Introduction
The breathing of dry air aggravates the severity of respiratory diseases ranging from asthma and COPD to infections such as influenza and COVID-19 (Ghosh et al., Reference Ghosh, Boucher and Tarran2015; Mecenas et al., Reference Mecenas, Bastos and Normando2020; Romaszko-Wojtowicz et al., Reference Romaszko-Wojtowicz, Cymes, Dragańska, Doboszyńska, Romaszko and Glińska-Lewczuk2020; Moriyama et al., Reference Moriyama, Hugentobler and Iwasaki2021). Dehydrated airways promote inflammation (Barbet et al., Reference Barbet, Chauveau, Labbe and Lockhart1988), mucus production (D’Amato et al., Reference D’Amato, Molino, Calabrese, Cecchi, Annesi-Maesano and D’Amato2018), cilia dysfunction (Ghosh et al., Reference Ghosh, Boucher and Tarran2015; D’Amato et al., Reference D’Amato, Molino, Calabrese, Cecchi, Annesi-Maesano and D’Amato2018; Moriyama et al., Reference Moriyama, Hugentobler and Iwasaki2021) and triggers of cough (Purokivi et al., Reference Purokivi, Koskela and Brannan2011; Zanasi and Dal Negro, Reference Zanasi and Dal Negro2022), notably adenosine triphosphate (ATP) release (Button et al., Reference Button, Okada, Frederick, Thelin and Boucher2013) and associated acidification of the airways (Zajac et al., Reference Zajac, Dreano, Edwards, Planelles and Sermet-Gaudelus2021). By these phenomena, and the associated reduction of mucociliary clearance of inhaled particles (Ghosh et al., Reference Ghosh, Boucher and Tarran2015; D’Amato et al., Reference D’Amato, Molino, Calabrese, Cecchi, Annesi-Maesano and D’Amato2018; Mecenas et al., Reference Mecenas, Bastos and Normando2020), dry upper airways amplify the health risks of polluted air (Edwards et al., Reference Edwards, Norden, Karnath, Yaghi, Roy, Johanson, Ott, Brownstein, Grove, Tomson and Friberg2021).
Non-communicable respiratory diseases such as asthma and chronic obstructive pulmonary disease are an increasing problem at both ends of the age range in low- and middle-income countries (Troeger et al., Reference Troeger, Blacker and Khalil2018). Children are especially vulnerable to respiratory disease, as indicated by asthma attacks in the United States among children relative to the general population (Pate et al., Reference Pate, Zahran, Qin, Johnson, Hummelman and Malilay2021). Among U.S. children, who also tend to suffer from dehydration (Brooks et al., Reference Brooks, Gortmaker, Long, Cradock and Kenney2017), Black and non-Black Hispanic children are most at risk of asthma, with Black children three to four times more likely to die of asthma than non-Black children (Forno et al., Reference Forno2009). Children of colour are also most dehydrated among all U.S. children (Brooks et al., Reference Brooks, Gortmaker, Long, Cradock and Kenney2017).
By their proximity to external air, the upper airways are more prone to dehydration than the lower airways, and dehydrate in many natural ways (Wolkoff, Reference Wolkoff2018), beyond whole-body dehydration. Mouth breathing (Svensson et al., Reference Svensson, Olin and Hellgren2006), heavy breathing as occurs on sustained exercise (Karamaoun et al., Reference Karamaoun, Haut, Blain, Bernard, Daussin, Dekerle, Bougault and Mauroy2022) and cold dry inhaled air (D’Amato et al., Reference D’Amato, Molino, Calabrese, Cecchi, Annesi-Maesano and D’Amato2018) all contribute to drying out of the upper airways. Dehydration of the larynx is a special threat, given that it is the site of fastest air flow within the airways on inhalation (Sivasankar and Fisher, Reference Sivasankar and Fisher2002). Laryngeal dysfunction associated with cough (Vertigan et al., Reference Vertigan, Kapela, Kearney and Gibson2018) has consequently long been associated with the breathing of dry air (Purokivi et al., Reference Purokivi, Koskela and Brannan2011; D’Amato et al., Reference D’Amato, Molino, Calabrese, Cecchi, Annesi-Maesano and D’Amato2018; Zanasi and Dal Negro, Reference Zanasi and Dal Negro2022). Increased responsiveness of laryngeal protective reflexes triggered by mechanical or chemical stimuli characterises cough hypersensitivity syndrome (Chung et al., Reference Chung, McGarvey, Song, Chang, Lai and Canning2022), reflected by an increased sensitivity to tussive stimuli and mediated through a neuropathic process associated with neural inflammation of the vagal sensory neurons in the laryngeal area and in the airway submucosa (Chung et al., Reference Chung, McGarvey and Mazzone2013). Cough and laryngeal hypersensitivity are often associated with airway conditions such as asthma and rhinosinusitis, and with gastro-oesophageal reflux (Bucca et al., Reference Bucca, Bugiani, Culla, Guida, Heffler and Mietta2011), while also often observed in athletes (Boulet and Turmel, Reference Boulet and Turmel2019), where chronic dehydration of the airways occurs on account of the high ventilation of exercise, generally through the mouth.
One of the primary roles of the upper airways is to humidify inhaled air (Fronius et al., Reference Fronius, Clauss and Althaus2012). Humidification of inhaled air necessitates a steady draw of water to the air/water surface from within the airway lining fluid (ALF), from tissues surrounding the airways and from moisture that is condensed on the ALF during exhalation. Water transport within and around the ALF occurs by the establishment of osmotic pressure differences above and below a mucus layer, and across the airway epithelium between the periciliary layer (PCL), within which cilia protrude from ciliated epithelial cells, and surrounding tissue (Tilley et al., Reference Tilley, Walters, Shaykhiev and Crystal2015; Bustamante-Marin and Ostrowski, Reference Bustamante-Marin and Ostrowski2017).
The osmotic movement of water through the mucus, a hydrogel (Song et al., Reference Song, Cahn and Duncan2020), is common to transpiring hydrogels in nature (Etzold et al., Reference Etzold, Linden and Worster2021), as on the surfaces of plant leaves (Wheeler and Stroock, Reference Wheeler and Stroock2008), where osmotic pressures (Shultze, Reference Shultze2017) can be sufficiently large to pull water against gravity across large vertical distances (Wheeler and Stroock, Reference Wheeler and Stroock2008). The facility of water to transport across hydrogels is characterised by a physicochemical (continuum) transport property of the hydrogel called the water permeability (Nishiyama and Yokoyama, Reference Nishiyama and Yokoyama2017), which increases and decreases with various natural phenomena, such as dehydration (which tends to shrink the hydrogel) (Vyazmin et al., Reference Vyazmin, Khramtsov, Pokusaev, Nekrasov and Zakharov2019). Shrinking and expansion of hydrogels, depending on their ionic nature (anionic or cationic), also occur with acidification (Tomar et al., Reference Tomar, Tyagi, Choonara, Kumar and Pillay2014), and alterations in ion content type and concentration (Tomar et al., Reference Tomar, Tyagi, Choonara, Kumar and Pillay2014).
Condensation layers inevitably exist over transpiring hydrogels (Vyazmin et al., Reference Vyazmin, Khramtsov, Pokusaev, Nekrasov and Zakharov2019) as can be illustrated by way of a simple example: Imagine a mucus-like hydrogel in contact with air and containing salt ions as well as deposited airborne particles, many of which are small enough to diffuse through the hydrogel pores, and others that are too large. The salt ions, fine and ultrafine particles diffuse with slight to moderate restricted motion, while large particle diffusion is restricted or prevented altogether. Moisture in the air condenses onto the hydrogel surface through natural condensation, as in the sudden sweep of super-saturated air as occurs on an exhalation. This leads to deposition of a mass of water on the surface of the hydrogel, and immediate diffusion of salt ions and small particles from the hydrogel into the condensation layer. An equal and opposite mass of water flows into the membrane. Once enough salt and ultrafine particles have diffused into the condensation layer to eliminate concentration gradients, and an equivalent mass of water has moved by osmosis into the hydrogel, solute concentration in the condensation layer will be the same as in the pores of the hydrogel. On evaporation, the mass of water in the layer diminishes, moving the surface of the condensation layer towards the mucus. Since the salt ions and small particles are partially restricted in their movement into the pores of the hydrogel, their concentration increases above the hydrogel, driving diffusion into the pores, and the reverse movement of water into the condensation layer, the basic physics of osmosis. To supply water at sufficient rate to meet the rate of evaporation, very large osmotic pressures can arise – osmotic pressure exceeding 5,000 atmospheres have been observed in natural hydrogels during evaporation in 40% RH air (Wheeler and Stroock, Reference Wheeler and Stroock2008). Condensation layers of this kind would appear to play a role in the function (humidification and filtration of inhaled air) and dysfunction (cough, clearance breakdown, vocal dysphonia, among others) of upper ALF, and relate to the rehydration efficacy of inhaled hypertonic salines (see Fig. 1).

Fig. 1. (a) Basic one-dimensional airway-lining-fluid geometry, steady-state, time-averaged water fluxes, and osmotic mucus force consequent to the encounter with relatively dry air. The flux of water through the mucus hydrogel (Qeχ) is proportional to the dehydration factor χ representing the degree to which the superficial layer of water above the mucus hydrogel that supplies evaporative water on inhalation is not restored on condensation of super-saturated water during exhalation. (b) Signalling pathways triggered by dehydration as associated with cilia-compression-activated adenosine triphosphate release.
We sought a basic, quantitative, continuum understanding of condensation layers as relates to these questions and their implications to respiratory health and the activation of neural pathways (Fig. 1). Previous analyses of water evaporation and heat transfer within human lungs during processes of breathing (see Ferron et al., Reference Ferron, Haider and Kreyling1985; Wu et al., Reference Wu, Tawhai, Hoffman and Lin2014; Karamaoun et al., Reference Karamaoun, Sobac, Mauroy, Van Muylem and Haut2018; Haut et al., Reference Haut, Nonclercq, Buess, Rabineau, Rigaut and Sobac2021, and the references therein), while quantifying humidification phenomena of inhaled air and overall evaporative draw from the airways as functions of depth of penetration of dry inhaled air, have omitted consideration of the evaporative physical stresses imparted on the airways by treating ALF as a single homogeneous layer of water with homogeneous transport properties. The existence of the condensation layer, and its relevance to airway function, appears therefore not to have been noted.
We explored airway fluid structure and function in healthy human airways during normal tidal breathing and sustained strenuous exercise [high minute volume (100 l min−1) and duration of approximately 1 h; (Joist et al., Reference Joist, Hoenderop and Bindels2005)] with a micro-structural model of mucus based on a classical hydrodynamic model presented elsewhere (Anderson and Malone, Reference Anderson and Malone1974) (see the Methods section and the Supplementary Material). The results of our research are reported here – along with a reflection on the implication of these results to recent discoveries of the nano- and micro-structural nature of respiratory gas and water exchange.
Results
Biophysics model
Evaporation from the airways during normal tidal breathing promotes osmotic movement of water through the ALF towards the air surface by way of an imbalance of osmolytic solutes including deposited particles and salt ions (concentrations of the latter being dominant in airway lining fluid, we refer only to salt ions in our analysis, see Supplementary Material) above and below the mucus layer. We find that this imbalance can be expressedFootnote 1 by time-averaged condensation layer and PCL salt ion concentrations relative to the salt ion concentration of surrounding tissue, C*:


The osmotic pressure imbalance (ΔΠ) created by the difference between Eqs. (1) and (2) pulls water from epithelial cells and surrounding tissues into the PCL and up through the condensation layer in proportion to a condensation factor χ implicit to which is a dehydration factor ξ, as further analysed in footnote Footnote 2 and in the Supplementary Material. The osmotic pressure ΔΠ displaces the mucus towards the epithelium, reducing the thickness of the PCL over many breaths of inhalation and exhalation time T by an amount

where A is the cross-sectional area of the airway region in question and ρ the mass density of water. In healthy hydrated airways, the condensation layer thickens to approximately the same extent (see the Supplementary Material for an exact expression), notably (Matsui et al., Reference Matsui, Davis, Tarran and Boucher2000).

The mucus layer, therefore, displaces relative to the condensation layer and PCL without loss of ALF volume, whereas, at low values of epithelial or mucus permeability, the condensation layer can thin (see the Supplementary Material) and even recede into the mucus, drying out mucus.
PCL thickness can be restored (or further thinned) by deposition of hypertonic (or hypotonic) saline on the surface of the ALF. Unlike the case of isotonic saline, for which deposition on the surface of ALF increases the condensation layer thickness without increasing PCL thickness and, therefore, is incapable of modulating dysfunction (Marshall et al., Reference Marshall, Gibson, Romer, Illidi, Hull and Kippelen2021), deposition of droplets of mass MD with hypertonic (or hypotonic) salt ion concentration CD may raise (or lower) the tonicity of the ALF sufficiently to hydrate (or dehydrate) the PCL by osmotic water flux from epithelial cells. This mass can be estimated by a simple mass balance. With uniform deposition of a mass of droplets MD in the upper airways (nose and trachea), the osmotic displacement of the mucus dosm in the direction of the airway lumen can be approximated for salt concentrations up to ~10% tonicity and after many breaths on time average by (see the Supplementary Material)

The evaporative osmotic force (ΔΠ) pressing down on the PCL reduces cilia motility (Davis and Lazarowski, Reference Davis and Lazarowski2008; Hill et al., Reference Hill, Swaminathan, Estes, Cribb, O’Brien, Davis and Superfine2010) and promotes the secretion of ATP (Davis and Lazarowski, Reference Davis and Lazarowski2008; Button et al., Reference Button, Okada, Frederick, Thelin and Boucher2013) among other biomarkers (Fig. 1b), such as the inflammatory cytokines interleukin-1β, interleukin-6 and tumour necrosis factor-α (Cromwell et al., Reference Cromwell1992; Hewitt and Lloyd, Reference Hewitt and Lloyd2021), to a degree

that follows from a first-order approximation relative to the base state (0) by a linear relationship between the secreted biomarker (B) and the osmotic force or mucus displacement distance (see the Supplementary Material). Here, αB is a dimensional airway constant (cm2 mg−1) that can be directly determined as described below from published data of ATP secretion following the compression of ciliated epithelial cells in vitro (Button et al., Reference Button, Okada, Frederick, Thelin and Boucher2013). Similar first-order approximate relationships can be expressed for diminution of cilia beat frequency (CBF) and elevation of exhaled breath particles (EBPs)Footnote 3 (Edwards et al., Reference Edwards, Brenner and Wasan1991; Lucassen-Reynders, Reference Lucassen-Reynders1993; Deng et al., Reference Deng, Dong and Liang2022) (see the Supplementary Material).
Condensation layer evolution, ATP secretion and neural activation of asthmatic airways
We sought to determine whether the enhancement of ATP [approximated by Eq. (6)] consequent to the normal breathing of dry air might generate sufficient ATP to elicit cough by activation of P2X3 receptors in hypersensitive (asthmatic) airways (Fowles et al., Reference Fowles, Rowland, Wright and Morice2017), and, therefore, whether rehydration of the upper airways [see Eq. (5)] might benefit airway function, and in the case of hypersensitive airways complement the ATP-blocking action of P2X3 antagonist drugs against chronic cough (Abdulqawi et al., Reference Abdulqawi, Dockry, Holt, Layton, McCarthy, Ford and Smith2015; Smith et al., Reference Smith, Kitt, Morice, Birring, McGarvey, Sher, Li, Wu, Xu, Muccino and Ford2020; Zhang et al., Reference Zhang, Sykes, Sadofsky and Morice2022). We assumed a base case of the nasal or mouth breathing of warm air (30°C) in circumstances of human breathing ranging from dry (10% RH) to moist (60% RH) air. We assumed in all tidal-breathing cases a tidal volume of 0.5 l with fast (T = 1 s), moderate (T = 2 s) and slow (T = 5 s) breaths. Assuming a 1-s transition from inhalation to exhalation, these cases correspond to a range of 20 breaths per minute (T = 1 s) to 5–6 breaths per minute (T = 5 s). We also considered the case of mouth breathing during strenuous exercise (increasing the ventilation rate by a factor 10 from the fast-breathing case, i.e., from 10 to 100 l min−1). The results of our analysis are summarised in Table 1 (see the Supplementary Material).
Table 1. Airway hydration parameters as a function of breathing parameters and environmental conditions. (1) Airway dehydration factor ξ following the inhalation of dry (10% RH) or moist (60% RH) warm (30 C) air, with an inhalation time T of 1, 2 or 5 s, and a temperature at the carina of 35°C. (2) Water mass evaporated (QeT) in milligrammes up to the carina (upper airways), and relative humidity at the carina (RHinh), on the inhalation of dry (10% RH) or moist (60% RH) warm (30°C) air, with an inhalation time T of 1, 2 or 5 s, and a temperature at the carina of 35°C (Table 2). (3) Depth of penetration by Weibel airway generation number of unsaturated air, and inhaled air volume (cm3) to saturation beyond the carina (Vsat), on the inhalation of dry (10% RH) or moist (60% RH) warm (30°C) air, with an inhalation time T of 1, 2 or 5 s, and a temperature at the carina of 35°C. (4) Displacement of mucus or thinning of PCL (micrometres) on the inhalation of dry (10% RH) or moist (60% RH) warm (30°C) air, with an inhalation time T of 1, 2 or 5 s, and a temperature at the carina of 35°C.

Fig. 2 shows the predicted evolution of the condensation layer as a function of the breathing conditions as described in Table 1. Normal (hydrated) permeabilities of the mucus and epithelial cell layers were assumed to be 1,000 μm s−1 and 100 μm s−1 (see Supplemental Material) (Matsui et al., Reference Matsui, Davis, Tarran and Boucher2000), recognising airway permeabilities have been reported over a wide range (Schmidt et al., Reference Schmidt, Michel, Braubach, Fauler, Neubauer, Thompson, Frick, Mizaikoff, Dietl and Wittekindt2017) and that ionic strength, pH, dehydration and mucin secretion can all reduce osmotic membrane permeabilities (Tomar et al., Reference Tomar, Tyagi, Choonara, Kumar and Pillay2014; Etzold et al., Reference Etzold, Linden and Worster2021). The breathing of dirty air may further diminish mucus water permeability (see the Supplementary Material)

Fig. 2. (a) Time-averaged condensation layer thickness as a function of the normalised epithelial (red) and mucus (blue) permeabilities in conditions of fast (T = 1 s) breathing of dry (10% RH) air via the nose (solid lines) or mouth (dashed lines) at rest or exercise. (b) Time-averaged normalised concentration as a function of the normalised epithelial (Black) and mucus (blue) permeabilities in conditions of fast (T = 1 s) breathing of dry (10% RH) air via the nose (solid lines) or mouth (dashed lines) at rest or exercise.
So long as water permeates effectively across epithelial and mucus barriers (normalised water permeability approximately 20% or greater of fully hydrated levels), the consequence of water evaporation from the upper ALF (Fig. 2a) is to slightly thicken the condensation layer (while thinning the PCL to the same degree), thereby conserving overall ALF volume. As breathing rate (minute volume) increases to 100 l min−1 (strenuous exercise), the thickening of the condensation layer reaches around 0.7 μm, which is equivalent to the distance of displacement of the mucus membrane towards the epithelium (Table 1). The salinity of the ALF varies to a small degree (Fig. 2b) in all but the high minute-volume case as can be seen by the inset of Fig. 2b. During strenuous exercise, the salinity of the condensation layer exceeds that of the PCL, while both salt concentrations are elevated, reaching around 20–40% higher osmolarity than at rest, similar to osmolarity increases that have been measured post exercise in athletes (Tatsuya et al., Reference Tatsuya, Yoshikawa, Ueda, Orita and Fujimoto2011). In the case of strenuous exercise, relatively small changes in permeabilities (around 40% of the assumed ‘fully hydrated’ permeation rate) can lead to loss of condensation layer volume and corollary loss of ALF volume (Fig. 2a), and in the case of very low permeabilities, the condensation layer can achieve a negative thickness, meaning that mucus dries out.
The compression that drives PCL thinning [see Eq. (4)] and ATP secretion in the airways [see Eq. (6)] increases linearly with evaporation rate Qe or ventilation rate. Cough reflex in asthmatics has also been shown to increase in a linear fashion with airway ATP levels (Fowles et al., Reference Fowles, Rowland, Wright and Morice2017) following topical deposition of ATP. These relationships are portrayed with supporting experimental data in Fig. 3a. We determined the linear coefficient characterising ATP concentration increase [see Eq. (6)] as αATP ~ 245 cm2 mg−1 by fitting data gathered in direct in vitro measurements of ATP secretion levels (50 nm) following compression (20-cm H2O) of cilia beneath a mucus mimetic gel (Button et al., Reference Button, Okada, Frederick, Thelin and Boucher2013). The linear coefficient characterising the relationship between cough frequency (CF) (coughs per minute) and mucus displacement [see Eq. (4)] is determined by matching the linear prediction with human CF data gathered on topical administration of ATP (Fowles et al., Reference Fowles, Rowland, Wright and Morice2017), leading to a remarkably simple relationship between CF (coughs per minute) in hypersensitive (asthmatic) human airways and the product of evaporation rate Qe (mg s−1), condensation factor χ (dimensionless) and inhalation time T (s):


Fig. 3. Predicted and experimentally measured (as reported by Button et al., Reference Button, Okada, Frederick, Thelin and Boucher2013; Fowles et al., Reference Fowles, Rowland, Wright and Morice2017) relationships between minute volume, adenosine triphosphate (ATP) concentration in the airways and cough incidence. (a) Predicted (solid Black line) time-averaged steady-state osmotic pressure force acting on the PCL as a function of minute volume (with maximum assumed minute volume of 200 l min−1). The predicted linear relationship between osmotic pressure and ATP concentration is based on the first-order approximate expression Eq. (6) with αB = αATP ~ 245 cm2 mg−1 determined by the measured ATP extracellular concentration (50 nm) and compressive force (20-cm H2O) reported in Button et al. (Reference Button, Okada, Frederick, Thelin and Boucher2013). The predicted linear relationship between (asthmatic airway) CF and ATP concentration is based on the first-order approximate expression Eq. (7) where the unity coefficient is determined by fit of Eq. (7) to the measured CF observed at the two ATP levels shown by the dotted red line by Fowles et al. (Reference Fowles, Rowland, Wright and Morice2017) on topical delivery of ATP at the estimated ALF concentrations depicted in the figure, with the airway lining fluid concentrations being estimated by the delivery efficiencies reported elsewhere (Schlesinger and Lippmann, Reference Schlesinger and Lippmann1976; Khan et al., Reference Khan, McDonough, Cockcroft, David and Hendeles2005). (b) Cough incidence (number of coughs) versus minute volume as a percentage of maximum minute volume following the deep breathing of dry air. Experimental data are as reported by Purokivi et al. (Reference Purokivi, Koskela and Brannan2011) for asthmatic subjects, and the prediction (thick red line) is based on Eq. (7) (Fig. 3a) without fitted parameters.
An identical relationship follows from the data of Fowles et al. (Reference Fowles, Rowland, Wright and Morice2017) for normal (non-asthmatic) airways with CF approximately 10-fold less common. Fig. 3a presents a comprehensive pictorial representation of the biophysical mechanism by which dehydration of the upper airways elicits cough reflex in the hypersensitive airways of asthmatic human subjects.
Fig. 3b compares the predicted CF relationship determined by Fig. 3a with the results of a Finnish study (Purokivi et al., Reference Purokivi, Koskela and Brannan2011) where 36 asthmatics and 14 healthy human subjects deeply breathed very dry air. Coughs post challenge were monitored in the study for up to 10 min, with primary coughing occurring in the first minute post challenge. Fig. 3b shows the results from this study for the asthmatic group in comparison with the prediction. Our predictions, which involve no fitted parameters, follow from the 30°C, 10% RH case of Table 1, on relatively deep breathing (T = 2 s), with the totality of coughs assumed to have occurred within 1 min post challenge. Cough incidence grows linearly as ATP levels grow (Fig. 3a) with increased mechanical stress on the airway epithelium caused by the amplification of airway dehydration accompanying rising ventilation rates. Reasonable agreement is also found (not shown) between the theoretical prediction of CF on the breathing of dry air in normal human subjects (where coughs observed in Purokivi et al., Reference Purokivi, Koskela and Brannan2011, are approximately an order of magnitude less frequent than with the asthmatics].
Clearance breakdown and inflammation in healthy airways
Airway dehydration, in otherwise healthy airways, further promotes inflammation, reduction in CBF and elevation of EBP owing to the evolution of the condensation layer portrayed in Fig. 2. Table 2 summarises theory predictionsFootnote 4 of this airway dysfunction, as well as the estimated masses of 5% hypertonic saline required to reduce airway dysfunction with a ~10-μm mass median aerodynamic diameter aerosol (Calmet et al., Reference Calmet2019), in the range of breathing circumstances characterised by Table 1.
Table 2. Airway function versus breathing parameters and environmental conditions. (1) Airway adenosine triphosphate (ATP) concentration following the inhalation of dry (10% RH) or moist (60% RH) warm (30°C) air, with an inhalation time T of 1, 2 or 5 s, and a temperature at the carina of 35°C. (2) Airway inflammatory cytokine concentration following the inhalation of dry (10% RH) or moist (60% RH) warm (30°C) air, with an inhalation time T of 1, 2 or 5 s, and a temperature at the carina of 35°C. (3) Cilia beat frequency diminution (1 − CBF)/αCBF following the inhalation of dry (10% RH) or moist (60% RH) warm (30°C) air, with an inhalation time T of 1, 2 or 5 s, and a temperature at the carina of 35°C. (4) Breakup factor B increase (1 − B)/αB on the inhalation of dry (10% RH) or moist (60% RH) warm (30°C) air, with an inhalation time T of 1, 2 or 5 s, and a temperature at the carina of 35°C. (5) Mass of hypertonic saline (CD/C * = 5) needed to restore the PCL thickness following the inhalation of dry (10% RH) or moist (60% RH) warm (30°C) air, with an inhalation time T of 1, 2 or 5 s, and a temperature at the carina of 35°C. (5) Mass of hypertonic saline (CD/C * = 5) needed to restore the PCL thickness following the inhalation of dry (10% RH) or moist (60% RH) warm (30°C) air, with an inhalation time T of 1, 2 or 5 s, and a temperature at the carina of 35°C.

We compared the predictions of Table 2 with a range of published human clinical data in Fig. 4. Fig. 4a, b shows a comparison with human clinical data gathered in states of strenuous (1 h) exercise as reported in Osaka (Tatsuya et al., Reference Tatsuya, Yoshikawa, Ueda, Orita and Fujimoto2011), Boston (George et al., Reference George, Scheuch, Seifart, Inbaraj, Chandrasingh, Nair, Hickey, Barer, Fletcher, Field, Salzman, Moelis, Ausiello and Edwards2022) and Munich (Mutsch et al., Reference Mutsch, Heiber, Grätz, Hain, Schönfelder, Kaps, Schranner, Kähler and Wackerhage2022) studies with predictions based on the case of inhaled air at 30°C and 10% RH.Footnote 5 The correlation of prediction and experiment reflects the fitting of these exercise data with the predictions for the determination of the linear perturbation constants.Footnote 6 The elevation of inflammatory cytokines in the saliva of the athletes post exercise in the Osaka study (Fig. 4a) parallels the rise in ATP observed with in vitro compression of cilia (Button et al., Reference Button, Okada, Frederick, Thelin and Boucher2013) and as predicted owing to the osmotic stress transmitted to the airway epithelium at high ventilation rate with the mouth breathing of dry air (Fig. 3a). The far greater predicted elevation of ATP (Fig. 3a) with ventilation rate accompanying strenuous exercise (50% of maximum minute volume), relative to the rise of inflammatory cytokines (Fig. 4a), is similar to the rise (approximately eightfold) in EBPs post exercise observed in both the Boston (George et al., Reference George, Scheuch, Seifart, Inbaraj, Chandrasingh, Nair, Hickey, Barer, Fletcher, Field, Salzman, Moelis, Ausiello and Edwards2022) and Munich (Mutsch et al., Reference Mutsch, Heiber, Grätz, Hain, Schönfelder, Kaps, Schranner, Kähler and Wackerhage2022) studies, suggesting relative EBP rise as an indicator of upper airway dehydration. Fig. 3c,d compares predictions (obtained without fitted coefficients) of CBF and EBP with data obtained in tidal breathing conditions via independent Mannheim (Birk et al., Reference Birk, Händel, Wenzel, Kramer, Aderhold, Hörmann, Stuck and Sommer2017) and Cambridge (Reihill et al., Reference Reihill, Douglas and Martin2021) studies. In the Mannheim study (Birk et al., Reference Birk, Händel, Wenzel, Kramer, Aderhold, Hörmann, Stuck and Sommer2017) (see Fig. 3c), newly tracheostomised patients were treated either with cool (ambient temperature) dry (compressed) air and nebulisation of isotonic saline or with heated (37°C) humidified (100% RH) air 8 h per day for 14 days post tracheotomy. Epithelial tracheal cells were harvested at days 2, 4, 6, 8 and 10 post surgery and CBF measured in vitro in both (non-randomised) groups. Nasal CBF diminishes on exercise with dehydration within the range of the predications based on moderate (intermediate to slow and fast) tidal breathing rate. In the Cambridge study (Reihill et al., Reference Reihill, Douglas and Martin2021) (see Fig. 4d), human subjects mouth breathed ambient (25°C) humid (40–50% RH) air for 20 min, then moved into a dry air (10% RH) ambient temperature environment where they remained for 2 h (Fig. 3d). The elevation of EBF on moving from the humid to the dry air environment in the Cambridge (Reihill et al., Reference Reihill, Douglas and Martin2021) study is roughly twofold at tidal breathing, close to the predicted value (see Fig. 3d; see also Table 2).

Fig. 4. (a) Post-exercise exhaled inflammatory marker concentration (CI) relative to pre-exercise. Data represent mean values (grey boxes) with standard deviations reported from Osaka study (Tatsuya et al., Reference Tatsuya, Yoshikawa, Ueda, Orita and Fujimoto2011). Prediction (Black box) is based on Eq. (36) of the Supplementary Material in the case of exercise (Table 2). (b). Post-exercise exhaled breath particles (EBPs) relative to pre-exercise. Data represent mean values (grey boxes) and standard deviations as reported from the Boston study (George et al., Reference George, Scheuch, Seifart, Inbaraj, Chandrasingh, Nair, Hickey, Barer, Fletcher, Field, Salzman, Moelis, Ausiello and Edwards2022), the Munich study (all participants) (Mutsch et al., Reference Mutsch, Heiber, Grätz, Hain, Schönfelder, Kaps, Schranner, Kähler and Wackerhage2022) and the Munich study (seasoned athletes only) (Mutsch et al., Reference Mutsch, Heiber, Grätz, Hain, Schönfelder, Kaps, Schranner, Kähler and Wackerhage2022). Prediction (Black box) is based on Eq. (38) of the Supplementary Material in the case of exercise (Table 2). (c) Cilia beat frequency post exposure to dry air (with isotonic saline) or perfectly humid air in patients following tracheotomy as reported by Birk et al. (Reference Birk, Händel, Wenzel, Kramer, Aderhold, Hörmann, Stuck and Sommer2017). Prediction (Black box) and standard deviation is based on the dry air (10% RH) mouth-breathing case of Table 2 with maximum of 20 and a minimum of 6 breaths per minute (i.e. 1 or 5 s inhalation times). (d) EBP post exposure to dry air (10% RH) relative to EBP in humid room conditions (40–50% RH) (Dehydration) (grey box) and following the delivery of 5% hypertonic saline to the upper airways (Rehydration) (grey boxes). Data represent mean values and standard deviation as reported from the Cambridge study (Reihill et al., Reference Reihill, Douglas and Martin2021). Prediction is based on Eq. (38) of the Supplementary Material in the case of fully hydrated airway lining fluid [equivalent to the case of slow (T = 5 s) nose breathing].
Rehydration of the upper airways
Following the breathing of dry air for 2 h, the subjects in the Cambridge study (George et al., Reference George, Scheuch, Seifart, Inbaraj, Chandrasingh, Nair, Hickey, Barer, Fletcher, Field, Salzman, Moelis, Ausiello and Edwards2022) inhaled via the nose approximately 10 mg of 5% hypertonic saline (with either NaCl, or CaCl2 or MgCl2) amounting to an estimated 3 mg deposition in the larynx and trachea based on a mass-median droplet diameter of around 10 μm (Calmet et al., Reference Calmet2019). Rehydration of the upper airways by the nasal inhalation of 5% NaCl, or CaCl2 or MgCl2 reduces EBP to levels reflective of the breathing of humid air (Fig. 4d). The measured reduction of EBP is close to the predicted value based on the 2.5-mg 5% hypertonic saline case of Table 2 relative to the dry air (10% RH) case reflecting the impact of rehydrating the PCL via the osmotic action of hypertonic salts on the epithelial cell layer. While the three salts show similar degrees of suppression (George et al., Reference George, Scheuch, Seifart, Inbaraj, Chandrasingh, Nair, Hickey, Barer, Fletcher, Field, Salzman, Moelis, Ausiello and Edwards2022) as predicted by the osmotic rehydration mechanism (Fig. 2), clearance times, and therefore duration of rehydration, will vary between the salts, the monovalent sodium clearing more rapidly than the divalent calcium and magnesium, as reported in the Cambridge study (George et al., Reference George, Scheuch, Seifart, Inbaraj, Chandrasingh, Nair, Hickey, Barer, Fletcher, Field, Salzman, Moelis, Ausiello and Edwards2022) and has been reported elsewhere (George et al., Reference George2020; Field et al., Reference Field, Moellis, Salzman, Bax, Ausiello, Woodword, Wu, Domici and Edwards2021).
Discussion
We find that the osmotic movement of water necessary to support humidification of relatively dry inhaled air produces a net physical force originating in a condensation layer that presses mucus towards the airway epithelium, stressing cilia, secreting ATP and activating neural pathways (Figs 1 and 3). This stress can engender cough in situations of cough hypersensitivity (Fig. 3b), and inflammation and clearance breakdown in relatively healthy airways (Fig. 4).
Previous theoretical considerations of the humidification of inhaled air have focused on predicting degrees of humidification of inhaled air as a function of ambient temperature and humidity in a wide range of breathing conditions (see Ferron et al., Reference Ferron, Haider and Kreyling1985; Wu et al., Reference Wu, Tawhai, Hoffman and Lin2014; Karamaoun et al., Reference Karamaoun, Sobac, Mauroy, Van Muylem and Haut2018; Haut et al., Reference Haut, Nonclercq, Buess, Rabineau, Rigaut and Sobac2021, and the references therein). By assuming airway fluid to be a homogeneous medium without osmotic restriction of ion and particle transport, previous analyses have omitted consideration of the physical stresses imparted on the airways by water evaporation. While the condensation of moisture on the upper airways during exhalation has been noted and analysed [see e.g. Haut et al., Reference Haut, Nonclercq, Buess, Rabineau, Rigaut and Sobac2021], the accumulation of the condensed water on top of mucus owing to the immediate diffusion of osmolytes from the mucus into the condensation layer, and the restricted movement of these osmolytes back into the ‘transpiring hydrogel’ of mucus, has been ignored, if only because the role of mucus in the regulation of water transport has not previously been considered. This has left unaddressed the question as to how, or if, physical stresses imparted to the airways by natural water evaporation are significant, and, therefore, whether these stresses might underpin phenomena of airway dysfunction that have long been reported as associated with the breathing of dry air (Barbet et al., Reference Barbet, Chauveau, Labbe and Lockhart1988; Purokivi et al., Reference Purokivi, Koskela and Brannan2011; Ghosh et al., Reference Ghosh, Boucher and Tarran2015; D’Amato et al., Reference D’Amato, Molino, Calabrese, Cecchi, Annesi-Maesano and D’Amato2018; Mecenas et al., Reference Mecenas, Bastos and Normando2020; Romaszko-Wojtowicz et al., Reference Romaszko-Wojtowicz, Cymes, Dragańska, Doboszyńska, Romaszko and Glińska-Lewczuk2020; Moriyama et al., Reference Moriyama, Hugentobler and Iwasaki2021). Our conclusion that these phenomena are indeed significant enough to alter ALF structure and function is consistent with general physical understanding of ‘transpiring hydrogels’ in nature (Wheeler and Stroock, Reference Wheeler and Stroock2008; Etzold et al., Reference Etzold, Linden and Worster2021).
The ‘condensation layer’ protects mucus from drying out by growing in thickness as the mucus displaces towards the epithelium (Fig. 2). In circumstances of poor epithelial and/or mucus water permeation, the condensation layer can recede into the mucus and disappear as topical mucus moisture, a condition we find occurs most readily on the mouth breathing of dry air at high minute volume – combining three of the frequent contemporary conditions that dehydrate the airways, an extreme case of which is strenuous exercise on a cold day (Fig. 2). The circumstance of poor water permeation arises in the disease condition of cystic fibrosis, where defects in the CFTR gene prevent chloride transport across the apical membrane of epithelial cells (Weiser et al., Reference Weiser, Molenda, Urbanova, Bähler, Pieper, Oberleithner and Schillers2011), and between cells via the paracellular route (Weiser et al., Reference Weiser, Molenda, Urbanova, Bähler, Pieper, Oberleithner and Schillers2011), thereby preventing water transport into the airways. CF has long been studied as a condition of chronic and excessive airway dehydration (Boucher, Reference Boucher2007). Among therapeutic approaches to relieve the symptoms of cystic fibrosis, rehydration of the airways by the delivery of hypertonic (NaCl) saline (Kelly et al., Reference Kelly, Martinsen and Tatkov2021) has proven particularly useful, if of shorter duration than is observed with hypertonic salines of divalent salts (CaCl2 and MgCl2) (Edwards et al., Reference Edwards2004; George et al., Reference George2020; Field et al., Reference Field, Moellis, Salzman, Bax, Ausiello, Woodword, Wu, Domici and Edwards2021), which nevertheless benefit rehydration of the upper airways to similar degrees (Fig. 4d), given that all the salts act by the same physical airway hydration mechanism.
Our analysis indicates that the topical delivery of hypertonic saline to the upper airways will hydrate the PCL after many breaths and in the steady-state conditions of the analysis, thereby reducing pressure on underlying cilia and reducing ATP secretion [see Eq. (5), Table 2 and Fig. 4d]. It appears, therefore, that the delivery of hypertonic salines to the upper airways with a droplet size of approximately 10 μm (i.e. in the approximate range of 8–15 μm) may provide an effective means of hydrating the upper airways and reducing propensity to cough in those with hypersensitive cough syndrome. By reducing the ATP trigger of P2X3 cough receptors, upper airway hydration with hypertonic salines, and particularly with divalent (calcium and magnesium) salines that retain hydration effects longer than monovalent (sodium) salines (Edwards et al., Reference Edwards2004; George et al., Reference George2020; Field et al., Reference Field, Moellis, Salzman, Bax, Ausiello, Woodword, Wu, Domici and Edwards2021), might also be a helpful adjuvant to the efficacy of P2X3 antagonist drugs. The combination of benefits of upper airway hydration with hypertonic salts implied by the relationship between airway dysfunction and upper airway hydration state indicated in Figs 3a and 4a– d suggests that the hydrating of the upper airways may confer in normally hydrating airways at risk of respiratory illness prophylactic and treatment benefits (George et al., Reference George, Scheuch, Seifart, Inbaraj, Chandrasingh, Nair, Hickey, Barer, Fletcher, Field, Salzman, Moelis, Ausiello and Edwards2022) akin to the benefits conferred in CF airways by full-lung airway hydration with hypertonic salines (Kelly et al., Reference Kelly, Martinsen and Tatkov2021).
Consistent with our observations is that topical administration of hypotonic saline has previously been shown to induce cough (Ventresca et al., Reference Ventresca, Nichol, Barnes and Chung1990). Notably, in (Ventresca et al., Reference Ventresca, Nichol, Barnes and Chung1990), pure water was administered by a nebuliser for approximately 1 min to non-asthmatic human subjects, with an output (around 20 mg per breath) suggesting ±60 mg of saline deposited in the trachea. This is approximately the quantity of water in tracheal ALF, suggesting from Eq. (6) a loss of approximately 50% of PCL thickness. This dramatic thinning of the PCL by the prolonged nebulisation of water will produce approximately five times the stress on cilia relative to the case analysed here of strenuous exercise and the breathing of dry air (Table 2) – and is, therefore, consistent with the observation that even normal human subjects tend to cough significantly on the breathing of pure water droplets or hypotonic aerosols.
There are many limitations to our analysis. We have used a continuum mechanics analysis to study water exchange between ALF and inhaled/exhaled air. Osmotic forces are themselves necessarily continuum principles, reflecting the restricted movement of solutes (ions, particles and other osmolytes) through a semi-permeable membrane (the mucus and airway epithelium in our model) (Anderson and Malone, Reference Anderson and Malone1974). The analysis does not, therefore, explicitly address the substructure of matter, leaving unaddressed the molecular composition of the condensation layer in the upper airways. Recent discoveries (Ninham et al., Reference Ninham, Reines, Battye and Thomas2022) of the role played by pulmonary surfactant in the movement of gases between the alveolar lumen and the systemic circulation suggest that the absorption of oxygen and nitrogen from inhaled air may significantly occur by way of nano-bubbles of oxygen and nitrogen formed in a lattice of pulmonary surfactant, on inhalation, seemingly transitioning to carbon dioxide and water on exhalation (Ninham et al., Reference Ninham, Reines, Battye and Thomas2022). Such nano-bubbles have an even better documented role in the endothelial surface layer (Reines and Ninham, Reference Reines and Ninham2019), where they reside within the glycocalyx (Reines and Ninham, Reference Reines and Ninham2019) and facilitate the oxygenation of blood. Nano-bubbles, whose dimensions range between 4 nm to approximately 40 nm, appear to have extremely small surface tension, permitting the containment of gas at realistic pressures. Conceivably, nano-bubble breakage on the expansion of the lattice structure of pulmonary surfactant in the small airways may contribute to small-airway respiratory droplet formation whose origin has long been held to occur to the ‘necking’ of surfactant between small airway walls (Scheuch, Reference Scheuch2020). The water that condenses on the upper airways on exhalation may then contain molecular and structural elements of nano-bubble forms in the alveolar region of the lungs and promote another ‘salt effect’ on the composition of the condensation layer that we do not consider in our study. That is, nano-bubbles are stabilised at a critical salt concentration of approximately 0.17 M (Zhou et al., Reference Zhou, Han, He, Feng, Wang, Wang, Luo, Dodbiba, Wei, Otsuki and Fujita2021; Ninham et al., Reference Ninham, Reines, Battye and Thomas2022), slightly above isotonic salt concentration, while this number is suppressed with convection (Nguyen et al., Reference Nguyen, Hampton, Nguyen and Birkett2012), as occurs along the surface of ALF in the upper airways. Should nano-bubbles exist in the condensation layer, the elevation of salt concentration that occurs on dehydration (Fig. 2b) might trigger their stabilisation and contribute to the lowering of surface tension and the frothiness of upper airway condensation layer that is observed to occur when the upper airways are dry (Fig. 4) (see Edwards et al., Reference Edwards2004; George et al., Reference George2020; Field et al., Reference Field, Moellis, Salzman, Bax, Ausiello, Woodword, Wu, Domici and Edwards2021). While our continuum analysis has permitted resolution of osmotic forces and prediction of macroscopic displacement of mucus, stresses on underlying epithelia and dysfunction as in the promotion of cough reflex, to understand macroscopic coefficients such as appear in Eq. (6) (and related relationships for CBF and EBP), molecular and cellular substructure and dynamics will need to be considered, and it is intriguing that the evolving understanding of gas and water exchange in the small airways may lead to new insights in the structure and function of the upper airways as well.
Our estimates of airway dysfunction are based on very small perturbations in PCL thickness and are therefore insufficient for estimating dysfunction in severely dehydrated airways. Our assumption of warm equatorial air simplified energy considerations, and avoided an obvious circumstance where further airway dehydration occurs, notably on the breathing of cold air (Barbet et al., Reference Barbet, Chauveau, Labbe and Lockhart1988; D’Amato et al., Reference D’Amato, Molino, Calabrese, Cecchi, Annesi-Maesano and D’Amato2018; Mecenas et al., Reference Mecenas, Bastos and Normando2020). Breathing cold air leads to air of very low water content (similar to our 10% RH case) while also requiring heating of inhaled air in the upper airways, which reduces evaporation loss, and has been well studied in previous work [see e.g. Haut et al., Reference Haut, Nonclercq, Buess, Rabineau, Rigaut and Sobac2021]. In our analysis, we assumed effective ion exchange between compartments of the ALF and therefore ignored a special circumstance as occurs in cystic fibrosis where the inability for chloride ions to transport across the epithelial membrane blocks water movement into the airways. This case of very low ‘effective’ epithelial water permeability results in circumstances that in practice resemble closely what is revealed in Fig. 2 in the circumstance of vanishingly small epithelial water permeability. The mucus dries out, and eventually the PCL does as well, resulting in a thickening of the mucus and an adherence of the mucus against the apical epithelial wall. We did not consider the shrinkage that can occur of the mucus layer owing to the dehydration of mucus, and the evolution of ionic content of the mucus, as can arise in relatively dehydrating breathing circumstances. This phenomenon has recently been studied and shown to produce shrinkage up to approximately 40% of a characteristic gel diameter, meaning a volume reduction of an order of magnitude or more. While our elucidation of condensation layer biophysics aligns with many general observations of airway hydration, dehydration and dysfunction, further comparison of model predictions with experiments is needed, and assumptions inherent in the model should be relaxed and consequences explored.
Humidifying inhaled air in conditions other than the slow nasal breathing of relatively warm and moist air can harm respiratory health by upper airway structural and function challenges aggravated by the breathing of dirty air. We find that these challenges – originating in osmotic stresses exerted by a condensation layer of water that wets airway lining mucus – can be offset by a combination of effective breathing habits, the humidification of inhaled air and the rehydration of the upper airways by hypertonic salt aerosols. Maintaining proper hydration of the upper airways may be necessary to combat the global respiratory health crisis associated with the breathing of contaminated air.
Methods
Our analysis of the biophysics of the condensation layer, and of the physical structure of ALF, during processes of breathing is based on the fundamental assumption that solutes within the ALF transport relatively rapidly across transport barriers of the epithelium and mucus on the time scale of the many breaths required to establish a steady-state time-averaged structure in a particular breathing condition. This assumption implies healthy relatively hydrated airways wherein epithelial transporters properly function and airway mucus is not particularly dehydrated. We further assume sufficiently small differences in temperature between the outside environment and body temperature such that heat transfer occurring on the evaporation and condensation of water in the airways has a small impact on the overall rates of water evaporation and condensation. These and other assumptions are listed in Table 3, and the full analysis is provided in the Supplementary Material.
Table 3. Base assumptions of biophysical model of hydration and dehydration of the human airways. See Supplementary Table S1 for a summary of the Weibel model characteristics on which all calculations in the central airways are based.
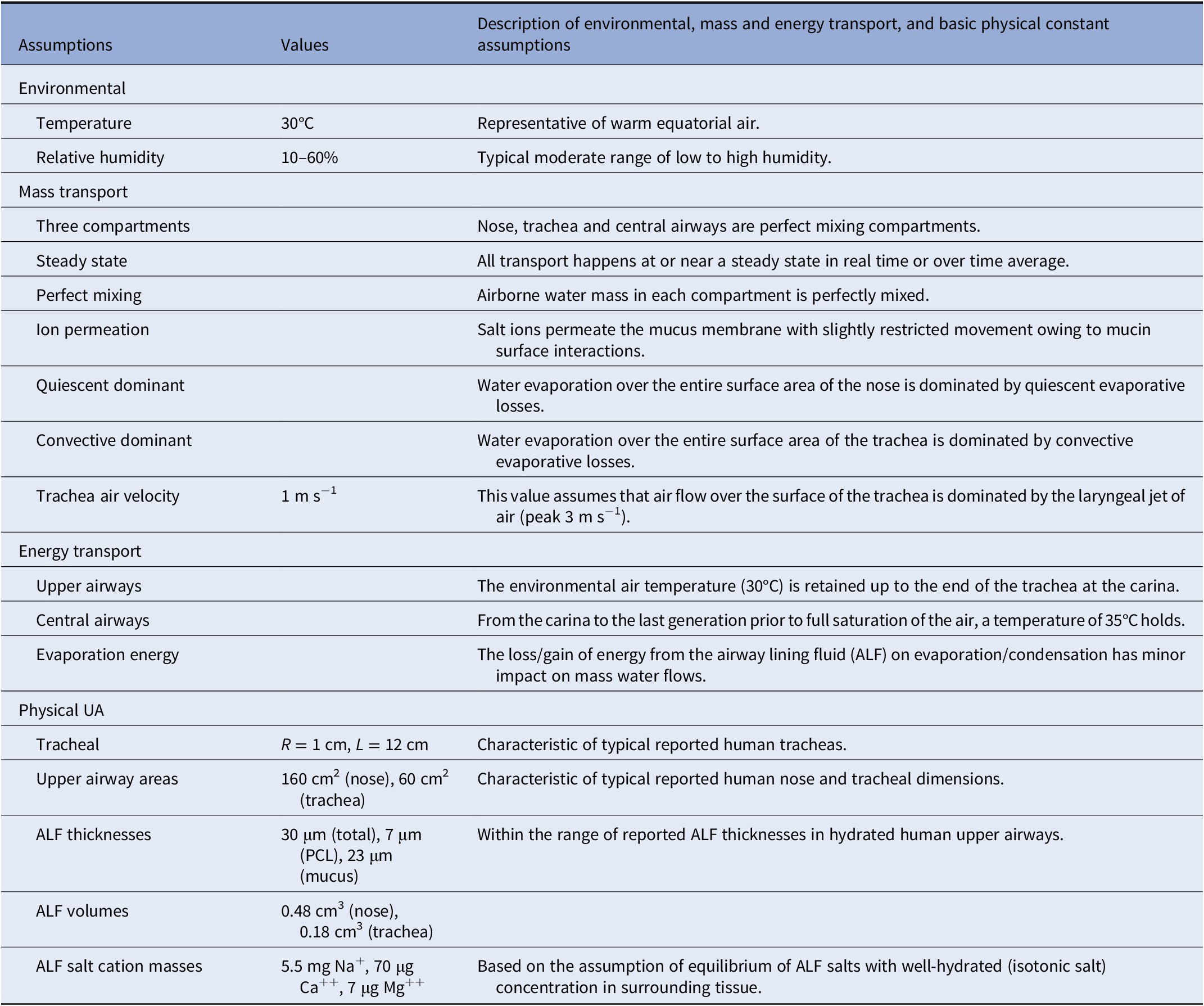
We assumed perfect mixing of air and water within the ‘compartments’ of the nose (and nasal pharynx), trachea (and larynx) and bronchi (and bronchioles) and used upper airways dimensions as reported in the literature for human airways, whereas from the carina to the small airways, we assumed Weibel lung geometry (see the Supplementary Material). We assume warm ambient air at 30°C. We assumed the gradient of temperature from the outside air to the carina to be such that outside ambient temperature exists up to the carina at which point the air temperature rises to 35°C. This gradient is approximately as has been observed in thermal mapping studies (Nguyen et al., Reference Nguyen, Hampton, Nguyen and Birkett2012) on the breathing of room temperature (25°C) air at variable ventilation rates, notably where the nose and trachea temperatures ranged from 29 to 32°C and the main bronchi temperatures from approximately 34 to 36°C. All of our mass transport considerations assumed steady or nearly steady-state flow conditions representing time averages of inhalation, exhalation and many cycles of repetitive tidal breathing. We assumed the base case of warm air (30°C) with high (60%) and low (10%) relative humidity (RH), and inhalation and exhalation times of 1, 2 or 5 s, ranging from fast to slow breathing. We also considered a case of ‘exercise’, as a special case of fast mouth breathing with a velocity of air flow 10 times elevated relative to normal breathing.
Given the very thin ALF relative to the curvature radii of the airways (ranging from hundreds of microns to several millimetres), we assumed the ALF to be essentially flat and of ‘infinite’ lateral dimension, with the principal physics of water and ion transport occurring in one dimension (Fig. 1). Fully hydrated ALF is assumed to be 30-micron thick in the nose and tracheal compartments, and 10-micron thick from the carina to the small airways, with a PCL of structured water of approximately 7-micron thickness, covered by a mucus hydrogel layer whose structure is static, over which is a thin layer of water from which water is directly exchanged with inhaled and exhaled air. This condensation layer of water will inevitably be thickest following the slow exhalation of relatively warm humid air and thinnest following the rapid inhalation of relatively cool dry air. We assumed a steady-state fully hydrated condensation layer thickness of approximately 1 μm given the water content of supersaturated state of exhaled air during an exhalation into a relatively warm trachea.
As principal air flow in the nasal cavity (approximately 12-cm length from the tip of the nose to the nasal pharynx) occurs in the narrow air passage of the middle or inferior meatus (approximately 0.2 cm radius, or approximately 10-cm2 surface area), we assume relatively quiescent conditions over the majority of ALF surface area within the nose (approximately 160 cm2) over the course of inhalation on normal tidal breathing. Principal air velocity in the trachea (approximately 12-cm length from the larynx to the carina) being driven by the jet of air that emerges from the larynx (with typical peak air velocity on inhalation of approximately 3 m s−1), we assume an average velocity on inhalation in the trachea of around 1 m s−1. Finally, we assumed salt concentrations in hydrated ALF are approximately equivalent to blood concentrations, such that in the reference state (C 0), osmolarity equilibrium has been obtained between the airways and surrounding epithelial cells and vascularised tissues. Table 3 summarises the approximate total masses of principal salt ions and water in the nose and trachea in a fully hydrated state.
The pore-level model of transport in the mucus hydrogel summarised in the Supplementary Material uses a periodic porous medium hydrodynamic model of osmosis as developed elsewhere (Anderson and Malone, Reference Anderson and Malone1974). Mucus is modelled as a porous medium with infinitely long cylindrical pores (i.e. the radius of the pores, R, is much smaller than the length of the pores or the mucus thickness, L). Each pore is identical to the other, and of an effective diameter deduced from the model by comparison of predictions with reports of experimentally measured ALF permeability values. The model shared in the Supplementary Material explicitly considers transport of salt ions together with deposited airborne particles. The model demonstrates that while restricted motion is far greater for nanoparticle than for salt diffusion within mucus, the high concentration of salts renders the net osmotic pressure by salt ions much greater than that of inhaled particles at least in conditions where cilia continue to beat. We speculate on the possibility of diminished water permeability within mucus owing to the breathing of dirty air and the roles this diminished permeation might have in the phenomena described in Figures 3 and 4. We deduced the dimensions of the model micro-structure of mucus by comparing the formulas derived in the Supplementary Material for permeability, reflection coefficient and other transport characteristics.
We determined the values of the model parameters in Table 1 by using the data summarised in Table 3 (see also Table S1 in the Supplementary Material for a summary of central airway Weibel geometry), and we determined the unknown variable needed for the determination of the dysfunction parameters appearing Table 2 by comparison with experimental data.Footnote 7 The perturbation assumptions by which we determined Eq. (6) and other airway inflammation measures are detailed in the Supplementary Material.
Open peer review
To view the open peer review materials for this article, please visit http://doi.org/10.1017/qrd.2023.1.
Supplementary materials
To view supplementary material for this article, please visit http://doi.org/10.1017/qrd.2023.1.
Conflict of interest
D.A.E. is a cofounder and shareholder of Sensory Cloud Inc. and a cofounder (while not a shareholder) of Pulmatrix. Each of these companies advances science, technology, and products for consumer health or therapeutic use by the inhalation of aerosols.
Comments
Comments to Author: I have examined with great interest the manuscript, “Breathing dry air promotes inflammation, activates neural pathways and disrupts clearance by osmotic stresses imparted on airway epithelia” because it claims to explain the rise of respiratory diseases which the authors attribute to climate change. This is attributed to chronic dehydration of the airways. To me, this is a bold claim. I do not find it supported by the evidence presented.
So, let’s turn to what is evidence is presented. It is found that water evaporation from the upper airways delivers an osmotic force on airway mucus that drives it toward the epithelium with increasing amount as the dryness of inhaled air increases. That part of the manuscript seems valid and supported. The connection of air dryness with climate change is suspected in general but not established. That respiratory disease and breathing abnormalities have been increasing seems a fact but attribute this solely to climate change strikes me as not supportable. Humans spend so much time indoors rather than outdoors and the air indoors is not controlled simply by climate change, so I am reluctant to recommend publication of this manuscript in its present form.