four connecting cavities derived from the neural tube. The right and left ventricles and the third ventricle are part of the forebrain, while the fourth ventricle is part of the hindbrain.
During development, the fluid-filled cavity of the primary vesicles become the lateral ventricles. They communicate with the third ventricle via the foramen of Monro in the diencephalon. The third ventricle communicates with the fourth ventricle in the hindbrain via the cerebral aqueduct (of Sylvius), which is continuous with the central canal of the spinal cord. The fourth ventricle is also connected to the subarachnoid space by a median aperture (the foramen of Magendie) and two lateral apertures (the foramina of Luschka).
1.12 Higher Association Areas of the Cortex
Higher association areas of the cortex are involved in complex processing of various sensory modalities, cognition and emotion. The prefrontal and limbic association areas are important for regulation of cognition, abstract reasoning, complex emotions and self-awareness. The cingulate and parahippocampal gyri are involved in expression of emotions and formation of memories. The hippocampal formation is important for declarative memory. It contains the Cornu Ammonis (CA) regions, the subiculum, and the dentate gyrus, and is found in the medial temporal lobe in the inferior horn of the lateral ventricle. The amygdala is a subcortical nucleus in the medial temporal lobe and is connected to the orbitofrontal cortex, the hypothalamus, and the nucleus accumbens.
1.13 Limbic System
The limbic system is a part of the brain involved in behavioral and emotional responses. There are several important structures within the limbic system and these include the basal ganglia, thalamus, hypothalamus, hippocampus, amygdala, and the cingulate gyrus.
1.13.1 Basal Ganglia
The basal ganglia are situated at the base of the forebrain and top of the midbrain. They are a group of subcortical nuclei connected to the cerebral cortex, thalamus, and the brainstem. The basal ganglia are responsible for control of voluntary motor movements, procedural learning, cognition, and emotion. They con- sist of several distinct structures (Figure 1.14) and these include the caudate nucleus and putamen (together known as the neostriatum) separated by the internal capsule). The internal capsule is the site of the passage of many fibers including the efferent corticobulbar fibers, corticospinal fibers, efferent corticopontine fibers, and afferent thalamocortical fibers.

Figure 1.14 Cross-section of the basal ganglia.
The caudate receives inputs from the prefrontal cortex and the putamen receives inputs from the sensorimotor cortex. They send outputs to the external and internal globus pallidus and then to the thalamus and cerebral cortex, forming a subcortical loop. The internal segment projects to the motor areas of the thalamus and the medial nucleus of the thalamus. The external segment projects to the subthalamic nucleus (STN), which also projects to the internal globus pallidus.
The neostriatum also projects to the substantia nigra par compacta (SNPC), which contains dopaminergic neurons and to the pars reticulata, which contains mainly GABAergic neurons and is one of the output nuclei of the basal ganglia to the thalamus (alongside the internal globus pallidus) and plays a vital role in movement execution. The neostriatum is supplied by small branches of the middle and anterior cerebral arteries. The neostriatum and internal capsule are commonly affected in stroke. Damage to the internal capsule leads to contralateral weakness. The most affected region of the internal capsule is the genu, where corticospinal fibers to the head, neck, and part of the upper limb are located.
1.13.1.1 Connections of the Basal Ganglia
The striatum receives input from the cerebral cortex and the SNPC (Figure 1.15). The striatum sends inhibitory connections to the internal and external globus pallidus. The external region therefore disinhibits the STN, which then sends excitatory input to the internal globus pallidus. This sends inhibitory input to the thalamus, leading to inhibition of information flow to the cerebral cortex.

Figure 1.15 Basal ganglia connections.
The basal ganglia also project to the medial dorsal nucleus of the thalamus, which then projects to the prefrontal association cortex. This region is involved in higher cortical and executive function.
Parkinson’s disease results from degeneration of dopaminergic neurons of the SNPC and excessive inhibition of the thalamus. The SNPC projects to both direct and indirect pathways in the striatum. Due to the presence of two different types of dopamine receptors, the net effect is to excite the direct pathway and inhibit the indirect pathway. However, the loss of the neurons in Parkinson’s disease upsets the fine balance of these pathways and reduces excitation of the motor cortex, resulting in poverty of movement.
1.13.2 Thalamus
The thalamus is located in the forebrain and contains nuclei with connections to the cerebral cortex, the hippocampus, the mammillary bodies, and the fornix.
The thalamus is divided into three major nuclear groups (anterior, medial, and ventral) (Figure 1.16). The ventral group contains ascending somatosensory relays (ventroposterior nuclei) and relays from the cerebellum and basal ganglia (ventrolateral). It also contains the motor association areas (ventro-anterior nuclei). The lateral and medial geniculate nuclei are found posteriorly. The anterior group projects to the cingulate gyrus and receives input from the mammillary bodies of the hypothalamus. The medial nuclei and the pulvinar receive input from the cerebral cortex and form the cortico-thalamo-cortical relays, which project to areas of the association cortex. These are the prefrontal cortex and the temporal–parietal–occipital association cortex, which mainly receive input from the medial nuclei and the pulvinar, respectively.

Figure 1.16 Nuclei of the thalamus.
The thalamus is supplied by the posterior cerebral artery and branches of the posterior communicating artery. The thalamus is involved in learning, episodic memory, regulation of sleep, and wakefulness. Lesions in the anterior thalamus can lead to obstruction of the interventricular foramen of Monro and lesions in the posteriomedial thalamus can obstruct the third ventricle and cerebral aqueduct, leading to the development of hydrocephalus.
1.13.3 Cingulate Gyrus
The cingulate gyrus is situated above the corpus callosum (Figure 1.10). The anterior part relays signals between the right and left hemispheres and is involved in autonomic functions and cognitive processes such as reward behavior, empathy, and emotion. The posterior part becomes continuous with the most medial part of the temporal lobe, the parahippocampal gyrus. The cingulate gyrus is thought to be involved in retrieving episodic memory information. Dysfunction of this gyrus is found in schizophrenia and depression. Deep brain stimulation of the subgenual cortex of the gyrus is used to treat intractable depression. Output fibers are mainly to the parahippocampal gyrus and are linked by the cingulum, a white-matter tract.
1.13.4 Hippocampus
The hippocampus is a seahorse-shaped structure and the hippocampal formation refers to the hippocampus proper (Cornu Ammonis), the dentate gyrus, and the subiculum. It is found in the temporal lobe, medial to the inferior horn of the lateral ventricle.
The hippocampus has been studied extensively and is used as a model system for electrophysiological studies, particularly for investigating neural plasticity. Damage to the hippocampus is commonly seen in patients with dementia, particularly Alzheimer’s disease.
Hippocampal tissue is made up of layers and these include, from outer to inner: an external plexiform layer; a stratum oriens layer containing basal dendrites and basket cells; a pyramidal cell layer containing the primary cells of the hippocampus; a stratum radiatum layer; and the stratum lacunosum-moleculare layers containing the perforate pathway made up of pyramidal cell apical dendrites and afferent fibers from the entorhinal cortex. The external plexiform layer contains the alvear pathway, and this contains pyramidal cell axons through which information from the hippocampus is passed to the inferior horn of the lateral ventricle before reaching the entorhinal cortex. In addition, the hippocampus also contains distinct regions. The shape of the hippocampus has been described as similar to a seahorse or a ram’s horn (Cornu Ammonis). The abbreviation CA is used to name the different regions: CA1, CA2, CA3, and CA4 (Figure 1.17).

Figure 1.17 Cornu Ammonis axons of the hippocampus.
1.13.4.1 Dentate Gyrus
The dentate gyrus contains granule cells and axons called mossy fibers, which synapse with the pyramidal cells in the CA3 field of the hippocampus. They also contain some pyramidal cells in the polymorphic cell layer.
1.13.4.2 Hippocampal Inputs
The hippocampus receives information from the lateral perforate and medial perforate pathways in the entorhinal cortex (Figure 1.18), the prefrontal cortex, the anterior cingulate gyrus, the pre- mammillary region, and the reticular formation of the brainstem. It also receives input from the thalamus to field CA1 and from the serotonin, norepineph- rine, and dopamine systems. The medial septal nucleus sends cholinergic and γ-aminobutyric acid (GABA)-ergic inputs to the hippocampus.

Figure 1.18 Hippocampal connections.
The largest input and output pathway of the hippocampus is via the fornix, which connects it to other structures including the mammillary bodies of the hypothalamus, prefrontal cortex and the lateral septal area. The entorhinal cortex (part of the parahippocampal gyrus) receives output from the deeper layers of the hippocampus and gives input to the superficial layers.
The fornix has two branches: the precommissural and the postcommissural pathways. The former connects to the septal nuclei, preoptic nuclei, ventral striatum, orbital cortex, and anterior cingulate gyrus. The postcommissural branch connects to the anterior nucleus of the thalamus and mammillary bodies of the hypothalamus. In Korsakoff’s syndrome, these bodies are damaged and hence patients have trouble learning new memories. The anterior thalamic nuclei connect to the cingulate cortex, which connects back to the entorhinal cortex and creates the Papez circuit, which is involved in learning, memory, and emotion.
1.13.4.3 Hippocampus and Memory
Memory is the ability to acquire, store, and retrieve information and are formed through learning. The process of learning activates engram cells, a population of cells that have undergone cellular changes as a result of learning and when reactivated by the original stimulus leads to memory recall.
1.13.4.3.1 Spatial Memory
The hippocampus is well known to be involved in spatial memory and navigation. Within the hippocampus are found place cells, which contain information regarding the spatial context in which a memory took place in one specific location. These cells cluster in place fields and fire action potentials when an animal passes a certain location. Place-cell responses have been seen in the pyramidal cells of the hippocampus and the granule cells of the dentate gyrus. This is in contrast to the grid cells found in the entorhinal cortex, which generate a map of firing patterns covering entire regions. The spatial firing sequences appear to be stored in the hippocampus during exploration and can be retrieved at a later time. The dorsal hippocampus is responsible for spatial memory, verbal memory, and learning conceptual information, and there appear to be more place cells in the dorsal region. The ventral hippocampus functions in fear conditioning and affective processes.
1.13.4.3.2 Explicit Versus Implicit Memory
Declarative or explicit memories are available in the consciousness as semantic facts or episodic memories (Figure 1.19). The areas of the brain involved are the hippocampus, the neocortex, and the amygdala. Non-declarative memories, also known as implicit memories, are memories of skills, and rely on the basal ganglia and cerebellum. Short-term memories (seconds to minutes) are brief memories including working memory and rely heavily on the prefrontal cortex. These memories can be consolidated into long-term memory.
Formation of explicit memory begins with consolidation and storage of encoded information in the hippocampus. Over time, certain memories can be transferred to the neocortex as general knowledge. The amygdala interacts with the hippocampus and neocortex to stabilize a memory and form new memories related to fear.
One of the earlier findings of how the hippocampus impacts memory consolidation came from the study of Henry Molaison, a patient who had a bilateral temporal lobectomy to treat his epileptic seizures. The surgery led to deficits including an inability to complete tasks that required long-term episodic memory and an inability to create new long-term memories. His spatial orientation was also severely affected. His long-term memory of events many years before the accident was largely intact and his procedural memory and intelligence were unaffected. This showed that the medial temporal lobe containing the hippocampus is important for declarative but not procedural memory consolidation.
Neurophysiological theories of synaptic plasticity based on the cellular models that underpin synaptic plasticity including long-term potentiation (LTP) and long-term depression (LTD) (see Chapter 5 for further details) can be used to explain the neural basis of learning and memory. Donald Hebb was the first to postulate that activation or inactivation of extant synaptic contacts depends on the synchronous impulse activity of pre- and postsynaptic nerve cells.
One of the most fascinating features of the hippocampus is its capacity for plasticity. Action potentials travel down the Schaffer collaterals and stimulate the release of glutamate into the synaptic cleft, which stimulates the α-amino-3-hydroxy-5-methyl-4-isoxazo-lepropionic acid (AMPA) and N-methyl-d-aspartate (NMDA) receptors. Once the AMPA receptors open, sodium travels into the postsynaptic membrane and elicits a membrane depolarization. With a large enough stimulation, a large amount of glutamate will be released, allowing a large membrane depolarization that removes the magnesium block typically seen at the NMDA receptor. This allows both sodium and calcium to enter the postsynaptic membrane. The calcium activates protein kinases and stimulates a cascade of short- and long-term changes. In the short term, there is insertion of AMPA receptors (Figure 1.20), and in the long term there are changes in transcription factors and increased protein translation. This forms the basis of LTP.
On the contrary, LTD is induced by prolonged low-frequency stimulation, which leads to prolonged calcium activation of phosphatase enzymes and an eventual removal of AMPA receptors and pruning of spines.
1.13.4.3.3 Implicit Memory
Implicit memory involves several different brain regions. Classical conditioning involves various sensory and motor systems. Operant conditioning involves the striatum and cerebellum and fear conditioning involves the amygdala.
Classic (Pavlov) conditioning refers to learning that occurs when a neutral stimulus becomes associated with a stimulus that naturally produces a behavior. It was first observed by the Russian physiologist Ivan Pavlov, who exposed dogs to sounds (neutral stimulus) immediately before receiving food (natural stimulus producing behavior). Initially, the dogs began to salivate only when they saw the food; however, later, the dogs learned to associate the sound with the food and began salivating as they heard the sound. The food is the unconditioned stimulus as it naturally leads to a behavior. The conditioned stimulus is the neutral tone, which upon repeated presentation leads to the same behavior as the unconditioned stimulus. Classic conditioning involves enhanced synaptic strength due to presynaptic facilitation, where there is increased release of neurotransmitters from the presynaptic cells under the action of an additional neuron.
Habituation is a type of non-associative learning where a repetitive stimulus leads to a decrement in the response intensity. Sensitization is the increment in response intensity in response to a stimulus. Experiments performed in the sea slug, Aplysia californica, by Eric Kandel were crucial in establishing the importance of synaptic changes to learning and memory. It was demonstrated that upon stimulation of the siphon receptors, the motor neuron is activated directly or indirectly through the excitatory interneuron as the gill is withdrawn. However, with repeated stimulation, there is reduced release of synaptic transmitters from the sensory neurons and therefore there is less withdrawal of the gill (habituation). This would typically occur when an animal repeatedly encounters a harmless stimulus. However, when the animal encounters a harmful stimulus, a vigorous response occurs to not only the harmful stimulus but also the harmless stimulus (sensitization). When the head or tail of the Aplysia is stimulated by an electric shock, there is activation of the facilitatory interneurons. These synapse with the sensory neurons of the head, and there is increased transmitter release leading to excitation of the excitatory interneurons and the motor neurons to the gill (Figure 1.21). Dishabituation refers to the restoration of a full-strength response that was weakened by habituation.
Operant conditioning, is learning that occurs based on the consequences of behavior (e.g. reinforcement or punishment). This is a voluntary behavioral response and according to the law of effect, the behavior is strengthened or weakened by the learner based on their desired result. The early studies on operant conditioning were performed by Edward Thorndike and B. F. Skinner. The “Skinner box” was designed to study the principles of animal behavior in a controlled environment.
1.13.5 Amygdala
The amygdala is one of two almond-shaped clusters of nuclei found medially within the temporal lobe and is responsible for emotions such as fear and aggression. Emotion is a subjective state and consists of a physical response involving the autonomic motor and endocrine systems. It also requires conscious registration, and this involves both the cerebral cortex and the amygdala. The amygdala is also involved in memory formation, reward processing, and decision making.
1.13.5.1 Inputs
The amygdala receives inputs from the hypothalamus, septal area, and the orbital cortex.
1.13.5.2 Outputs
The major outputs of the amygdala include the ventral amygdalofugal pathway, the stria terminalis, the hippocampus, the entorhinal cortex, and the dorsomedial nucleus of the thalamus. The amygdala connects to the hypothalamus via the stria terminalis and the ventral amygdalofugal pathway, the latter of which also connects to the anterior olfactory nucleus, the anterior perforated substance in the forebrain, the piriform cortex, the orbitofrontal cortex, the anterior cingulate cortex, and the ventral striatum. This pathway is particularly important in associative learning and the linking of behavior with reward and punishment. The stria terminalis continues as precommissural and postcommissural branches. The precommissural branch travels to the septal area and the postcommissural branch to the hypothalamus. The stria terminalis also projects to the habenula (involved in reward and aversion processing), which is part of the epithalamus. See section 1.15.
1.13.5.3 Amygdala and Fear Conditioning
The amygdala is involved in Pavlovian fear conditioning, a behavioral paradigm that involves learning to associate stimuli with certain adverse effects. In fear conditioning, an animal associates a neutral conditional stimulus, such as a tone, with an aversive unconditional stimulus, such as an electric foot shock, and responds by freezing even when it is not receiving the aversive stimulus. Fear extinction refers to the reduction in the conditioned fear responses after repeated presentation of the conditioned stimulus without the unconditioned stimulus that had elicited the fear.
1.13.6 Nucleus Accumbens
The nucleus accumbens is part of the striatum. It receives input from the orbitofrontal cortex, the cingulate cortex, and the amygdala and is involved in emotion and motivation.
1.13.7 Prefrontal Cortex
The prefrontal cortex is located in the frontal lobe and is implicated in personality development, decision making, and reasoning. Its role was discovered from the symp- toms and signs that Phineas Gage (an American railroad construction worker) developed following an accident that involved a rod being driven into his brain resulting in irreversible damage to his frontal lobe.
The medial part of the prefrontal cortex connects with the amygdala, the hippocampus, and the temporal lobe. The lateral part connects to the basal ganglia, the premotor cortex, the supplemental motor area, the thalamus, and the cingulate cortex. The orbitofrontal region forms connections with the amygdala, the medial part of the thalamus, the hypothalamus, and the basal ganglia.
1.13.8 Olfactory Bulb
The olfactory nerve projects to the olfactory bulb in the forebrain. The bulb is separated from the olfactory epithelium by the cribriform plate. It contains neurons involved in olfaction and receives sensory input from axons of the olfactory receptor neurons in the olfactory epithelium and outputs to the mitral cell axons. It then sends olfactory information to the amygdala, the orbitofrontal cortex, and the hippocampus. It also receives information from the amygdala, the neocortex, the hippocampus, the locus coeruleus, and the substantia nigra.
The bulb is divided into the main and accessory parts. The main bulb connects to the amygdala via the piriform cortex of the primary olfactory cortex. Associative learning between certain odors and behaviors takes place in the amygdala. The accessory bulb forms a parallel pathway.
1.13.9 Hypothalamus
The hypothalamus is bordered by the optic chiasm anteriorly and the mammillary bodies posteriorly. The function of the hypothalamus is to maintain homeostasis. It links the nervous system to the endocrine system via the pituitary gland, which is located below it. It contains four regional groups of nuclei (Figure 1.22): the preoptic region (which contains the preoptic nucleus), the supraoptic region (which contains suprachiasmatic, supraoptic, paraventricular, and anterior nuclei), the tuberal region (which contains dorsomedial, ventromedial, arcuate, premammillary and lateral tuberal nuclei), and the mammillary region (which contains mammillary and posterior nuclei). The medial preoptic nucleus secretes gonadotropin-releasing hormone (GnRH), which is responsible for the secretion of luteinizing hormone (LH) and follicle-stimulating hormone (FSH) by the pituitary. The paraventricular and supraoptic nuclei both produce the peptide hormones and antidiuretic hormone (ADH). The paraventricular nucleus also releases thyrotropin-releasing hormone (TRH), corticotropin-releasing hormone (CRH), and somatostatin. TRH is responsible for the formation and secretion of the thyroid-stimulating hormone (TSH) in the pituitary gland, which in turn regulates the production of thyroid hormones in the thyroid gland. TRH also stimulates the release of prolactin from the pituitary gland. Corticotropin-releasing hormone activates the release of adrenocorticotropic hormone (ACTH) from the pituitary gland. Somatostatin regulates the endocrine and digestive systems. The anterior nucleus is involved in thermoregulation by stimulating the PNS, and causes hyperthermia if damaged. The suprachiasmatic nucleus regulates the circadian rhythm and pineal gland function. The ventromedial nuclei mediate satiety. The lateral nuclei mediate hunger via orexin neurons. The arcuate nuclei secrete prolactin, growth hormone releasing hormone (GHRH), and GnRH. Dorsomedial nuclei regulate blood pressure and heart rate. The posterior nuclei release vasopressin and are involved in thermoregulation via the SNS. The mammillary nuclei are involved in memory.
1.13.9.1 Hypothalamus and Sleep
Sleep is a reversible state of reduced consciousness that is important for regulation of inflammatory processes, removal of toxins via the glymphatic system, maintenance of a reduced metabolic rate and memory consolidation. Sleep deprivation has negative consequences for the cardiovascular system, endocrine regulation, glucose tolerance and mental health. Sleep can be measured using polysomnography (PSG), which has been used to reveal distinct sleep states. Normally, people transition between non-rapid eye movement (NREM) stages and rapid eye movement (REM) stages and this is regulated by reciprocal inhibition of monoaminergic and cholinergic neurons. There is increased activity of cholinergic neurons and decreased activity of adrenergic and serotonergic neurons during REM sleep and this is reversed in NREM sleep. NREM sleep occurs during the transition from being awake to sleeping and is characterized by light sleeping with slowing of brain waves. Rapid eye movement sleep is characterized by rapid eye movements with faster breathing and increased heart rate and blood pressure.
The sleep cycle is regulated by the circadian rhythm and is controlled by the suprachiasmatic nucleus (SCN) of the hypothalamus. The circadian rhythm is a biological clock maintained in a 24-hour pattern by clock genes such as Per, tim, and Cry. The retina sends inputs to the SCN, which can regulate the circadian rhythm and sleep by releasing a number of hormones such as norepinephrine, and this can stimulate the pineal gland to release melatonin. Activation of the ventrolateral preoptic (VLPO) area is also important in initiating sleep. Wakefulness is regulated by ascending arousal pathways activating the cortical system and uses chemicals such as norepinephrine, serotonin, dopamine, acetylcholine, histamine, and orexin.
1.14 Pituitary Gland
The pituitary gland is functionally and anatomically linked to the hypothalamus (via the infundibulum; Figure 1.23) and is responsible for releasing several hormones. It is located in the sella turcica of the sphenoid bone and is covered by the diaphragma sellae. The gland is divided into the anterior (adenohypophysis) lobe – derived from an outpouching of the roof of the pharynx called Rathke’s pouch – and the posterior lobe. The anterior lobe is divided into three parts: Pars anterior (hormone secretion), Pars intermedia, and Pars tuberalis. The posterior (neurohypophysis) lobe releases two hormones (oxytocin and vasopressin) that are initially produced in the hypothalamus.
The anterior pituitary is supplied by the superior hypophyseal artery (a branch of the internal carotid artery), which forms a capillary network and a plexus called the hypophyseal portal system. The infundibulum and posterior pituitary gland are supplied by the superior hypophyseal artery, the infundibular artery, and the inferior hypophyseal artery. Both lobes are drained by the anterior and posterior hypophyseal veins.
1.15 Epithalamus
The epithalamus is a dorsal segment of the diencephalon and contains the pineal gland, habenula and stria medullaris. The pineal gland is responsible for secreting melatonin, a hormone involved in the regulation of the circadian rhythm of the body. This gland is supplied by the posterior choroidal arteries (a branch of the posterior cerebral artery) and drains to the internal cerebral veins. The stria medullaris, contains afferent fibers from the septal nuclei and the anterior thalamic nuclei to the habenula, bilateral structures connected by a commissure. The lateral habenula is primarily involved in learning from reward omission and aversive experiences, affect, cognition, and social behavior. The medial habenula may also be involved in fear responses, mood and memory.
1.16 Midbrain
The midbrain (mesencephalon) is part of the brainstem and lies above the pons and below the forebrain (Figure 1.24). It is comprised of two parts: the tectum and the cerebral peduncles (crus cerebra and tegmentum). The tectum houses four colliculi inferior to the pineal gland and superior to the trochlear nerve. The oculomotor nerve exits between the peduncles and the optic tract is found on the superior border.
The cross-section of the midbrain reveals several fiber tracts, and these include the frontopontine fibers, the corticospinal fibers, the corticobulbar tracts, and the temporopontine fibers. The substantia nigra, the tegmentum, and the tectum can be visualized posteriorly. The cerebral aqueduct and the periaqueductal gray matter can be seen in the midline, and the medial longitudinal fasciculus can be seen anteriorly. The red nuclei (which receive input from the cerebral cortex and cerebellum and are involved in coordination of sensorimotor information) and decussation of the rubrospinal tracts (motor control, modulation of flexor muscle tone, reflex activity, and inhibition of antigravity muscles) can be seen at the level of the superior colliculus. The oculomotor nucleus can be seen with the oculomotor nerve projecting anteriorly (Figure 1.24). The trochlear nerve can be seen at the level of the inferior colliculi.
The blood supply to the midbrain is the basilar artery and its branches (the posterior cerebral artery, the superior cerebellar artery, the posterior choroidal artery, and the interpeduncular branches).
1.17 Hindbrain
1.17.1 Pons
The pons is found below the midbrain and above the medulla (Figure 1.25). It develops from the embryonic metencephalon. The pons is the site of origin for several cranial nerves (Figure 1.26).

Figure 1.25 Sagittal section of the brain demonstrating the medulla and pons.

Figure 1.26 Cranial nerves emerging from the pons and medulla.
The pons is connected to the cerebellum via the middle cerebellar peduncles. Underlying the cerebellum is the fourth ventricle. The angle formed at the junction of the pons, the medulla, and the cerebellum is the cerebellopontine angle. The floor of the fourth ventricle reveals some anatomical landmarks including the medial eminence at the midline, the facial colliculus (containing the abducens nucleus and the facial motor fibers), and the stria medullaris (a part of the epithalamus). The pons is comprised of the ventral pons containing the pontine nuclei (responsible for coordination of movement), corticospinal and corticobulbar tracts, and the dorsal pons (tegmentum), which forms part of the reticular formation responsible for arousal and attentiveness and which contains cranial nerves and the fourth ventricle.
1.17.2 Vascular Supply
The pons is supplied by the pontine artery (basilar artery branch), the superior cerebellar artery, and the anterior inferior cerebellar artery. The pons drains to the anterior pontomesencephalic vein, and then to the basal and cerebral veins. The inferior aspects drain into the inferior petrosal sinus, which drains into the internal jugular veins.
1.18 Medulla Oblongata
The medulla oblongata contains the ascending and descending tracts and the brainstem nuclei. The inferior margin is marked by the origin of the first pair of cervical spinal nerves as the medulla exits the skull through the foramen magnum.
Several structures can be visualized on the anterior surface. These include the pyramids, the olives, and five cranial nerves (abducens nerve, accessory nerve, hypoglossal nerve, trochlear nerve, and trigeminal nerve). Posteriorly, the fasciculus gracilis, the fasciculus cuneatus, and the posterior median sulcus can be seen.
The internal structure of the medulla is usually best appreciated in cross-section and is typically discussed at the level of the pyramidal decussation, the medial lemnisci decussation (Figure 1.27), and the level of the olives.
At the level of the pyramidal decussation, we can see the descending motor fibers. The central portion contains the gray matter and the outer portion is made up of white matter, which contains the fasciculus gracilis and the fasciculus cuneatus. Corresponding portions of gray matter extend to these regions and are the nucleus gracilis and nucleus cuneatus, respectively. The spinocerebellar tracts with the lateral spinothalamic tracts in between can be found laterally, with the trigeminal nucleus found posterior to these tracts.
The decussation of the sensory pathways can be found at the level of the decussation of the medial lemniscus. The medial lemniscus is a bundle of large myelinated axons that function as second-order neurons of the dorsal column–medial lemniscus pathway to transport sensory information. The medial lemniscus is formed by the crossings of the internal arcuate fibers, which are composed of axons of nucleus gracilis and nucleus cuneatus. Centrally the hypoglossal nucleus and laterally the medial longitudinal fasciculus can be seen with the nucleus ambiguus. The medial longitudinal fasciculus controls horizontal eye movements by interconnecting oculomotor and abducens nuclei. The nucleus ambiguus contains cells bodies of motor nerves involved in swallowing and speaking. The inferior olivary nucleus (ION) is found between the nucleus ambiguus and the pyramids and coordinates signals from the spinal cord to the cerebellum. The climbing fiber axons leave the ION and travel to the cerebellar Purkinje cell.
At the level of the olives, the central canal opens into the fourth ventricle, and at this level the inferior olivary nucleus and inferior cerebellar peduncles can be seen. The vestibular nuclei can be seen in the midline. Laterally, the nucleus of the tractus solitarius (which generates peristaltic activity of the gastrointestinal system during swallowing) can be seen.
1.18.1 Vascular Supply
The vessels that supply the medulla include: the anterior spinal artery, the posterior spinal artery, the posterior inferior cerebellar artery, the anterior inferior cerebellar artery, and the vertebral artery.
1.18.2 Pathways
The ascending tracts refer to the neural pathways by which sensory information from the peripheral nerves is transmitted to the cerebral cortex. In some texts, ascending tracts are also known as somatosensory pathways.
The descending tracts are the pathways by which motor signals are sent from the brain to the lower motor neurons. The lower motor neurons then directly innervate muscles to produce movement, discussed in Chapter 7.
1.19 Cranial Nerves
There are 12 paired cranial nerves that arise from the brain. The first two, the olfactory and optic nerves, originate in the cerebrum and the rest arise in the brainstem.
1.19.1 Olfactory Nerve
This is the first and the shortest nerve. It is derived from the olfactory placode, a thickening of the neural ectoderm, which give rise to the olfactory epithelium of the nose containing the olfactory receptor neurons. These are bipolar cells that gives rise to unmyelinated axons, which are found in bundles and penetrate the cribriform plate of the ethmoid bone. They then enter the cranium and then the olfactory bulb to synapse with neurons called mitral cells, forming the synaptic glomeruli. From here, second-order neurons pass to the olfactory tract, which travels to the optic chiasm and divides into the two stria. The lateral stria carries axons to the primary olfactory cortex in the uncus of the temporal lobe and the medial stria to the anterior commissure, where they meet the olfactory bulb of the opposite side. The primary olfactory cortex sends fibers to the piriform cortex, the amygdala, the olfactory tubercle, and the secondary olfactory cortex.
The olfactory mucosa is found on the roof of the nasal cavity and is made up of pseudostratified columnar epithelium containing basal cells (which form new stem cells), sustentacular cells (for structural support, analogous to glial cells), olfactory receptor cells (bipolar cells consisting of dendrite processes with cilia that react to odors and stimulate olfactory cells), and a central process projecting in the opposite direction through the basement membrane. The mucosa also contains Bowman’s glands, which secrete mucus.
1.19.2 Optic Nerve
The optic nerve develops from the optic vesicle. It is a part of the central nervous system (CNS) and is covered by meninges. It is formed by the convergence of axons from the retinal ganglion cells, which in turn receive information from bipolar cells and the photoreceptors of the eye. Each optic nerve leaves its respective orbit via the optic canal, enters the middle cranial fossa, and unite to form the optic chiasm. Here, fibers from the nasal medial half of each retina cross to the contralateral optic tract; however, lateral fibers remain ipsilateral. Therefore, the right optic tract, for example, would contain fibers from the right temporal (lateral) retina but the left nasal retina. The optic tracts then reach the lateral geniculate nucleus (LGN) in the thalamus, which carries visual information in the optic radiation. The upper radiation carries fibers from the superior retinal quadrants (corresponding to the inferior visual field quadrants) through the parietal lobe to reach the visual cortex. The lower radiation carries fibers from the inferior retinal quadrants (corresponding to the superior visual field quadrants), through the temporal lobe, via Meyer’s loop, to reach the visual cortex for processing of visual information.
1.19.3 Oculomotor Nerve
The oculomotor nerve originates from the oculomotor nucleus at the level of the superior colliculus in the midbrain of the brainstem. It travels through the dura mater and enters the cavernous sinus. It leaves the cranium via the superior orbital fissure and divides into the superior branch to supply the superior rectus (which elevates the eyeball) and the levator palpabrae superioris (which raises the upper eyelid). It travels with sympathetic fibers that innervate the superior tarsal muscle (which helps to raise the eyelid). The inferior branch supplies the remaining extra ocular muscles (the inferior rectus depressing the eyeball, the medial rectus for adduction, and the inferior oblique for elevation, abduction, and lateral rotation). The Edinger–Westphal nucleus is dorsal to the oculomotor nuclei and contains preganglionic parasympathetic neurons to the ciliary ganglion. From here, postganglionic parasympathetic fibers supply the ciliary muscles for pupil constriction and the sphincter pupillae for accommodation.
Damage to the oculomotor nerves leads to ptosis, a down-and-out position of the eye and a dilated pupil. This can occur due to raised intracranial pressure, aneurysm of the posterior communicating artery, or damage to the cavernous sinus. It can also be found in other diseases such as multiple sclerosis and myasthenia gravis.
1.19.4 Trochlear Nerve
The trochlear nerve originates from the trochlear nuclei in the midbrain at the level of the inferior colliculus. It has the longest intracranial route because it is the only nerve to emerge from the dorsal brainstem, making it particularly vulnerable to damage. It travels within the cavernous sinus and passes through the superior orbital fissure to innervate the superior oblique muscle, which functions to depress, abduct, and intort the eye. Damage to this nerve leads to double vision and a characteristic head tilt toward the unaffected side.
1.19.5 Trigeminal Nerve
The trigeminal nerve provides sensory and motor innervation to the face. It originates from three sensory nuclei (the mesencephalic, principal sensory, and spinal nuclei) and one motor nucleus extending from the midbrain to the medulla. It has three branches, the ophthalmic, the maxillary, and the mandibular nerves, which arise from the trigeminal ganglion to provide sensory innervation to the face. The mandibular branch also supplies the muscles of mastication. Clinically, the corneal reflex can be performed to test damage to the ophthalmic nerve (which acts as the afferent limb to detect the stimulus) or the facial nerve (which is the efferent limb causing contract of the orbicularis oculi muscle).
1.19.6 Abducens Nerve
The abducens nerve is the sixth paired cranial nerve, with a somatic motor function to the lateral rectus muscle. It arises from the abducens nucleus in the pons, exiting the brainstem at the junction of the pons and the medulla. It then enters the subarachnoid space and pierces the dura mater, and enters the cavernous sinus through the superior orbital fissure. It can be damaged by any space-occupying lesion, which leads to diplopia and unopposed adduction.
1.19.7 The Facial Nerve
The facial nerve is derived from the second branchial arch. The upper motor neuron of the facial nerve is in the primary motor cortex, with its axons descending to the ventral and dorsal facial nucleus in the pons. The dorsal regions supply the muscles of the upper face and receive input from both hemispheres, and the ventral region supplies the muscles of the lower face and receives mainly contralateral inputs. The facial nerve passes through the internal auditory meatus and then on to the facial canal to synapse on the geniculate ganglion. It gives rise to the greater petrosal nerve, supplying the lacrimal gland, and joins the deep petrosal nerve to form the nerve of the pterygoid canal, innervating the pterygopalatine ganglion. It gives rise to the nerve to the stapedius muscle of the ear and the chorda tympani nerve, which supplies the submandibular ganglion and the anterior two thirds of the tongue.
The facial nerve leaves the cranium through the stylomastoid foramen and supplies the external ear, the external auditory meatus, the posterior belly of the digastric, the stylohyoid, the superior and inferior auricular, and the occipitalis muscles. It then travels to the parotid gland, and without supplying it splits into five branches (temporal, zygomatic, buccal, marginal mandibular, and cervical) to supply the muscles of facial expression.
1.19.8 Vestibulocochlear Nerve
The vestibulocochlear nerve has two main sensory divisions: the vestibular and cochlear nerves, which arise from the vestibular nuclei in the pons and medulla, and the cochlear nuclei in the inferior cerebellar peduncle, respectively. They combine at the pons and emerge at the cerebellopontine angle, exiting the cranium via the internal acoustic meatus. The vestibular nerve is responsible for equilibrium and the cochlear nerve is responsible for hearing.
The cochlea contains inner hair cells that respond to vibrations of sound and trigger action potentials from the spiral ganglia. Sound frequency is coded by the position of the activated inner hair cells.
The vestibular hair cells are found in the otoliths (the saccule and the utricule), where they detect linear motion of the head, and the semicircular canals (which detect rotational movement of the head), and coordinate balance. They are also important for the vestibulo-ocular reflex, which allows the stabilization of images on the retina while the head is moving.
Inflammation of the vestibular branch of the nerve can lead to vertigo, nystagmus, loss of equilibrium, and nausea/vomiting. Inflammation of the membranous labyrinth (labyrinthitis) can cause similar symptoms and in addition affect the cochlear nerve, leading to tinnitus.
1.19.9 Glossopharyngeal Nerve
The glossopharyngeal nerve begins in the medulla and leaves the cranium via the jugular foramen. It has mixed sensory and parasympathetic components. The sensory branch innervates the oropharynx via the pharyngeal branch, which merges with the vagus nerve to form the pharyngeal plexus. It forms the afferent limb of the gag reflex (the efferent limb is via the vagus nerve). It supplies the posterior one third of the tongue via the lingual branch and the palatine tonsils via the tonsillar plexus. It also supplies the carotid body and sinus. The tympanic branch of the nerve enters the middle ear and forms the tympanic plexus to supply the middle ear, tympanic membrane, and the eustachian tube. It supplies the stylopharyngeus muscle of the pharynx and provides parasympathetic innervation to the parotid gland.
1.19.10 Vagus Nerve
The vagus nerve extends from the medulla and exits the cranium, where it gives rise to an auricular branch and courses downward into the carotid sheath. At the base of the neck, the right vagus nerve enters the thorax anterior to the subclavian artery and the left vagus nerve passes between the left common carotid and subclavian arteries. In the neck it gives rise to pharyngeal branches (which supply the pharynx and soft palate), the external superior laryngeal nerve (which supplies the cricothyroid muscle), and the internal laryngeal branch (which supplies the laryngopharynx and part of the larynx). The right vagus nerve also gives rise to the recurrent laryngeal nerve (which supplies the intrinsic muscles of the larynx) and then forms the posterior vagal trunk in the thorax. The left vagus nerve forms the anterior vagal trunk. These contribute to the formation of the esophageal plexus innervating the smooth muscle of the esophagus. Cardiac branches to the heart arise in the thorax. The left vagus nerve gives rise to the left recurrent laryngeal nerve under the arch of aorta. The vagal trunks enter the abdomen via the esophageal hiatus in the diaphragm and then terminate into branches to supply the esophagus, the stomach, and the small and large intestines up to the splenic flexure.
1.19.11 Accessory Nerve
The accessory nerve has a somatic motor function and supplies the sternocleidomastoid and trapezius muscles. It contains spinal and cranial components. The spinal components arise from C1 to C6 spinal nerve roots, which merge and enter the cranium via the foramen magnum. After exiting the cranium, it descends to the sternocleidomastoid muscle and then to the posterior neck to supply the trapezius. The cranial component arises in the medulla and exits the cranium via the jugular foramen. It combines with the vagus nerve at the inferior ganglion.
1.19.12 Hypoglossal Nerve
The hypoglossal nerve supplies the extrinsic (genioglossus, hyoglossus, styloglossus) and intrinsic muscles of the tongue. The palatoglossus muscle is innervated by the vagus nerve. The hypoglossal nerve arises from the hypoglossal nucleus in the medulla and exits the cranium via the hypoglossal canal.
1.20 Cerebellum
The cerebellum is derived from the rhombencephalon and is found in the posterior cranial fossa. It is a large structure of the hindbrain with a protruding central vermis, which sits between the cerebellar hemispheres.
On the ventral aspect of the brain, the hemispheres are connected to the pons by the middle cerebellar peduncle. On the ventral aspect of the cerebellum is the flocculus, which is found on the cerebellopontine angle and is important for vestibular function. On each side, close to the medulla, are tonsils, and clinically these are at risk of coning into the foramen magnum when CSF is withdrawn in patients with increased intracranial pressure, resulting in pressure on the vital respiratory and autonomic centers of the medulla.
There are three main lobes of the cerebellum. The anterior lobe extends from the cerebellar peduncle and terminates at the primary fissure and continues as the posterior lobe. The smallest lobe is the flocculonodular lobe and this lies between the posterolateral fissure (inferiorly) and the cerebellar peduncles (superiorly).
1.20.1 Functional Divisions
The cerebellum can be divided into three functional areas: the cerebrocerebellum, the spinocerebellum, and the vestibulocerebellum (Figure 1.28).
The large division is the cerebrocerebellum, which is formed by the lateral hemispheres and is responsible for planning movements and motor learning. It also regulates muscle activation and visually guided movements. It receives inputs from the cerebral cortex and pontine nuclei. The fibers travel through the internal capsule and cerebral peduncles and terminate ipsilaterally in the pons and send their axons to the contralateral middle cerebellar peduncle. The cerebellum also receives information via the ascending spinocerebellar mossy fibers. Fibers from the inferior olive in the medulla form the climbing fibre input via the inferior cerebellar peduncle. They are thought to mediate plasticity in the mossy fiber–granule cell–Purkinje cell pathway to coordinate movement. Outputs from the dentate nucleus and the other deep cerebellar nuclei leave the superior cerebellar peduncle and then cross the midline at the lower midbrain, pass through the red nucleus and ascend to terminate in the thalamus. The cerebellum represents ipsilateral movement and sensation.
The spinocerebellum comprises the vermis and intermediate zone of the cerebellar hemispheres. It receives proprioceptive information and regulates body movements by allowing for error correction.
The vestibulocerebellum is the functional equivalent to the flocculonodular lobe. It controls balance and ocular reflexes, and receives inputs from the vestibular system and sends outputs back to the vestibular nuclei.
1.20.2 Deep Cerebellar Nuclei
The cortical output of the cerebellum, which is typically inhibitory, involves the deep cerebellar nuclei (Figures 1.28 and 1.29). There are three nuclei on each side: the dentate nucleus, the nucleus interpositus, and the fastigial nucleus. Some areas, such as the flocculus and the flocculonodular lobe, send outputs to the vestibular nuclei in the medulla. Together, the entire cerebellar output is transmitted to the brainstem descending motor pathways and the motor areas of the cerebral cortex via the thalamus.
1.20.3 The Cerebellar Peduncles
There are three peduncles that connect the cerebellum with the brainstem on each side. The middle peduncle is the largest and the superior peduncle is the major output pathway from the deep nuclei.
1.20.4 Cerebellar Cortex
The cerebellar cortex contains many tightly packed folds (folia). It has three well-defined layers: (i) an outer molecular layer consisting mainly of parallel fibers that run parallel to the folia and dendrites; (ii) the Purkinje cell layer containing large Purkinje cells; and (iii) a granule layer consisting of many small granule cells (Figure 1.30).
The Purkinje cells are the output cells of the cerebellar cortex. They have extensive dendrites and are intersected at right angles by the parallel fibers, allowing each Purkinje cell to receive and make synaptic contact with many parallel fibers along a single folium. The Purkinje cells send axons out of the cortex and inhibit cells in the deep cerebellar nuclei.
1.20.5 Vascular Supply
The cerebellum is supplied by the superior cerebellar, anterior inferior cerebellar, and posterior inferior cerebellar arteries. The venous supply to the cerebellum includes the superior cerebellar veins draining to the straight sinus and internal cerebral veins and inferior cerebellar veins draining to the transverse sinus, superior petrosal sinus, and occipital sinus.
1.21 Spinal Cord
The spinal cord is an organized bundle of nervous tissue extending from the medulla oblongata through the vertebral canal to the L2 vertebral level and terminates as the conus medullaris. Spinal nerves arise from the end of the spinal cord and together are known as the cauda equina. Compression of these leads to the neurosurgical emergency known as cauda equina syndrome and can lead to paralysis. The spinal cord enlarges at the level of C4–T1 (cervical enlargement) and is the site of origin of the brachial plexus. A second enlargement is at the level of T11–L1 (lumbar enlargement), and is where the lumbar and sacral plexi originate.
The spinal cord is surrounded by the spinal meninges (dura, arachnoid, and pia mater) and contains CSF. It is anchored distally to the coccyx by a fibrous band of tissue called the filum terminale. The dura mater extends from the foramen magnum to the filum terminale and is separated from the vertebral canal by the epidural space containing the internal vertebral venous plexus. The dura mater also surrounds the epineurium of spinal nerves that pierce the dura. The arachnoid mater is separated from the pia mater by the subarachnoid space and contains CSF. The pia mater surrounds the spinal cord, nerve roots, and blood vessels and inferiorly fuses with the filum terminale. The pia mater thickens to form denticulate ligaments which attach to the dura mater, and these help suspend the spinal cord in the vertebral canal.
1.21.1 Neurovascular Supply
The arterial supply to the spinal cord is via the anterior spinal artery (a branch of the vertebral artery) and the two posterior spinal arteries (branches of the vertebral artery or the posteroinferior cerebellar artery), which anastomose in the pia mater. The segmental medullary arteries, of which the largest is the anterior segmental medullary artery (the artery of Adamkiewicz), also supply the spinal cord. Disruption of the blood supply to the spinal cord leads to nerve cell death and signs of weakness, paralysis, and loss of reflexes.
The spinal cord venous drainage includes an extrinsic and an intrinsic network. The extrinsic system includes radicular veins, the pial venous network, dorsal and ventral spinal veins. They are connected to the valveless vertebral venous plexuses, that communicate with the dural venous sinuses and are composed of an external plexus surrounding the vertebral column, internal plexus within the spinal canal (linked together via segmental veins) and basivertebral veins in the vertebral bodies. The intrinsic venous system includes the sulcal (drains to the anterior spinal veins) and radial veins (drains to the posterior spinal veins), linked by the transmedullary anastomotic veins within the parenchyma of the spinal cord.
The spinal nerves originate from the spinal cord and form the peripheral nervous system. They begin as anterior (motor) and posterior (sensory) nerve roots and unite at the intervertebral foramina to form a single spinal nerve, which leaves the vertebral canal via the intervertebral foramina and divides into the posterior and anterior rami (Figure 1.31).
A peripheral nerve has an outer covering called the epineurium. Nerve fibers are organized into bundles called fascicles and each one is covered by the perineurium. Each individual neuron is covered by the endoneurium. Each individual neuron is covered by the endoneurium, a layer of delicate connective tissue around the myelin sheath of each myelinated nerve fiber in the peripheral nervous system (Figure 1.32).
A single spinal nerve innervates a strip of skin known as the dermatome and is used clinically to assess spinal injuries. During embryology the mesoderm adjacent to the neural tube (paraxial mesoderm) differentiates into 31 somites or segments. The ventral part gives rise to the ribs and vertebral column. The dorsal part consists of the dermomyotome. The dermatome forms the dermis, which eventually stretches to create a segmental innervation and the classic dermatome map (Figure 1.33).

Figure 1.33 The development of the dermatome.
1.21.2 Transverse Section of the Spinal Cord
The spinal cord contains inner gray matter surrounded by the white matter. The gray matter is divided into the dorsal horn, the intermediate column, the lateral horn, and the ventral horn. The dorsal horn contains neurons receiving somatosensory information. The ventral horn contains motor neurons that innervate skeletal muscle. The intermediate column and lateral horn contain neurons that innervate visceral and pelvic organs.
1.21.3 Rexed Laminae
The spinal cord can also be visualized in layers or laminae, where cells are grouped according to their structure and function (Figure 1.34). Lamina I contains cells that respond to noxious or thermal stimuli and sends information via the lateral spinothalamic tract. It corresponds to the marginal zone. Lamina II corresponds to the substance gelatinosa and is involved in sensation of all stimuli and moderates pain sensation. Lamina III is involved in proprioception and light touch sensation. Lamina IV is involved in non-noxious sensory information processing. Lamina V relays sensory information to the brain via the contralateral and spinothalamic tracts, and receives descending information from the brain via the corticospinal and rubrospinal tracts. Lamina VI receives proprioceptive information and sends information to the brain via ipsilateral spinocerebellar pathways and processes spinal reflexes. Lamina VII is a large region and receives information from lamina II to VI and relays motor information to the viscera. Lamina VIII is involved in modulating motor output to skeletal muscle. Lamina IX contains motor neurons innervating striated muscles and muscle spindles. Lamina X, known as the gray commissure, surrounds the central canal of the spinal cord and contains decussating axons.
1.22 Introduction to Neuroembryology
Neuroembryology is the study of the development of the nervous system during embryogenesis. The embryo develops as a zygote following fertilization and subsequently cleaves to become the morula, which reorganizes to form the blastocyst cavity (Figure 1.35). The blastocyst is comprised of two different cell types: the outer cell mass (trophoblast) and the inner cell mass (embryoblast). The trophoblast contacts with the endometrium of the uterus to facilitate implantation and the formation of the placenta.The embryoblast forms the embryo itself and is divided into the hypoblast (which becomes the yolk sac) and the epiblast (which becomes the amniotic cavity). The epiblast contains a groove in its midline called the primitive streak, which helps confer anterior–posterior and dorsal–ventral spatial information to early differentiating cells. The one-dimensional layer of epithelial cells (blastula) reorganizes into a multilayered structure, and this is known as gastrulation. The purpose of gastrulation is to form three germ layers (endoderm, mesoderm, and ectoderm) and this stage is followed by organogenesis. The endoderm develops into the linings of the respiratory and alimentary tracts, the liver, and the pancreas. The mesoderm forms the notochord, somites, and the mesenchyme. The ectoderm develops into skin, the nervous system, and neural crest cells. The neural crest cells give rise to the pharyngeal arches, which contribute to the development of the facial skeleton. Pharyngeal arches are often used interchangeably with branchial arches (Figure 1.36).
1.22.1 Neurulation (Formation of the Neural Tube)
The embryonic blastocyst develops into a two-layered structure: the epiblast and the hypoblast. In humans, the primitive streak, an opening on the epiblast midline forms ~14 days after fertilization. By the end of the third week, gastrulation occurs and the epiblast and hypoblast develop into the ectoderm and endoderm, respectively. The notochord is a rod of mesodermal cells that extends throughout the entire dorsal plane of the embryo and is responsible for inducing the overlying lateral edges of the ectoderm to form the neural plate (Figure 1.37), which will remodel to form a single closed tube. The notochord arises from a collection of cells called the Spemann–Mangold organizer. The discovery of these cells introduced the concept of induction in embryology where the identity of certain cells influences the development of surrounding cells. Later in development, the cranial and caudal portions of the neural tube form the brain and spinal cord, respectively. Closure of the neural tube is accompanied by accumulation of amniotic fluid in the central canal, which forms the primitive ventricular system the brain. The ventricular system is lined by the ventricular zone (VZ), which is an embryonic layer containing radial glial cells and is the site of neurogenesis during embryogenesis. The subventricular (SVZ) zone lies adjacent to this area, containing intermediate neuronal progenitor cells that divide into postmitotic neurons.
1.23 Regional Formations in the Brain
Once the formation of the neural tube has been completed, the neural tube continues to differentiate to form different regions of the brain. These regions are the prosencephalon (forebrain), the mesencephalon (midbrain), the rhombencephalon (hindbrain), and the spinal cord (Figure 1.38).
The proper formation of these regions requires the presence of inducing morphogens. The development of the midbrain and hindbrain requires the isthmus organizer, which releases signals that organize the expression of transcription factors.
The forebrain divides into the telencephalon and the diencephalon. The telencephalon includes the olfactory bulbs, hippocampus, and cerebrum. The diencephalon includes the optic nerves, epithalamus, thalamus, and hypothalamus.
The developing hindbrain divides into the metencephalon and the myelencephalon. Coordinating and fine-tuning muscle movements are functions primarily found in the hindbrain, because the cerebellum and pons derive from the metencephalon. The myelencephalon develops the medulla, which relays vital information regarding breathing and heart rate to the brain from the rest of the body and is responsible for involuntary reflex movements, such as sneezing and swallowing.
Three membranous layers cover the CNS: the dura mater (derived from the surrounding mesenchyme), the arachnoid, and the pia mater (both derived from the neural crest cells).
1.24 Neurogenesis
Embryonic neurogenesis refers to the generation of neurons from neural stem cells or neuron progenitor cells. Adult neurogenesis occurs in specialized niches of the brain throughout life, namely in the SVZ of the lateral ventricles and in the subgranular zone (SGZ) of the hippocampal dentate gyrus.
The VZ of the neural tube contains neural precursor cells (NPCs), which become radial glial cells (RGCs). The RGCs oscillate between the basal and apical VZs. They proliferate and symmetrically divide during the cell cycle. This process is known as interkinetic nuclear migration. Progenitor cells also accumulate in the SVZ and continue to expand to give rise to excitatory pyramidal projection neurons and glial cells. They switch to asymmetric cell division and become a postmitotic neuron, which migrates and forms the neocortex or becomes an intermediate progenitor.
RGCs give rise to a six-layered cortex. The earliest neurons become inner layer 6 and the last-born neurons give rise to layer 2. The marginal zone is the external layer (layer 1). RGCs also generate oligodendrocyte and astrocyte progenitors. The oligodendrocytes produce myelin sheath in the CNS. Astrocytes become the most abundant cells in the brain and have several functions including maintenance of the blood–brain barrier (BBB) and metabolism.
There are several transcription factors that play an important role in regulating neurogenesis. The Paired box protein (Pax-6), is involved in neural stem cell proliferation, neuronal specification and migration in the CNS. The Sox family of proteins also have an important role in neuronal fate commitment and differentiation.
Programmed cell death is an evolutionarily conserved trait that is an integral part of the development of the nervous system. It is postulated that up to half of the original neuronal cell population is eliminated as a result of apoptosis. The elimination of superfluous neurons during development is critical for the optimization of synaptic connections, often referred to as the nerve growth factor theory- the idea that targets of innervation supply limiting amounts of neurotrophic factors, which the developing neurons depend on for their survival.
1.25 Neuronal Migration
Neurons can migrate to their target location via radial migration along radial glia fibers in a perpendicular fashion to the ventricular surface. Glutamatergic neurons are made in the VZ and travel to their target zones via radial migration. Radial migration of neurons occurs in several distinct modes, and these include locomotion, somal translocation, and via multipolar migration. Neurons can also migrate to their target location via tangential migration, which involves migration in trajectories parallel to the ventricular surface and orthogonal to the radial glia fibers. Inhibitory GABAergic cortical interneurons travel via tangential migration to the cerebral cortex. Neurons from distinct sites converge and form neural circuits via tangential migration.
1.26 Axon Guidance
The formation of networks following cell division and migration is crucial to normal brain development. The first stage of development of a network is the formation of axonal tracts. Axons project and reach their target through a series of coordinated steps. Axons grow from a region within their terminal called the growth cone.
The growth cone possesses expansions at the tip called lamellipodia and fine processes called filopodia that help move the axon in a certain direction in response to axon cues or guidance molecules such as netrin, semaphorin, Slit–Robo, and Notch. The netrins are large, soluble proteins produced by the VZ and floor plate, which can function as both attractants (via interaction with the Netrin receptor DCC, also known as colorectal cancer suppressor), and repellants via the Slit proteins, which bind to receptors from the round- about (Robo1 - Robo4) family. Midline cells express Slit, thereby controlling axon crossing at the midline and preventing recrossing of axons that have already crossed by interacting with the Robo receptors.
Pioneer axons are the first to grow in a defined region. They are not morphologically different from other axons; however, they facilitate the formation of a navigation path for follower axons. Pioneer axons and follower axons can fasciculate to form a nerve tract. Pioneer axons are aided by guidepost cells, which are specialized populations of neurons or glia that express guidance cues to enable the growth cone to navigate to its destination in a series of short, sequential segments.
1.27 Synapse Formation
The next step in the development process is to form synapses. A growth cone will transform to become a bulbous presynaptic site. This is followed by a rapid increase in intracellular calcium (Ca2+) ion concentration, which causes the shape of the growth cone to change. Various adhesion molecules and factors further contribute to the shape of the cone, and finally the first contact with the postsynaptic membrane is made. Synapse formation is finely regulated and the presynaptic region becomes highly specialized architecturally to allow regulated secretion of neurotransmitters. The postsynaptic region is characterized by clustering of receptors opposite the presynaptic active zone, to allow for efficient transfer of information.. There may also be multiple receptors that bind to the same neurotransmitters, such as GABAA and GABAB receptors, both binding to GABA.
The location of the synapse and its properties will impact the flow of information in the circuit. The synapses formed may be of a specific type – e.g., glutamatergic neurons – and they may also form on specific regions, such as soma or dendritic spines. Those located near the soma or the initial segment of the axons have a greater influence on the firing properties of the action potential. Those in distal dendrites are smaller in nature and must summate to depolarize the membrane.
Synapses also show specificity and can be governed by many factors, such as the Sonic Hedgehog cues expressed by postsynaptic neurons and its receptor Brother of CDO (Boc) in the presynaptic neurons, which aid in the targeted formation of the synapses.
Synaptic maturation is a slower process that continues until the end of adolescence and is designed to refine synaptic connections by elimination and by allowing competition between different inputs. The importance of sensory experience on synapse elimination and stabilization was demonstrated in the classic visual deprivation experiments by Hubel and Wiesel (see section on Ocular Dominance).
1.28 Brain Plasticity
The ability of the nervous system to change structurally and functionally due to various environmental factors is known as plasticity. Structural plasticity includes changes in the cellular architecture and functional plasticity includes changes in function due to changes in the structural components.
Synaptic plasticity includes changes in strength and transmission due to experience or input changes and is particularly pronounced during critical periods of development. Network changes are modifications of neuronal networks composed of linked neuronal populations.
Repetitive firing of adjacent excitatory neurons is known to strengthen synaptic connections or weaken with the firing of inhibitory neurons (Hebb’s postulate). This also forms the basis for LTP and LTD models of synaptic plasticity. Spike-timing–dependent plasticity refers to the frequency of presynaptic firing and the order of pre- and postsynaptic firing during a precise time, and this affects the strength of synaptic plasticity. However, some synapses do not follow Hebb’s postulate and their strength may be influenced by other factors, such as dendritic depolarization or changes in sensory input. The pruning and formation of synapses leads to various changes, such as spine morphology and neurotransmitter release, with a resultant change in the strength of synaptic connections leading to the modification of neural networks.
Homeostatic plasticity, a major form of non-Hebbian plasticity, refers to cellular and molecular mechanisms that limit the unopposed persistence of activity-dependent synaptic plasticity, which has the inherent risk of inducing extreme neural states and therefore counters the self-reinforcing nature of Hebbian plasticity. It allows neural circuits to achieve functional stability by e.g. equipoise of intrinsic excitability and synaptic strength. Metaplasticity is a higher-order form of synaptic plasticity, and is known as the plasticity of synaptic plasticity. This refers to modulation of synaptic plasticity based on activation history in the same synapse (homo- synaptic metaplasticity) or nearby synapses (heterosy- naptic metaplasticity).
1.29 Critical Period Versus Sensitive Periods
During a critical period, the presence of an experience is needed for the development of a specific circuit. During a sensitive period, sensory experience influences development, but this is not exclusive to a set period. Sensitive periods are important for cognitive abilities, such as learning a language.
1.30 Critical Periods of Brain Development Are Windows of Heightened Plasticity
Critical periods during early brain development are epochs of heightened plasticity, in which sensory experience is required to establish optical cortical representations of the environment. The onset of critical periods is regulated by the balance of excitatory and inhibitory connections. The leading contributor to critical opening in the visual cortex is an increase in GABAergic inhibition, reportedly dependent on brain–derived neurotrophic factor (BDNF) levels. Closure of the critical period is important to ensure that there is sequential consolidation and retention of new and complex perceptual, cognitive, and motor functions. However, if sensory experience is abnormal during this period, there may be permanent detrimental effects. If the abnormal experience occurs after the period has closed, there will be reversible, and less detrimental effects on the brain.
1.31 Critical Periods of Sensory Systems
1.31.1 Ocular Dominance
The neurons in the primary visual cortex of mammals contain monocular neurons in layer 4 and binocular neurons in the remaining layers. Neurons with the same eye preference are grouped together in ocular dominance (OD) columns. In the classic experiments by Reference TN and HubelHubel and Wiesel (1963), it was demonstrated that monocular deprivation of one eye in kittens during a critical period abolishes the ability of that eye to respond to visual stimulation and shifts the OD columns in favor of the open eye. However, a similar experiment in adult cats had no detrimental effect on vision. In humans, monocular deprivation from conditions such as strabismus can have the same effect, known as amblyopia, and can also lead to problems with depth perception.
1.31.2 Auditory Processing
Neurons in the primary auditory cortex (A1) are topographically arranged by their response to different frequencies. Organization of these tonotopic maps are genetically predetermined but refined in an activity dependent manner, through spontaneous activity and, following hearing onset. During critical periods, heightened plasticity drives maturation of specific features of the auditory system, such as identification of phonemes and language acquisition. However, this period is also exquisitely sensitive to impoverished environmental influences, which may have inadvertent effects on frequency tuning. Similarly, overexposure to pure tones may result in overrepresentation of the tone frequency within the map. Critical periods have also been described for other processes such as binaural hearing, which allows the localization of different sound sources. This can be greatly affected in childhood hearing loss associated with ear infections, with overrepresentation of stimuli to the unaffected ear and weakening of responses to the affected ear, resulting in reduced ability to navigate the auditory scene.
1.32 Plasticity Regulators
As external sensory inputs increase, there is increased cortical activity which guides neural circuit development. This stage is aided by neurotrophic factors that promote plastic changes and simulate neuron growth. However, as inhibitory circuits mature further, these factors decline in number and plasticity regulators prevent further circuit changes to ensure that the cortical representations that have been developed are stable and will not be modified further by passive abnormal sensory inputs. There are several plasticity regulators that are established during the closure of the critical period. These molecular brakes include perineuronal nets (envelops inhibitory neurons) and chemorepulsive cues (restricts axon growth). Axonal growth can be impeded from the binding of axonal growth inhibitors such as Nogo to myelin receptors. There are several plasticity regulators that work in synergy to regulate cortical excitatory/inhibitory (E/I) balance. A high E/I ratio during development is important for remodeling of cortical circuits. However, a high inhibitory tone is important for maintaining stable cortical representations throughout the lifespan. Evidence for this comes from conditions characterized by a downregulation of inhibition, which is associated with impaired sensory processing. During natural aging, there is a reduction in GABA concentration, and this contributes to reduced sensory processing and impaired learning.
Critical period plasticity can be modulated by reopening critical windows in the adult brain or through the premature closure of periods of abnormal plasticity in the developing brain. Approaches that may be used to reset neural networks include for example, the removal of perineuronal nets or manipulation of inhibitory GABAergic circuits.
1.33 Introduction to Comparative Neuroanatomy and Animal Models
Our understanding of the basic function and structure of the nervous system comes largely from studies on a range of species (Figure 1.39). The benefits of comparative neurobiological studies have enabled the discovery of several well-known concepts, such as the squid giant axon and the ionic basis of the action potential by Hodgkin and Huxley, discovery of dendritic spines in the CNS of the chicken by Cajal, and understanding the cellular basic of learning and memory in Aplysia by Kandel, to name a few. The examination of the CNS of different species allows us to discern features that are not easily seen in humans. In this section, we discuss the most common species used for neurobiological research.
1.33.1 Rodents
There are several anatomical differences between human brains and those of rodents. Firstly, it is thought that humans have approximately 86 billion neurons compared to approximately 70 million in mice. The human brain cortex is folded, which increases its surface area, whereas the rodent cortex is smoother. Humans also have highly specialized regions for language and cognition. Rodents, however, have more evolved olfactory bulbs, necessary for their superior sense of smell.
Rats were historically the most popular animals used for neurobiological research due to their genetic and physiological similarities to humans. They are also larger in size than mice and therefore easier to handle and perform procedures on. However, the use of this model has declined largely due to the difficulties in directed manipulation of the rat genome.
Mice are formidable models for research in neuroscience. The ability to manipulate the mouse genome has allowed us to decipher the functions of genes and their role in disease processes. There are several advantages of using mice as models. They are small in size, allowing novel compounds to be delivered in smaller quantities for testing. They are also relatively cheap to maintain and reproduce quickly. They can also be inbred to yield identical strains, which removes genetic heterogeneity. The gene regulation networks are largely conserved in mice and human, however there are striking differences in gene expression patterns, and equally there are notable differences in immune responses, stress responses and metabolism between mice and humans. This often leads to disappointing results from clinical trials that are spurred on by positive results found in mouse studies. Rodent use in various physiological processes and diseases has been well described in the literature and the reader is directed to these sources for further reading.
1.33.2 Non-Human Primates
The use of non-human primates for basic neuroscience research has provided great insight into brain function and has allowed the testing of several therapeutic strategies used in human disease. Although there are many similarities between the human brain and the primate brain, there are notable anatomical differences. The human brain is 4.8 times bigger in size than it is for a comparable monkey (Figure 1.37). Human neocortex expansion has likely contributed to the higher cognitive abilities of humans. The prefrontal cortex forms a larger part of the human brain with a greater density of neurons compared to macaque brains. Other notable differences include a much smaller or absent fasciculus arcuatus (important for language processing) in non human primates. The consequence of an increase in size means that there is an increase in the number of specialized subregions such as the parietal cortex.
1.33.2.1 Macaque Monkey Model
The most frequently used non-human primates used in brain research are the long-tailed macaque (Macaca fascicularis) and the rhesus macaque (M. mulatta). Marmosets, squirrel monkeys, and cebus monkeys have also been used, although less frequently.
For ethical reasons, the human brain cannot be easily manipulated. If a particular phenomenon of brain function cannot be studied in other animals, macaques provide a suitable alternative as they are biologically similar to humans. Macaques can be used to systematically investigate the activity of individual neurons and their relation to higher cognition.
The use of deep brain stimulation (DBS) for intractable Parkinson’s disease has been mastered in rhesus macaques. It involves the implantation of electrodes in specific regions in the brain, which are then connected to an external neurostimulator. DBS applies intermittent electrical current to the target with higher frequencies than normal firing rates in that region. The most common targets are the STN and the internal globus pallidus and sometimes the ventral intermediate thalamus. The internal globus pallidus is a favored target for gait disturbances, problems with word fluency, and axial symptoms or troublesome dyskinesias. The STN is preferred in patients with higher medication requirements.
Long-tailed macaques have also been used to study Alzheimer’s disease as they naturally develop protein deposits in the brain, similar to that in humans. Genetically modified macaques with a mutation of the Huntingtin protein can result in symptoms and signs of Huntington’s patients similar to humans.
Brain–machine interfaces (BMIs) are new strategies aimed at restoring mobility in severely paralyzed patients and have been tested in macaques. They involve the insertion of implants into the cortex connected to an electrical stimulator on the spine of a monkey with induced spinal cord injury. The implant records the neuronal activity of regions involved in motor control, interfaced with electrical stimulation in the epidural region of the spinal cord. A computer can recognize the animal’s intention to walk and then send signals to the stimulator. This subsequently activates neural circuits in the spinal cord that control the muscle activity involved in walking. The brain–spine interface is therefore able to restore locomotion of the paralyzed leg and has been useful for proof-of-concept studies in humans.
1.33.3 Drosophila
The use of Drosophila (fruit flies) has been instrumental in furthering advances in neuroscience, with many milestone discoveries made. The advantages and limitations of this model are shown in Table 1.1.
Table 1.1 Advantages and limitations of the Drosophila model
Advantage | Disadvantage |
---|---|
Limited ethical concerns | Major differences in systems compared to humans limiting translation research e.g. lack of an adaptive immune system. |
Ease of genetic manipulation | Difficulty in assessing complex behaviors. |
Genetic manipulation is fast and inexpensive (3 months, <$500 per transgene) | Only basic measures of cognitive decline |
Plethora of available resources/stocks (e.g., genome-wide RNAi-library) | Need to maintain large breeding stocks due to inability to cryobiologically preserve flies |
Short generation time | Less-complex and adaptive immune system than in vertebrates |
Fully sequenced and annotated genome | Effects of drugs on the organism might differ (e.g., conversion of pro-toxins to toxins in liver) |
Conservation of basic signaling pathways and cellular processes | Fly lines are subject to genetic drift via random mutations complicating genetic research of disease pathways |
The availability of balancer chromosomes allowing the maintenance of lethal mutations |
The Drosophila brain contains several discrete structures. These include the antennal lobes, important for the olfactory chemosensory pathway, and the mushroom bodies that are involved in olfactory associative learning and memory. A prominent structure in the central brain is the central body complex, which consists of four substructures: the fan-shaped body, the ellipsoid body, the paired nodule, and the protocerebral bridge. The central complex is involved in learning and memory, locomotor control, and courting behavior. The visual neuropils in the Drosophila form the optic lobe, which consists of four major substructures: the lamina, medulla, lobula, and the lobula plate. The subesophageal ganglion controls the mouth, salivary glands, and neck muscles (Figure 1.39).
Initial use of Drosophila for research was aimed at understanding the principles of heredity. Early studies of this model led to the discovery of balancer chromosomes, allowing the maintenance of mutations in essential genes without the need to genotype the animal for further breeding. Early demonstrations of how x-ray exposure can lead to genetic mutations, with resultant change in the genome, were conducted in Drosophila. The discovery of mitotic recombinations, where it was first demonstrated that reciprocal genetic exchange between chromosomes occurred outside the germlines, was first seen in Drosophila.
The importance of various genes in nervous system development, segmentation, and pattern formation were discovered using x-ray–induced mutations. The bithorax complex of genes and the polycomb gene were shown to be important in segmentation in the fruit flies.
Key genes involved in embryonic pattern formation and nervous system development such as Notch were first identified in Drosophila. The loss of Notch leads to the formation of an embryo with hypertrophied regions of the CNS and an underdeveloped ventral hypoderm, leading to premature differentiation into neuroblasts. Components of its pathway such as neuralized are also important in learning and memory.
Larval Drosophila were among the first species to be used as a model for synaptic transmission through the study of their neuromuscular junction (NMJ). Presynaptic and postsynaptic genetic manipulation techniques were used to discover the functions of many proteins, including the roles of synaptotagmin as a calcium sensor.
1.33.4 Zebrafish
The zebrafish has become an instrumental model in answering fundamental questions about the brain and has recently seen many sophisticated applications in investigating clinical diseases. The zebrafish contains many structures that are similar to other vertebrates. Approximately 6 hours post fertilization (hpf), the CNS begins to develop. By 24 hpf, the brain can be distinguished into the forebrain, midbrain, and hindbrain (Figure 1.40). The forebrain further differentiates into the telencephalon, the diencephalon, the hypothalamus, and the retina.

Figure 1.40 Zebrafish developmental timeline.
The telencephalon in zebrafish is composed of the subpallium (ventral telencephalon), the pallium (dorsal telencephalon), and the olfactory bulb. The subpallium in the zebrafish serves a similar function as the mammalian basal ganglia. The ventral telencephalon contains the ventral nucleus and a dorsal nucleus. GABAergic and cholinergic neurons are formed in this region. The dorsal telencephalon is composed of a more complex array of regions including the central, lateral, posterior, and dorsomedial zones. It serves a similar function to that of the hippocampus and amygdala in mammals. The olfactory bulb of the telencephalon receives odor information.
The zebrafish diencephalon is composed of the thalamus, pineal body, and habenula. The habenula connects the forebrain with the midbrain and hindbrain, and is involved in fear modulation, reproductive behavior, and sleep initiation.
The hindbrain is easily visualized in the zebrafish. Numerous motor neurons and those that innervate the branchial arches and the cerebellum originate here. The hindbrain contains reticulospinal neurons including the Mauthner cell, which mediates escape responses.
The zebrafish has several advantages as an animal model, including small size and low maintenance costs. Zebrafish larvae have further advantages in being easily manipulated, permeable to small molecules, rapidly generated, optically translucent, and having non-protected status prior to 5 days post fertilization (dpf). The zebrafish genes are thought to share approximately 70% homology with that of human genes and has high homology to mammalian biology. The zebrafish possesses conserved neural circuits underlying basic behaviors and pathways and is therefore likely to be relevant for translational research. Neurons are accessible during in vivo imaging and can therefore be used to investigate how neuronal circuits are involved in complex behavior.
The behavioral repertoire of zebrafish larvae has been exploited to not only understand the functions of distinct regions of the brain but also to test various neuropharmacologic agents. Zebrafish have a developmentally regulated BBB, which is functional at 10 dpf and is therefore ideal for testing drugs. Behavior that is commonly tested in zebrafish includes thigmotaxis (the tendency to move toward the periphery of an environment) for testing anxiety, startle responses to various stimuli, and optomotor responses, an innate visuomotor reflex, initiated by neurons in the pretectum/tectum or through the activation of hindbrain integrator neurons which activate downstream motor commands. Locomotion play an integral role in the feeding, social, and defensive activities of the zebrafish and is produced by reticulospinal neurons of the brainstem along with descending vestibulospinal or neuromodulatory projections. The sleep/wake pattern of the zebrafish is similar to humans and provides great insight into the biology of the circadian rhythms and can be used as a model to study human sleep. Other behavior paradigm tests include habituation (to test non associative learning) and prey capture (decision making and cognition).
The zebrafish has also been used to model various clinical diseases. These include neurodegenerative diseases such as Alzheimer’s disease, Parkinson’s disease, and Huntington’s disease. It has also been used to model brain tumors (Figure 1.41), intracerebral hemorrhage, and arteriovenous malformations.

Figure 1.41 Zebrafish larvae at 5dpf showing glioblastoma tumor growth (green)
The use of zebrafish for translational research is still at an infantile stage for many diseases and therefore requires rigorous testing and exploration. For example, although it is exceptional for large scale pharmacologic testing, the route of administration of drugs in zebrafish includes passive exposure in water rather than active administration and therefore may produce differing results in humans.
1.33.5 Caenorhabditis elegans
Caenorhabditis elegans is a nematode approximately 1 mm in size. It is a formidable organism for the study of genomes that may otherwise be difficult in mammalian systems. As an animal model it has several advantages, such as a transparent body, a short life cycle, and fecundity. Furthermore, as they are self-fertilizing hermaphrodites, each progeny represents a genetic clone.
The C. elegans lineage is invariant and the complete cell lineage for the 959 cells in the adult has been described. The sequencing of the genome has also demonstrated that approximately 38% of worm genes have a human ortholog and therefore provides opportunity for it to be used as an in vivo model for the study of certain human conditions. It also has a compact genome size and therefore genetic screens can be conducted to further elucidate the molecular underpinnings of certain behaviors.
The complete connectome of C. elegans contains 302 neurons for the adult hermaphrodite. It has been used to further our understanding of well-known signaling pathways and study the complexities of the nervous system.
It has been particularly useful for studying memory and learning. These organisms have an ability to quickly make decisions, and this is observed when presented with harmful substances; however, when this is simultaneously presented with attractive odorants, it decides whether to migrate to the attractive odorant or avoid it due to its proximity to the harmful substance. It also possesses long-term memory as evidenced by its enhanced attractive responses to certain odors as adults if it was previously exposed to this at the larval stage.
1.33.6 Hydra
Hydra was discovered at the beginning of the eighteenth century. Since then, it has been used as an animal model to improve our understanding of fundamental processes in animal biology (Figure 1.42). In 1744, Trembley published his Mémoires, which provided the first description of asexual reproduction by budding, the first controlled experiments in animals on regeneration, the first successful animal grafts, the first study of phototaxis in animals without eyes, and the first vital staining of tissues. Later, Ethel Browne Harvey used Hydra in a series of experiments that introduced a new concept in developmental biology, known as the organizer phenomenon. More specifically, she showed that when grafting the tentacle of one Hydra onto the body column of another Hydra, one can trigger the growth of a new Hydra at the grafting site. This new Hydra would develop normally as if it had been the result of budding or asexual reproduction.

Figure 1.42 Gross structure of the Hydra.
Hydra has been studied for more than 300 years, mostly because of how easy it is to culture and manipulate in a laboratory. Hydra can grow and shrink by an order of magnitude (from 1 mm to 1 cm) depending on various factors such as food availability and environmental temperature. Consequently, its nervous system is composed of a variable number of neurons from a few hundred to a few thousand depending on the size of the animal. Traditionally, two main types of neurons have been reported: sensory cells, exposed to the external or gastric environment, and ganglion cells, which form a two-dimensional lattice known as a nerve net. Moreover, the nerve net of Hydra is made up of two separate components: the endodermal nerve net and the ectodermal nerve net. The morphology of both sensory cells and ganglion cells can vary in terms of the size of their cell body and the ramification of their neurites.
The behavioral repertoire of Hydra is quite limited and includes basic behaviors such as periodic contractions of the body column on the longitudinal axis and along its radius, photic response, and feeding.
Establishing links between neuronal activity, anatomy, and behavior in Hydra has been challenging because of technical limitations. When it comes to electrophysiology, single-cell recording approaches such as sharp electrode recordings are not possible because Hydra neurons are small and spread out. On the other hand, with extracellular recordings (e.g., using suction electrodes) it is difficult to precisely localize their source. For these reasons it has not been possible until recently to measure how many networks exist in the nervous system of Hydra and in what behavior each of them participates.
The application of modern methods in molecular biology to Hydra has the potential to greatly advance our understanding of its nerve net. The sequencing of the Hydra genome makes it possible to study its surprisingly rich repertoire. Indeed, despite its basal metazoan lineage, the Hydra genome comprises more than 20,000 genes including a large number of neuronal genes such as sodium, potassium, and calcium channels, and receptors for glutamate, GABA, dopamine, serotonin and a vast array of neuropeptides. Also, methods for creating stable transgenic lines have been developed, enabling the use of a large range of modern molecular tools. Among these tools, genetically encoded calcium indicators are particularly well suited to study the activity of the nervous system of Hydra for multiple reasons as they are small, transparent, and contain neurons that are widely scattered.
Figure acknowledgements
The following images were created using a Biorender industrial license: Figure 1.1, 1.4, 1.7, 1.11, 1.15, 1.20, 1.22, 1.24, 1.27, 1.29, 1.39-1.40
The following images were created using a shutterstock license: Figures 1.2–1.3, 1.5–1.6, 1.8, 1.9–1.10, 1.12–1.14, 1.16–1.18, 1.23, 1.25–1.26, 1.28, 1.30–1.38
The following images are credited to the authors of this chapter: Figure 1.19, 1.21, 1.41–1.42