Introduction
European Union (EU) legislation on the restriction of hazardous substances1 as well as an articleReference Saito, Takao, Tani, Nonoyama, Takatori, Homma, Nagaya and Nakamura2 in Nature about 15 years ago provided the triggers for a strong effort into the science and technology of lead-free piezoceramics, with many stakeholders coming into play. While EU legislation restricts the use of lead in piezoelectric devices unless exempted under specific conditions,1 the article in Nature suggests that lead-free compositions may now be available to replace lead in certain piezoceramic applications.Reference Saito, Takao, Tani, Nonoyama, Takatori, Homma, Nagaya and Nakamura2 With the goal to reduce the production and waste disposal of toxic lead, research on lead-free piezoceramics strives to make lead zirconate titanate (PZT) and similar perovskite materials redundant. Shrout and ZhangReference Shrout and Zhang3 have summarized the complexity of this task in a concise manner.
The scope of this endeavor to develop lead-free piezoceramics can be gleaned by considering the history of PZT,Reference Jaffe, Cook and Jaffe4,Reference Trolier-McKinstry and Randall5 which highlights many years of development. The toxicity of lead, including risks to the environment during mining, processing, and disposal, is the driving force for this research. The article by Bell and DeubzerReference Bell and Deubzer6 in this issue summarizes the current level of understanding. Legislation in Europe, and in many other parts of the world,Reference Rödel, Jo, Seifert, Anton, Granzow and Damjanovic7 to restrict and reduce hazardous substances such as lead has proven to be a strong driving force toward research into nontoxic replacements. Review processes and interactions with industry to address a variety of legislative directives have been developed for different applications. Exemption 7(c)-I, for example, has been recently reviewed and provides an exemption for lead in piezoelectronic devices until July 2021, with applications for further extension of this exemption due to the European commission by January 2020. Bell and DeubzerReference Bell and Deubzer6 have outlined current legislation and future options.
Piezoelectricity describes the creation of an electric charge in response to a mechanical stress. The converse effect is the development of a strain as a function of an applied electric field (Figure 1). The proportionality constant is identical for both cases and is described as the piezoelectric coefficient, d.Reference Jaffe, Cook and Jaffe4,Reference Heywang, Lubitz and Wersing8 Since polarization is a vector and stress a second rank tensor, the piezoelectric constant must be written as a third rank tensor, but can be transformed to a second rank matrix.Reference Newnham9 As we are considering the replacement of PZT and of related materials, which are ferroelectrics and are converted to piezoelectrics by a poling process, ferroelectric crystal structures are becoming important.

Figure 1. (a) The direct piezoelectric effect provides an electric charge upon application of a mechanical stress, whereas (b) the converse piezoelectric effect describes the situation where strain develops under an applied electric field.
Domains in ferroelectrics (Figure 2) can be considered to be a form of twins that develop at the paraelectric/ferroelectric phase transition in order to reduce the elastic and electrostatic energies.Reference Tagantsev, Cross and Fousek10 Application of an electric field may lead to lattice extension (intrinsic contribution to the piezoelectric effect), domain-wall movement resulting in a strain contribution (extrinsic contribution to the piezoelectric effect), and phase transitions.Reference Damjanovic11 Consequently, the thermodynamics of the different crystal symmetries (Figure 3) is of paramount importance for understanding and designing piezoelectric materials. In particular, the anisotropic flattening of the free energy along nonpolar directions is key for good piezoelectric properties, as it facilitates easy rotation of the polarization vector.Reference Damjanovic11,Reference Rossetti, Khachaturyan, Akcay and Ni12 This important topic, contrasting piezoelectric mechanisms between PZT-related materials and new lead-free compositions, is covered in the article by Damjanovic and Rossetti in this issue.Reference Damjanovic and Rossetti13

Figure 2. Scanning electron microscope micrograph of an etched surface of (K, Na)0.94Li0.6NbO3 that features a complex domain structure in the polycrystalline grain ensemble. Courtesy: J.L. Zhang, Shandong University.

Figure 3. The cubic high-temperature perovskite phase transforming into one or several of the low-temperature phases: rhombohedral, orthorhombic, and tetragonal.Reference Jaffe, Cook and Jaffe4 Lead (barium, calcium, bismuth, potassium, or sodium in the lead-free replacements) occupies the A-site, while titanium, zirconium, niobium, tantalum or iron are on the B-site.
Note that domain wall movement, as well as phase transformations, are strongly hysteretic processes, which may lead to energy dissipation in the material. A temperature increase may then prompt thermal runaway and limit material performance. Hence, an important distinction in piezoceramics is between soft (high strain, high losses) and hard (low strain, low losses) piezoceramics.Reference Uchino14 A further classification stems from the pertinent applied field. Piezoelectric properties under low driving field (small signal properties) and under high driving field (large signal properties) are relevant for different applications.
Lead-free material options
The endeavor to find new lead-free perovskites has mostly focused on mimicking PZT. In PZT, a so-called morphotropic phase boundary (MPB) forms and provides properties mostly independent of temperature. This MPB features changes in phase structure with just composition and not temperature. Elements with high ionic polarizability, low cost,Reference Rödel, Jo, Seifert, Anton, Granzow and Damjanovic7 and low environmental impactReference Ibn-Mohammed, Koh, Reaney, Acquaye, Wang, Taylor and Genovese15 are required. Due to the similar electronic structure of bismuth and lead with the lone 2s-electron pair facilitating hybridization with oxygen, sodium-bismuth-titanate-based (NBT) materials have been contemplated since 1991.Reference Takenaka, Maruyama and Sakata16 These materials are typically relaxors and offer complex phase diagrams.Reference Ma, Guo, Beckman and Tan17 Consequently, dedicated studies of in situ phase transitions using scattering techniques such as neutron and synchrotron diffraction played an important role in elaborating structure–property relationships.Reference Ma, Guo, Beckman and Tan17,Reference Daniels, Jo, Rödel and Jones18 The current understanding of lead-free relaxor-based piezoceramics is discussed in the article by Paterson et al. in this issue.Reference Paterson, Nagata, Tan, Daniels, Hinterstein, Ranjan, Groszewicz, Jo and Jones19
“Lead-free at last” was the title chosen by CrossReference Cross20 to accompany a paper by Saito et al.Reference Saito, Takao, Tani, Nonoyama, Takatori, Homma, Nagaya and Nakamura2 on the development of potassium sodium niobate (KNN)-based lead-free piezoceramics. These materials feature optimum properties at a polymorphic phase transition with attendant drawbacks of strong temperature dependence.Reference Hollenstein, Damjanovic and Setter21,Reference Zhang, Xia and Shrout22 More recent research has focused on chemical modifications to provide enhanced temperature stability of the piezoelectric properties.Reference Wang, Bando, Katsumata, Inaguma, Taniguchi and Itoh23 This approach and attendant materials are discussed by Wang et al.Reference Wang, Malič and Wu24 in their article in this issue.
Liu and RenReference Liu and Ren25 proposed an alternative developmental path to mimicking PZT in 2009, using barium titanate as the base material, doping it with calcium and zirconium, and thus furnishing it with a tricritical pointReference Liu and Ren25 or convergence region where the cubic, orthorhombic, tetragonal, and rhombohedral phases coexist.Reference Keeble, Benabdallah, Thomas, Maglione and Kreisel26 This material again features a complex phase structure with good properties, albeit limited by a low Curie temperature. In their article in this issue, Gao et al.Reference Gao, Ke, Acosta, Glaum and Ren27 discuss structure–property relationships for different compositions in the pseudobinary phase diagram Ba(Zr,Ti)O3–(Ba,Ca)TiO3. A new material class based on bismuth ferrite (BF) addresses the needs for high-temperature applications. While the first publications on bismuth ferrite-barium titanate (BF-BT) are already several years old,Reference Leontsev and Eitel28 there has been recent progress by a number of researchers. Most appealing is the promise for use of BF-BT at high temperatures, not only replacing PZT, but also considerably improving high-temperature capabilities.Reference Lee, Kim, Park, Kim, Song, Kim, Kim, Do and Jeong29 In a study by Lee et al.,Reference Lee, Kim, Park, Kim, Song, Kim, Kim, Do and Jeong29 excellent piezoelectric properties with a Curie temperature in excess of 400°C were reported when BF-BT with the addition of either Bi1.05GaO3 or Bi1.05(Zn0.5Ti0.5)O3 was sintered and then quenched. Recently, a correlation of the phase diagram of BF-BT with electrical properties was achieved.Reference Kim, Khanal, Nam, Fujii, Ueno, Moriyoshi, Kuroiwa and Wada30 Similarly, it was demonstrated that an air quenching treatment in BF-BT affords an improvement in piezoelectric properties through salient changes in atomic structure.Reference Calisir and Hall31
Figure 4 summarizes the evolution in output of publications and patents in the field of lead-free piezoceramics. Work by many researchers has led to a secondary effect (i.e., development of spin-off nonpiezoelectric applications). The new insight, notably of bismuth-based perovskites, has spawned new research fields into oxygen conductors,Reference Li, Pietrowski, De Souza, Zhang, Reaney, Cook, Kilner and Sinclair33 high-temperature dielectrics,Reference Dittmer, Jo, Damjanovic and Rödel34 and energy-storage materials.Reference Gao, Dong, Mao, Liu, Zhang, Yang, Cao and Wang35 Similarly, a greater understanding of niobate-based materials has yielded the development of new antiferroelectric niobates for energy storageReference Guo, Shimizu, Mizuno and Randall36 and for electrocalorics.Reference Koruza, Rožič, Cordoyiannis, Malič and Kutnjak37
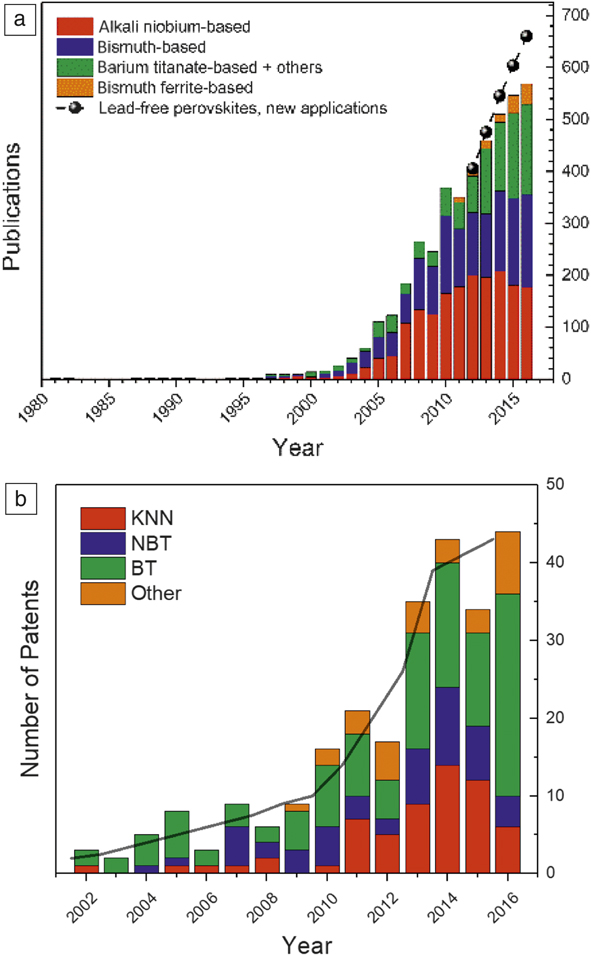
Figure 4. Evolution of lead-free piezoelectric research output in terms of (a) publications and (b) patents. This graph was compiled by searching for “lead-free” and “piezoceramics” in Web of Science32 and then checking each paper individually, whether it indeed discusses the replacement of lead zirconate titanate for piezoceramic applications. (a) The color bars represent the piezoelectric applications, while the black circles with the dashed line (lead-free perovskites, new applications) outline spin-off applications stemming from research into lead-free piezoceramics. KNN, potassium sodium niobate; NBT, sodium bismuth titanate; BT, barium titanate.
Future directions
While legislation in Europe and other regions has provided a strong impetus for further research, intense subsequent efforts have brought our scientific understanding close to the level of knowledge on lead-containing piezoceramics. This is particularly true for structural investigations of lead-free piezoceramics, where complementary in situ (temperature-, stress- and electric-field-dependent) neutron and synchrotron scattering techniques have provided a highly advanced understanding.Reference Levin and Reaney38 As a result, avenues have opened up for new materials with properties better than PZT for select applications.Reference Rödel, Webber, Dittmer, Jo, Kimura and Damjanovic39 For example, NBT-based materials have advantages over PZT in high-power applications,Reference Doshida, Shimizu, Mizuno and Tamura40 and KNN can be sintered with nickel for multilayer applications, with nickel providing high electromigration resistance and stability when subjected to high applied electric fields.Reference Kawada, Kimura, Higuchi and Takagi41 In the area of thin films, KNN materials offer excellent properties.Reference Shibata, Suenaga, Nomoto and Mishima42 Additionally, KNN-based ceramics are preferentially used for medical imaging transducers of 1–3 structured composites (fibrous inclusions in continuous matrix).Reference Shen, Li, Chen, Zhou and Shung43,Reference Li, Wang, Zhu, Cheng and Yao44 Our current knowledge of some of the primary piezoelectric properties (e.g., temperature-dependent piezoelectric properties) has reached a level sufficient for use in applications (Figure 545–90). In Figure 5, we see that BT and KNN exhibit a piezoelectric coefficient competitive to PZT at room temperature,Reference Shrout and Zhang3 while BF-BT has a higher Curie temperature than PZT.Reference Shrout and Zhang3 NBT-BT has advantages with high power applications and mechanical reliability (not covered with this graph). However, at this point in time, we also need to dedicate research efforts into better understanding secondary properties, such as electrical and mechanical properties, electrical fatigue, and machinability.Reference Koruza, Bell, Frömling, Webber, Wang and Rödel91 Based on the current level of product development, the article by Shibata et al. in this issue discusses the status of product transfer into applications.Reference Shibata, Wang, Tou and Koruza92 At the same time, new materials (e.g., based on bismuth ferrite)Reference Lee, Kim, Park, Kim, Song, Kim, Kim, Do and Jeong29 and new physical mechanisms are being discovered. In terms of new mechanisms, the opportunities to enhance depolarization temperature by either quenchingReference Mira, Nagata and Takenaka93 or using compositesReference Zhang, Pan, Guo, Liu, Ning, Chen, Lu, Yang, Chen, Zhang, Xing, Rödel, Cao and Chen94,Reference Riemer, Venkataraman, Jiang, Liu, Dietz, Stark, Groszewicz, Buntkowsky, Chen, Zhang, Rödel and Koruza95 and by hardening through hard second phasesReference Lalitha, Riemer, Koruza and Rödel96 seem particularly noteworthy.

Figure 5. A 3D figure showing d 33 as the z-axis, and the corresponding Curie temperature/depolarization temperature (T C/T d) as the x-axis. The y-axis may be assigned to different material systems. BT and KNN exhibit a piezoelectric coefficient competitive to lead zirconate titanate (PZT) at room temperature,Reference Shrout and Zhang3 while BF-BT has a higher Curie temperature than PZT. Note: d 33, piezoelectric coefficient; NBT, sodium bismuth titanate; KNN, potassium sodium niobate; BF, bismuth ferrite; BT barium titanate.Reference Saito, Takao, Tani, Nonoyama, Takatori, Homma, Nagaya and Nakamura2,Reference Takenaka, Maruyama and Sakata16,Reference Liu and Ren25,Reference Wu, Wu, Wu, Xiao, Zhu and Pennycook45–Reference Yang, Liu, Wei and Hou90
Acknowledgments
We thank J. Koruza for valuable discussions, and Y. Zhang and F. Weyland for their assistance in figure preparation. J.F.L. thanks the NSFC for support under Grant Nos. 51332002 and 51761135118.

Jürgen Rödel is a professor in materials science at TU Darmstadt, Germany, distinguished visiting professor at Tsinghua University, China, and specially appointed professor at Tokyo Institute of Technology, Japan. He received his MS degree in materials science from Universität Erlangen-Nürnberg, Germany, and his PhD degree from the University of California, Berkeley. His current interests include the development of lead-free piezoceramics and the concept of mechanically tuned conductivity. He has (co-)authored 300 publications. Rödel received the Deutsche Forschungsgemeinschaft Research Award for both young scientists (Heinz Maier-Leibnitz Prize) and for senior scientists (Gottfried Wilhelm Leibniz Prize). He is a member of the German National Academy of Science and Engineering. Rödel can be reached by email at [email protected].

Jing-Feng Li is the Cheung Kong Scholar Distinguished Professor at Tsinghua University, China. He received his PhD degree from Tohoku University, Japan, in 1991, and was an assistant professor and associate professor until joining Tsinghua University as a full professor in 2002. His research interests include piezoelectric and thermoelectric materials and microelectromechanical systems technologies. He has published two books and 400 papers, holds 33 patents, and received the Young Researcher Award from The Japan Institute of Metals and an outstanding young scientist grant from the National Natural Science Foundation of China (NSFC). He is editor-in-chief of the Journal of Materiomics. Li can be reached by email at [email protected].