Introduction
Conventionally, intelligence is seen as a property of individuals. However, it can also be a property of collectives (Wolpert and Kagan, Reference Wolpert and Kagan1999; Malone and Bernstein, Reference Malone and Bernstein2015). Examples include collective-decision-making by social insects (Mallon et al., Reference Mallon, Pratt and Franks2001), slime mould navigating mazes (Reid and Latty, Reference Reid and Latty2016), and even intelligent behaviour of individual cells and viruses which are themselves a collective of chemical processes. Humans are also intelligent, and our intelligence primarily derives from our social behaviour which is currently global in its reach. These examples serve to highlight the fact that intelligence, broadly construed, operates across diverse length and time scales. An open question is whether or not intelligence can operate at the planetary scale, and if so, how a transition to planetary-scale intelligence might occur and whether or not it has already occurred or is on our near-term horizon. Understanding the current state of intelligence on Earth and steering its future will require understanding how human and technological systems are integrated, and might display collective intelligence at a planetary scale.
The appearance of intelligence in the evolution of life may represent one of a series of major transitions in a planet's history (Leigh, Reference Leigh1995; Carter, Reference Carter2008). From Earth's evolution, we see that once intelligence manifests in the form of a global technological civilization, it has the power to reshape a planet in profound ways. On the one hand, a civilization's technological and energy-harvesting capacities may allow it to ‘engineer’ the world, creating new planetary-scale behaviours and functions allowing that civilization to survive over longer timescales than would otherwise be possible. Conversely, those same technological capacities may lead the species, or at least its global civilization, into a kind of suicide (i.e. nuclear weapons). They may also drive the planet into new states of its coupled systems (atmosphere, hydrosphere, etc.) which truncate the civilization's evolution (i.e. climate change: Rockström et al., Reference Rockström, Steffen, Noone, Persson, Chapin, Lambin, Lenton, Scheffer, Folke, Schellnhuber, Nykvist, De Wit, Hughes, van der Leeuw, Rodhe, Sörlin, Snyder, Costanza, Svedin, Falkenmark, Karlberg, Corell, Fabry, Hansen, Walker, Liverman, Richardson, Crutzen and Foley2009).Footnote 1
The consideration of intelligence in astrobiological studies has, however, tended to regard it solely as the property of one or more species which evolve on a planet and then go on to create a technological civilization rather than as a collective property existing in groups of organisms or societies. By implication, most astrobiologists do not view intelligence as a property of the biosphere, from which a ‘Technosphere’ might emerge as an evolutionary stage of global intelligence. This perspective is implicit in the Drake Equation (Shklovskii and Sagan, Reference Shklovskii and Sagan1966), through the terms f i and f c (the fractional appearance on habitable zone exoplanets of intelligent species and communicating civilizations respectively), where it is assumed that intelligence refers to individual members of a species and not necessarily their collective actions. Thus, one sees extended debates in the literature concerning the possibility of ‘convergent evolution’ by which intelligence may, or may not, be inevitably selected for in species via Darwinian processes (Wallace, Reference Wallace1903; Simpson, Reference Simpson1964; Mayr, Reference Mayr1995; Sagan, Reference Sagan1995; Lineweaver, Reference Lineweaver, Seckbach and Walsh2008). In this paper, however, we wish to broaden the view of intelligence by taking a planetary view of its appearance and effect. Here, we consider the ways in which the appearance of technological intelligence may represent a kind of planetary scale transition. In this way, it might be seen not as something which happens on a planet but to a planet. Our approach follows the recognition among researchers that the correct scale to understand key aspects of life and evolution is planetary, as opposed to the traditional focus on individual species (Margulis and Sagan, Reference Margulis and Sagan1986).
Thus, our purpose is to introduce, and explore the consequences of the idea of planetary intelligence. In the process, we hope to articulate ways in which the concept may prove useful for three distinct domains: Earth Systems and Exoplanet studies; Anthropocene and Sustainability studies; the study of Technosignatures and the Search for Extraterrestrial Intelligence (SETI). We believe the concept of planetary intelligence holds promise in providing a framework for understanding possible paths of long-term inhabited planetary evolution that is both broad and deep. Most important, it may ultimately help unite disparate perspectives into a single explanatory paradigm for the transitions in the Earth-system observed in the past, with what we are experiencing now and will experience in the future evolution of the Earth.
Planetary intelligence: definitions and uses
Our explicit definition of planetary intelligence is the acquisition and application of collective knowledge, operating at a planetary scale, which is integrated into the function of coupled planetary systems. One nascent example would be the global response to the planetary-scale crisis of ozonosphere erosion by CFCs. Another, still very much a work in progress, could be a global response to the crisis of anthropogenic global warming. However, we call these examples ‘nascent’ because, while they involve a global coordinated response to a potential existential threat, the decision-making is at the level of localized activities of individuals and governments.
As we will describe, a transition to global planetary intelligence should include a kind of intelligence that is more than the aggregate sum of the localized activities of life on smaller scales. We are interested in properties that exist at the scale of biospheres and/or technospheres (where technospheres are the aggregate planetary activity of technology; Herrmann-Pilath, Reference Herrmann-Pillath2018), and in their coupling to other planetary systems (e.g. geospheres), that are not apparent in individual organisms and subsystems comprising a biosphere or technosphere. Thus, the cognitive activity we are interested in must operate via feedback loops that are global in scale, coordination and operation. The concept of ‘human computation’ is one relevant example. Human computation includes examples where humans are computational elements in information processing systems, such as crowd-sourced activities like wiki editing or human-assisted AI (Michelucci et al., Reference Michelucci, Shanley, Dickinson and Hirsh2015). In addition, by defining planetary intelligence in terms of cognitive activity – i.e. in terms of knowledge that is only apparent at a global scale – we are explicitly broadening our view of technological intelligence beyond species that can reason or build tools in the traditional sense. We note that terms such as ‘knowledge’ and ‘cognition’ are usually reserved to describe individuals, but it is exactly our goal to push these concepts and determine in what sense they can apply to planetary-scale processes. We will clarify these points in the sections that follow.
There are successive distinct domains where we wish to explore the operation, and effect, of planetary intelligence (Fig. 1). We will argue that each relates to a different, but successive, phase of planetary evolution.
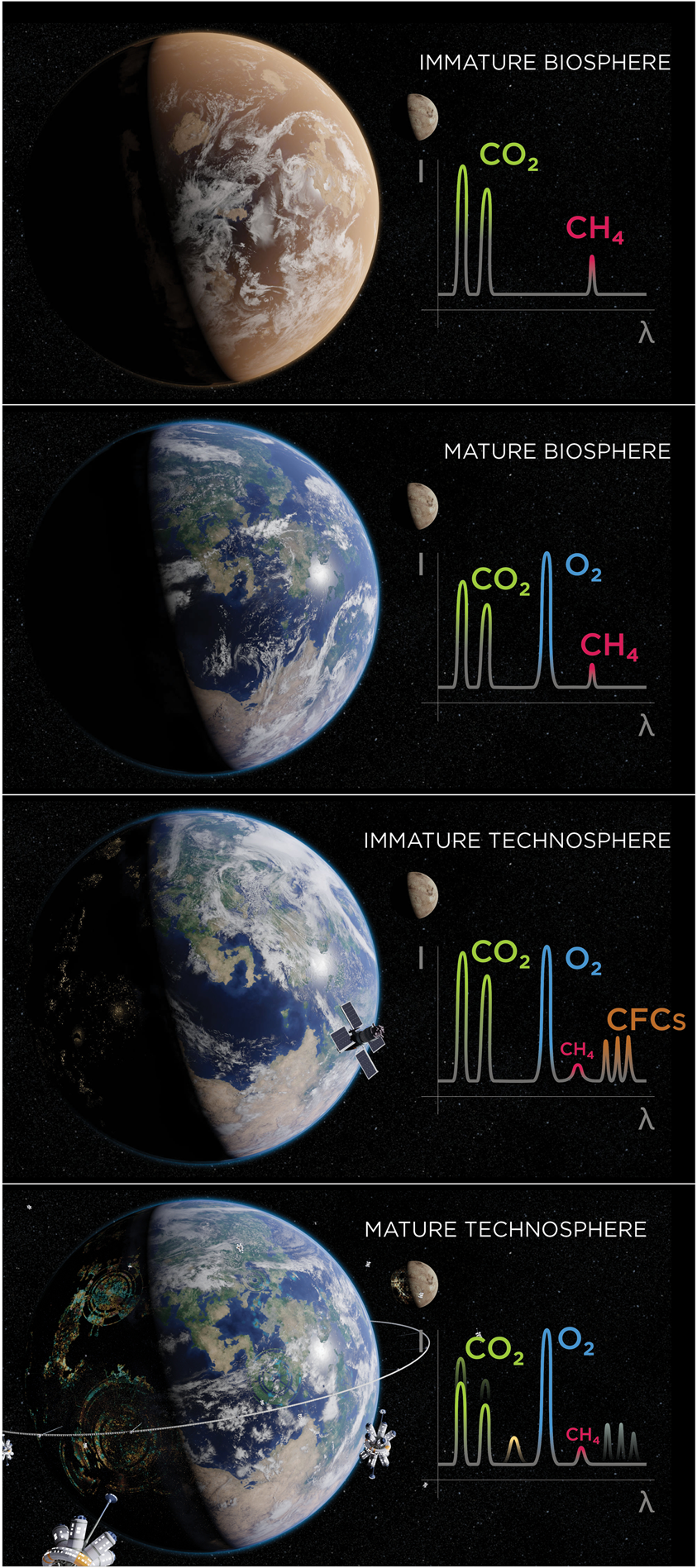
Fig. 1. Four possible domains of planetary intelligence. (a) On a planet with an immature biosphere (such as the Earth during the Archean Eon) there are insufficient feedback loops between life and geophysical coupled systems to exert strong co-evolution. (b) On a planet with a mature biosphere (such as Earth after the Proterozoic) the biosphere exerts strong forcing on the geophysical state establishing full co-evolution of the entire system. This feedback may provide some degree of long-term stabilizing (i.e. Gaian) modulations for the full system. (c) On a planet with an immature Technosphere (represented by the current Anthropocene Earth) feedbacks from technological activity produce strong enough forcing on the coupled planetary system to drive it into new dynamical states. These forcings however are unconstrained by intention relative to the health of the civilization producing the technology. (d) On a planet with a mature Technosphere, feedback loops between technological activity and biogeochemical and biogeophysical states have been intentionally modified to ensure maximum stability and productivity of the full system. Alongside each planetary image, we show a schematic atmospheric spectrum. An immature biosphere would show an atmosphere mostly in equilibrium dominated perhaps by CO2. In a mature biosphere life would have changed atmospheric chemistry leading to a highly non-equilibrium state such as perhaps high concentrations of O2. In an immature Technosphere new ‘pollutant’ species appear, such as CFCs, while industrial activities such as combustion may alter the abundance of other pre-existing gases like CO2 and methane. In a mature Technosphere all atmospheric constituents may have their concentrations modified to produce long-term stable and productive states for the full (civilization + biosphere) system. This is represented via a range of possible peaks for different constituents.
First, we will examine whether it is possible to consider intelligence, or some form of cognition, operating on a planetary scale even on those worlds without a planetary-scale technological species (Fig. 1(a) and (b)). This would require some form of collective cognition to have been a functional part of the biosphere for considerably longer than the relatively short tenure of human intelligence on Earth. If true, then the inherently global nature of the complex, networked feedbacks which occur in the biosphere may itself imply the operation of an ancestral planetary intelligence.
Second, we wish to consider whether the changes humans have been introducing to the planet through our industrial activities – the changes marking the ‘Anthropocene’ geological epoch (Crutzen, Reference Crutzen, Steffen, Jäger, Carson and Bradshaw2002; Steffen et al., Reference Steffen, Richardson, Rockström, Cornell, Fetzer, Bennett, Biggs, Carpenter, de Vries, de Wit, Folke, Gerten, Heinke, Mace, Persson, Ramanathan, Reyers and Sörlin2015) – may be understood as a transition in both the kind and level of global cognitive activity. Here, we are interested in the emergence of networks of processes that originate with human agency but become active and autonomously operate on levels beyond individuals. Thus, we will consider the idea of an emerging technosphere and its place in the Anthropocene (Fig. 1(c), Haff, Reference Haff2014a, Reference Haff2014b).
A focus on the Anthropocene allows us to assess the sustainability requirements for a long-lived industrial planetary civilization through the lens of planetary intelligence. Many current threats to sustainability are characterized by inadvertent planetary-scale changes in the environment. These are caused by our aggregate activities being unguided by an awareness of their global scale consequences (Grinspoon, Reference Grinspoon2016). It is not hard to argue that the long-term survival of our, or any global scale ‘project of civilization’ will require a fundamentally different mode of planetary-scale behaviour in which knowledge of planetary-scale impacts feeds back on, and modulates, behaviour in an intentional loop (e.g. perhaps mediated by artificial intelligence as our systems become increasingly integrated). This means we will need to consider the question of timescales within such feedback loops and also the scale at which decisions are made.
We note that decisions favouring the sustainability of collectives may not be the same as the preferences favoured by individuals. A clear but simple example in social choice theory is Arrow's Impossibility Theorem. Arrow's theorem demonstrates how, based on a simple set of reasonable assumptions, there is no possible way to rank the preferences of choices made by individuals into a ranked set of preferences for a collective (Arrow, Reference Arrow1950). That is, a collectives' rankings among a set of choices will not reflect that of its individual members in any procedural way.
The idea of planetary-scale collective cognition brings with it the question: would planetary behaviour dominated by stabilizing feedback between awareness and consequences represent a new type, or new level of planetary intelligence? If so, then our concept also takes on an aspirational quality. A deeper understanding of the transition to this mode could be useful for the project of building a sustainable global civilization (United Nations, 2015).
Finally, we wish to generalize these questions beyond the singular example of terrestrial history by asking whether planetary intelligence is likely to be a property of some (or perhaps most) inhabited worlds elsewhere in the universe, or at least the long-lived ones we are most likely to remotely detect (Fig. 1(d)). This implies that past, current and potential future transitions in Earth's history may have counterparts on other planets. Work on the ‘Astrobiology of the Anthropocene’ (Haqq-Misra and Baum, Reference Haqq-Misra and Baum2009; Frank and Sullivan, Reference Frank and Sullivan2014; Frank et al., Reference Frank, Carroll-Nellenback, Alberti and Kleidon2017, Reference Frank, Carroll-Nellenback, Alberti and Kleidon2018; Mullan and Haqq-Misra, Reference Mullan and Haqq-Misra2019) has already indicated that technological civilizations engaging in large-scale energy harvesting could trigger strong climate-changing feedbacks. The transition to long-term sustainable forms of such civilizations (if such a thing is possible) may have general, generic features which themselves involve transitions in planetary intelligence (Grinspoon, Reference Grinspoon2016). This line of inquiry can help us to both reflect upon terrestrial evolution from a less parochial perspective and formulate potential paths and states for planetary scale cognition on other planets. Such an effort may also be useful in deriving new observable diagnostics for ‘exo-civilizations’ by articulating characteristics of technological civilizations which can be detected from a distance (aka ‘technosignatures’). Thus, a characterization of planetary intelligence and its role in planetary evolution may be particularly useful for technosignature studies which currently represent a new and highly active direction in astrobiology and SETI (Genio and Wright, Reference Genio and Wright2018; Wright et al., Reference Wright2020).
Historical preliminaries: biosphere, Noosphere and Gaia
Consideration of planetary scale cognitive activity goes back to the formative development of biogeochemistry, Earth Systems' Science and Astrobiology. Indeed, the modern concept of the biosphere can be traced to the work of Vernadsky, the founder of both geochemistry and biogeochemistry (Vernadsky, Reference Vernadsky1998). It was Vernadsky who saw that the aggregate activity of life on Earth must be considered part of a system – the biosphere – which strongly couples to the other planetary systems: atmosphere, hydrosphere, cryosphere and lithosphere. In his view, this coupling was driven by the thermodynamics of free-energy gradients (Kleidon, Reference Kleidon2010). As Vernadsky wrote,
‘Activated by radiation, the matter of the biosphere collects and redistributes solar energy and converts it ultimately into free energy capable of doing work on Earth. A new character is imparted to the planet by this powerful cosmic force. The radiations that pour upon the Earth cause the biosphere to take on properties unknown to lifeless planetary surfaces, and thus transform the face of the Earth.’
For Vernadsky, the biosphere was an emergent phenomenon that appeared with, and evolved in tandem with the diversity of individual species. Indeed, the evolution of such species could only be fully accounted for in the context of the wider biosphere. But this emergence, he argued, always involved some degree of cognitive or ‘cultural’ activity.
After developing the concept of the biosphere, Vernadsky went on to explore the concept of the Noosphere (‘noos’ being Greek for Mind). Unlike Teilhard de Chardin's explicitly theological version of the idea (Teilhard de Chardin, Reference Teilhard de Chardin1959), for Vernadsky the Noosphere was an emergent shell of influence based on the totality of what he called ‘cultural biogeochemical energy’. In using the term ‘culture’, Vernadsky meant collective cognitive activity. He held that such activity had always been present in the biosphere from microbes to mammals. But, he argued, the collective cognitive activity in these species, and hence in the biosphere, was insignificant in both measure and impact until the development of Homo Sapiens' scientific and industrial activity.
While Vernadsky thought that ‘cultural biogeochemical energy’ was a minor player in the biosphere until recently, Lynn Margulis had her own conception of the idea and believed it played a larger role in planetary evolution via the Gaia Theory she famously developed along with James Lovelock. The Gaia Hypothesis, as first developed by Lovelock (Reference Lovelock1984) held that Earth's life was able to maintain global conditions, such as average temperature, within a range that kept the planet habitable (Lovelock, Reference Lovelock1984). Lovelock argued this would occur through negative feedbacks between life and planetary geochemistry. These feedbacks would act to keep perturbations in global conditions in check. What Margulis brought to the collaboration was a focus on the remarkable capacities of microbes to serve as drivers for Gaian feedbacks (Margulis and Lovelock, Reference Margulis and Lovelock1997).
What matters for our concerns is that through her research on evolutionary cooperation (as opposed to competition), Margulis saw the microbial domains as rich with a kind of ‘pre-intelligence’. As she wrote ‘the view of evolution as chronic bloody competition … dissolves before a new view of continual cooperation, strong interaction, and mutual dependence among life forms. Life did not take over the globe by combat, but by networking’ (Margulis and Sagan, Reference Margulis and Sagan1986, p. 122).
Gaia was not, however, to be seen as an organism. As Margulis wrote ‘[Gaia] is an emergent property of interaction among organisms, the spherical planet on which they reside, and an energy source, the Sun’ (Margulis and Sagan, Reference Margulis and Sagan1986). This concept of the emergence of a new planetary property from the networked activity of individual players was the central insight of what came to be called Gaia Theory. As Margulis later wrote ‘Gaia is the regulated surface of the planet incessantly creating new environments and new organisms…. Less a single live entity than a huge set of interacting ecosystems, the Earth as Gaian regulatory physiology transcends all individual organisms’ (Margulis and Sagan, Reference Margulis and Sagan1986, p. 120).
Gaia Theory was controversial when it was first proposed, particularly because some saw it as introducing a teleological principle into evolution (Dawkins, Reference Dawkins1982), while others argued that there was no means for it to arise through natural selection (Doolittle, Reference Doolittle2017). We note that there still remain questions concerning the evolution and efficacy of biospheric feedbacks for producing a full planetary homeostasis (Kirchner, Reference Kirchner2002). Recent work, however, points to evolutionary mechanisms that may select for the global-scale negative feedbacks which could maintain such a system (Lenton et al., Reference Lenton, Daines, Dyke, Nicholson, Wilkinson and Williams2018).
Still, the basic principles of Gaia Theory, effectively repackaged as ‘Earth Systems Science’, now represent the cornerstone of modern approaches to Earth's evolutionary history. What Earth Systems Science took from Gaia Theory was its recognition of the biosphere as a principal driver of planetary evolution, as well as the profound role of collective microbial activity in shaping critical biospheric feedbacks.
The concepts of Biosphere, Noosphere and Gaia – as developed by Vernadsky, Lovelock and Margulis – are the foundations for what follow in our argument. Taken as a whole, they represented the crucial first coherent attempts to recognize that life and its activity (including intelligence) may best be understood in their full planetary context. Our goal in what follows is to focus on the ways in which a theory of planetary intelligence may be pursued and prove useful.
Theoretical preliminaries
In this section, we provide a brief overview of the conceptual tools needed to develop a functioning theory of planetary intelligence. We note that this list is neither exclusive nor exhaustive but represents a possible set of ideas and approaches that could allow properly articulating a theory of intelligence as a planetary scale process. Below we outline five possible properties coupled planetary systems should possess to be considered a world displaying planetary intelligence (see also Table 1 and Fig. 2).
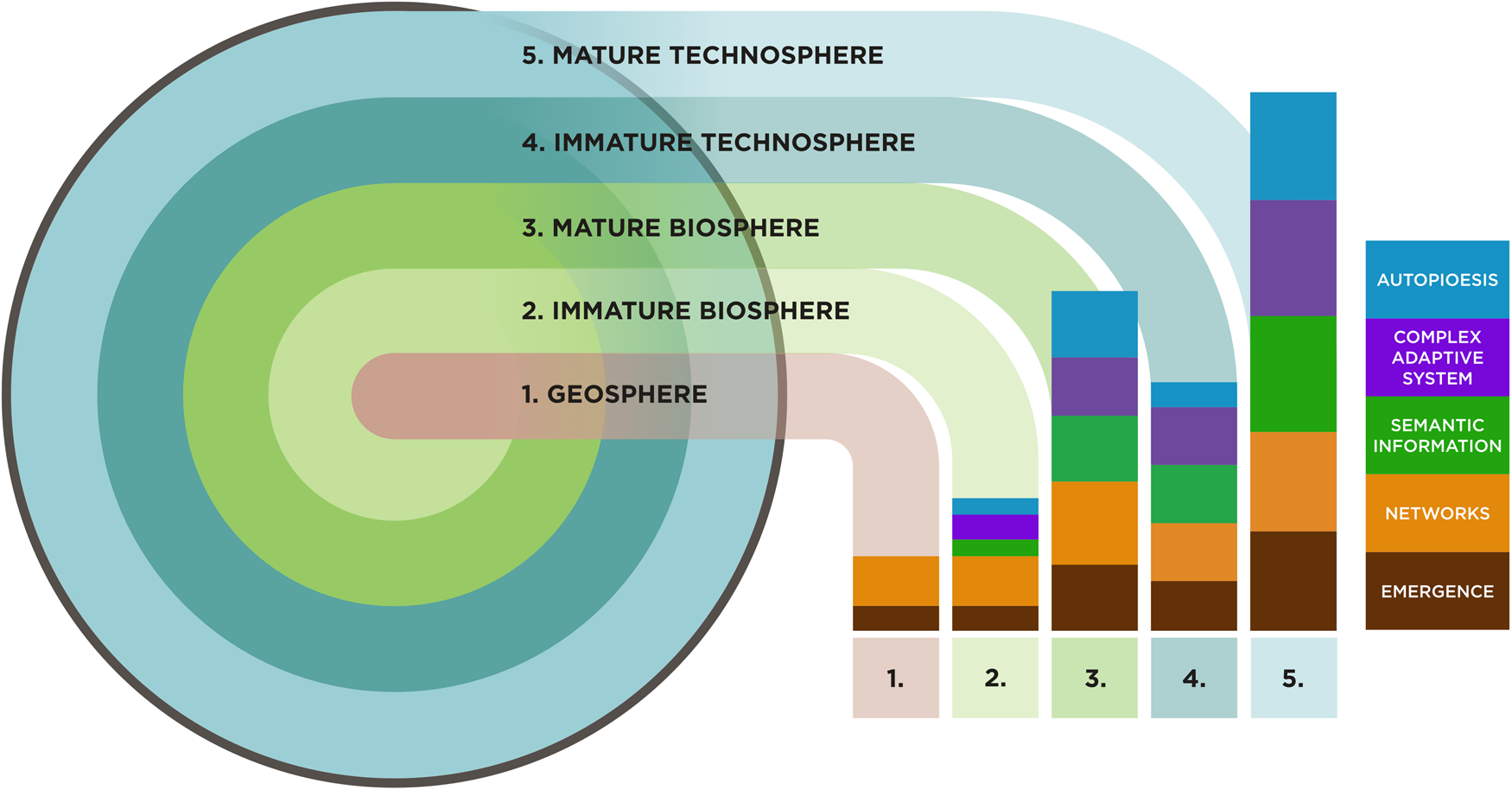
Fig. 2. Schematic representation of the evolution of coupled planetary systems in terms of degrees of planetary intelligence. We propose five possible properties required for a world to show cognitive activity operating across planetary scales (i.e. planetary intelligence). These are: (1) emergence, (2) dynamics of networks, (3) networks of semantic information, (4) appearance of complex adaptive systems, (5) autopoiesis. Different degrees of these properties appear as a world evolves from abiotic (geosphere) to biotic (biosphere) to technologic (technosphere). While the extent of each property shown in the histogram to the right is meant to be schematic, they represent a proposed evolutionary trajectory whereby a planet develops greater or lesser degrees of self-organizing and self-sustaining complexity. Thus, in the path from an abiotic world to one with a mature biosphere, the evolution of life pushes the planet from one which could not be described as a global complex adaptive system and did not exhibit autopoiesis to one in which those properties are both present and robust. Likewise, an immature technosphere actually shows lower degrees of planetary intelligence than a mature biosphere because key properties such as autopoietic sustainability have been reduced.
Table 1. Properties of planetary intelligence

Emergence
Ever since Erwin Schrodinger's essential book ‘What Is Life’ popularized the need to find the underlying physical principles which make living systems different from non-living ones (Schrodinger, Reference Schrodinger2012), researchers have attempted to find them. The hope has always been to find the first principle ‘laws of life’ similar to what has been found for fundamental laws of nature in other areas of physics. However, 70 years after the publication of ‘What Is Life’, no such foundational laws have been found. For some researchers, like Stuart Kaufmann, laws cannot be found because life, and its evolutionary processes, is fundamentally non-ergodic (Kauffman, Reference Kauffman2019). This view implies that biological systems do not explore all available phase space volumes (perhaps because the phase space volume is too large at the physical scale of chemistry or other evolutionary processes), but instead chart contingent paths through them. For Kauffman and others, life is an emergent property of the physiochemical systems from which it is constructed.
A standard view of emergence is to say, ‘the whole is greater than the parts’, such that properties and behaviours at collective scales cannot be predicted from, or reduced to, consideration of the parts alone. While emergence is most often regarded as a property of complex systems, e.g. biological and technological, it is also apparent in physics. Phillip Andersen, a Nobel Laureate in Physics for his work on condensed matter, famously wrote in an essay titled ‘More is Different’ (Anderson, Reference Anderson1972) that ‘The ability to reduce everything to simple fundamental laws does not imply the ability to start from those laws and reconstruct the universe’. It is also important to note, however, that emergent properties are not antagonistic to the reductionist view: in fact, it is by virtue of the fact that reductionism is possible that we can observe emergent properties at all.
It is also noteworthy that emergence is often associated with some degree of top-down causation where the emergent system creates modes of behaviour in its subsystems that would not be possible without the new and previously unpredicted higher-level rules (Ellis et al., Reference Ellis, Noble and O'Connor2012).
Thus, planetary intelligence, in the mode presupposed by Margulis, Vernandsky and others would necessarily be an emergent, collective property of the subsystems comprising the biosphere, that in turn induces new modes of behaviour on individual parts (e.g. organisms). Importantly, this implies by extension that life is not a scale-specific phenomenon, but instead one that emerges from chemistry and drives the organization of matter from the properties of cells to the planetary scale. The natural boundary for these processes is, therefore, planetary. Our suggestion is that intelligence, as the mechanism that controls the function, decision-making and seeming goal-directedness of many living processes is also not scale-specific, and is a general phenomenon that operates even at the planetary scale.
Information and networks
The view of life as an emergent phenomenon does not, however, imply that ‘law-like’ general principles for life cannot be found. The ability to articulate such law-like patterns is particularly important for an effort to use the properties of Earth's biosphere to understand life on other worlds (Walker et al., Reference Walker, Bains, Cronin, DasSarma, Danielache, Domagal-Goldman, Kacar, Kiang, Lenardic, Reinhard, Moore, Schwieterman, Shkolnik and Smith2018). In this pursuit, we regard it is essential to recognize that life involves a critical new quantity/property which non-living systems do not: the active use of information (Walker et al., Reference Walker, Kim and Davies2016). Information flows appear in living systems from cells to ecosystems to cities, and also downward in the form of networks of connection that constrain the behaviour and function between system components and subsystems. A perspective focusing on networks and information flow offers the possibility for developing a more general approach to understanding how law-like behaviours appear (emerge) in living systems. For example, studies of the biochemical networks at three levels of scale (cells, ecosystems and the biosphere) reveal a network structure that is common across scales of biological organization, including individuals and communities, and is distinct from random networks (Walker et al., Reference Walker, Kim and Davies2016; Kim et al., Reference Kim, Smith, Mathis, Raymond and Walker2019). This implies deeper levels of network structure in living systems than has been understood so far. This should be the case as these properties are now known to be universal across biochemical networks, they depend on size (i.e. the number of compounds which are nodes in the network), and they do not depend on the scale of organization. The emergence of intelligence operating on the scale of planetary behaviour/function would best be described via information flowing through the technosphere's/biosphere's geochemical and geophysical networks (Fig. 3), which can take different forms including processes at higher-scales constraining and determining the behaviour of lower-level entities (e.g. as happens in social systems, where our decisions are dependent on cultural and societal context).
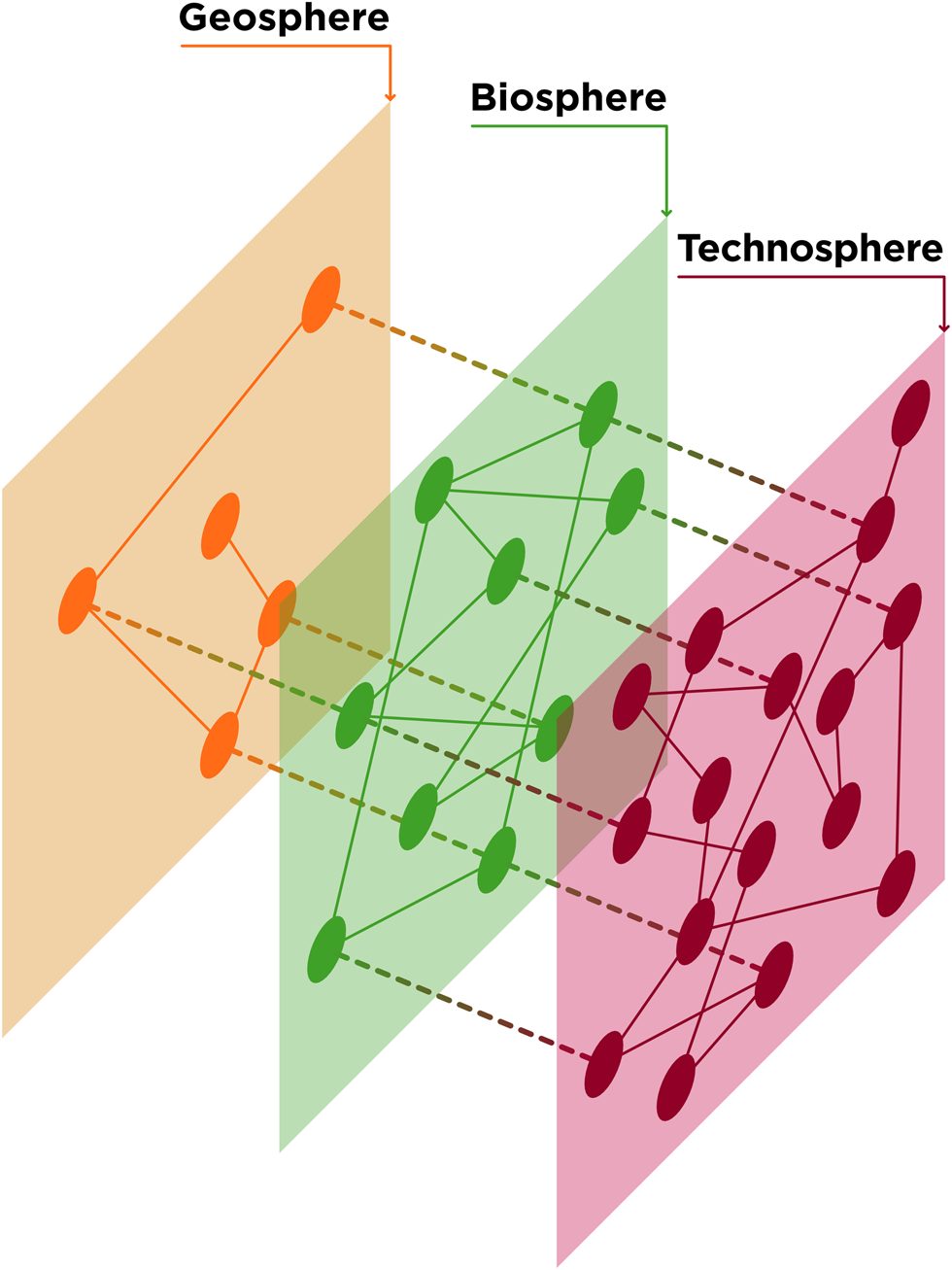
Fig. 3. Multi-level networks as a property of planetary scale operation of intelligence. Each layer of the coupled planetary systems constitutes its own network of chemical and physical interactions. Specific nodes in each layer represent links connecting the layers. Thus, the geosphere contains chemical/physical networks associated with processes such as atmospheric circulation, evaporation, condensation and weathering. These are modified by the biosphere via additional networks of processes such as microbial chemical processing and leaf transpiration. The technosphere adds an additional layer of networked processes such as industrial scale agriculture, manufacturing byproducts and energy generation.
Semantic versus syntactic information
If information is organizing the biosphere, where and in what ways is that information used? Does the importance of information in generating life's form and function also imply the presence of agents and agency beyond that of individual intelligent organisms operating within the global network? At what levels of organization can agency be said to appear? Does such agency imply intelligence?
From the perspective of these questions, the definition of information must include not only the physical criteria proposed by Shannon, i.e. measures of noise in communication channels. Instead, the definition of information we are interested in must also focus on the role of meaning. In living systems, information always carries a semantic aspect – its meaning – even if it is something as simple as the direction of a nutrient gradient in chemotaxis (Wadhams and Armitage, Reference Wadhams and Armitage2004). The definition and dynamics of semantic information represents a growing research domain with many applications (Kolchinsky and Wolpert, Reference Kolchinsky and Wolpert2018). For example, these questions might be better cast in terms of causal structure rather than as ‘informational’ in the Shannon sense (Ay and Poloni, Reference Ay and Poloni2007). Many of these approaches would be well suited to our questions. Thus, a consideration of planetary intelligence would recognize the centrality of semantic information flows (as well as syntactic i.e. Shannon flows) or causal structure, across biospheric and technospheric networks.
Complex systems: boundaries and signals
Many kinds of networks appear in the Universe. Networks of thermonuclear reactions within stars, for example, give rise to the elements with their specific abundances. The networks associated with life however, from metabolisms to social hierarchies, often represent higher levels of self-regulated behaviour and form what are called Complex Adaptive Systems (CAS). A CAS can be defined as one composed of semi-autonomous agents who interact in interdependent ways to produce system-wide patterns or behaviours which then influence the behaviour of the agents. Indeed, many of the characteristics of planetary intelligence we have articulated above appear in definitions of CAS (Miller and Page, Reference Miller and Page2009).
When considering planetary intelligence as a CAS however we wish to ultilize John Holland's emphasis on the role of boundaries and signals in their operation (Holland, Reference Holland2012). Emergence establishes an upward and downward cascade of order in living systems (organelles, cells, organs, animal, community) and for Holland such systems were always characterized by a boundary that was self-establishing. Most importantly, a primary function of these boundaries was to recognize signals. The boundary has to know what to keep in/out and what to let in/out. Without such signal processing, the boundary is nothing more than an inanimate wall. Thus, information flows embedded with significance through self-maintained boundaries represent critical elements of life and its use of semantic information at different levels of organization. We expect that intelligence operating on planetary scales would represent a CAS and would express some of its functions through the creation of signal-sensitive boundaries at different levels of structure and function.
The autopoietic view
Thus far we have argued that to properly situate the question of planetary intelligence within the biospheres' emergent, networked interactions with the other coupled planetary systems, one must account for the ways in which life manifests and uses semantic information through the creation of an inside and an outside (a boundary). In addition, one must understand this manifestation of interiority given life's ability to maintain itself as a transient, low entropy system. To this end, we draw from work on life – and intelligence – as an autopoietic system (Maturana and Varela, Reference Maturana and Varela2012).
Autopoiesis means self-making or self-producing (Beer, Reference Beer2020). An autopoietic system is a network of processes that recursively depend on each other for their own generation and realization. From this perspective, life is an autonomous system that is organizationally closed. Organizational closure means the individuality of a biological system is created by the system itself. Thus, living systems have the capacity to maintain their identities in spite of fluctuations and perturbations coming from without. In this view, life is a process of maintaining an identity from within. However, this unity is never static and can never be ‘durably secured’ (Thompson, Reference Thompson2010). The organism must always operationally reconstitute itself by maintaining the physiochemical and information processing capacities that constitute its own ‘going-on’. It must continually create the conditions for its own existence via metabolism. If the dynamic falters or stops, the organism dies.
We note that this dynamic entails information flows in both the Shannon and semantic/cognitive sense. Information flows emerge as meaningful in autopoiesis. The sugar gradient in chemotaxis may be conceived of as containing information (it has a computable slope) but that information is not meaningful without the presence of the cell sensing and responding to the gradient and then moving up the slope. It is in this way that ‘knowing’ can be said to appear with the establishment of an autopoietic system and this, in turn, allows the idea to become a potentially productive path for understanding the emergence of intelligence on planetary scales.
We note that autopoiesis figured strongly in Margulis' exposition of the structure and function of Gaia. As she wrote ‘Living systems, from their smallest limits as bacterial cells to their largest extent as Gaia, are autopoietic: they self-maintain’ (Margulis and Sagan, Reference Margulis, Sagan and Thomas1986).
Finally, we explicitly note that many other ideas on the nature of life and intelligence could prove relevant to questions of emergence, life and intelligence on planetary scales. For example, Kauffman's concept of autocatalytic agents, proposed to understand the emergence of life, has been generalized to understand the creation of CAS (Kauffman, Reference Kauffman, Barrow, Davies and Harper2004). Yet another perspective on the evolution and nature of cognitive activity comes from Integrated Information Theory (IIT) which holds that consciousness emerges within networks of sufficient complexity with the right connectivity (Tononi, Reference Tononi and Banks2009). However, we focus on the autopoietic view as it currently holds a descriptive completeness that will allow us to reach across the three domains of our inquiry: Biosphere, Anthropocene, Technosignatures/Exocivilizations.
In what follows we will explore the concept of planetary intelligence across a series of evolutionary domains. In particular, we are first interested in worlds possessing only a biosphere that make the transition from what we will call immature to mature. As we will see this transition involves the nature of the feedback networks between life and the non-living geospheres (atmosphere, hydrosphere and lithosphere). We will then explore a similar transition in the possible evolution of technospheres. In Fig. 2 we provide a schematic of how the properties of planetary intelligence described in this section might manifest themselves across these transitions. We will unpack the meaning of Fig. 2 in the sections that follow.
Planetary intelligence before technological species: biosphere networks
Once a species capable of constructing a technological civilization appears, intelligence by most definitions exists on a planet. As we will see, however, this does not imply it is meaningful to discuss the existence of a planetary intelligence as the dominant driver of planetary evolution in such a world. Life on Earth emerged almost 4 billion years ago. By 3 billion years ago, collectives of single-celled organisms existed in large enough quantities to begin affecting the coupled geophysical/geochemical systems (Lenton and Watson, Reference Lenton and Watson2011). The formation of methanogens, for example, is believed to have changed atmospheric chemistry sufficiently to alter the Earth's radiative properties and trigger the first global glaciation or ‘snowball Earth phase’. In addition, for the first two billion years of Earth's evolution, its atmosphere consisted primarily of N2 and CO2 with O2 acting only as a trace gas. It was the evolution of oxygenic photosynthesis by cyanobacteria that led to the atmosphere's Great Oxygenation Event (GOE) approximately 2.5 billion years ago (Catling, Reference Catling2014). The GOE made O2 abundant in Earth's biogeochemical networks with profound consequences such as allowing for far more energetic modes of metabolism (Lenton and Watson, Reference Lenton and Watson2011).
Microbes also play an essential role in Gaian and Earth Systems Science descriptions of planetary evolution through the establishment of feedback loops which maintain the planet in stable dynamic equilibria. Known and proposed examples of such feedbacks abound: climate regulation through biologically enhanced rock weathering (Zeebe and Caldeira, Reference Zeebe and Caldeira2008); the maintenance of O2 partial pressures below 30% through methane-producing microbes (Lenton and Watson, Reference Lenton and Watson2000; Berner et al., Reference Berner, Beerling, Dudley, Robinson and Wildman2003); climate regulation through cloud-albedo control linked to algal gas emissions (Charlson et al., Reference Charlson, Lovelock, Andreae and Warren1987); the biological transfer of selenium from the ocean to the land as dimethyl selenide (Watson and Liss, Reference Watson and Liss1998)
Given the critical role of microbes in establishing these feedback loops, when formulating questions of planetary intelligence one can first ask if microbes, or their communal networks, possess anything like cognition. In other words, do microbes or their collectives ‘know’ anything about the world, rather than just bumping into it? This leads us to ask what is meant by knowing or, more formally, to consider the nature of cognition across all forms of life. A succinct definition is given by Shettleworth (Reference Shettleworth1993) who sees cognition as ‘the mechanisms by which animals acquire, process, store, and act on information from the environment’. A more extensive definition is given by Lyon (Reference Lyon2015).
Biological cognition is the complex of sensory and other information-processing mechanisms an organism has for becoming familiar with, valuing and interacting with its environment in order to meet existential goals, the most basic of which are survival (growth or thriving) and reproduction.
There is now considerable evidence that bacteria exhibit a range of behaviours associated with cognition in the sense given above. Signal Transduction (ST), the most basic form of sense perception, is known to occur in bacteria in multiple forms allowing them to sense and respond to a wide array of environmental cues. Bacteria can also communicate through a process known as Auto-Induction where they stimulate changes in their genetic expression when certain environmental molecules reach threshold concentrations (Miller and Bassler, Reference Miller and Bassler2001). This is the basis of the much discussed process of bacterial quorum sensing where advantageous genetic changes in populations are induced at concentrations dependent on population density. Equally important was the discovery of rich social behaviours in species like Myxococcus xanthus (‘the primate of eubacteria’, Lyon, Reference Lyon2015) which has proven capable of structured, multi-dimensional swarming (Kaiser and Warrick, Reference Kaiser and Warrick2014), pack-like predation (Berleman and Kirby, Reference Berleman and Kirby2009), and the use of chemical cues to lure faster-moving prey (Shi and Zusman, Reference Shi and Zusman1993). Memory and learning, both bedrock conceptions of cognition, have also both been shown to be present in the bacterial toolkit of behaviours (Wolf et al., Reference Wolf, Fontaine-Bodin, Bischofs, Price, Keasling and Arkin2008).
From this perspective, there have been forms of cognitive activity (i.e. Vernadsky's cultural biogeochemical energy) on the planet for much longer than there have been animal nervous systems, and certainly far pre-dating the appearance of the genus homo. If the microbes which form planetary feedback loops can be said to collectively know things about their world then, perhaps, it may be possible and useful to ask if this knowing is integrated into higher scale, emergent behaviours which would represent planetary intelligence.
To see this, consider how the feedback loops which maintain Earth's O2 levels can be conceptualized (and modelled) as networks with information flow. Some habitable zone planets may also be capable of generating O2-rich atmospheres (Domagal-Goldman et al., Reference Domagal-Goldman, Segura, Claire, Robinson and Meadows2014) through a variety of processes in the atmosphere. Thus, O2 levels can vary due to purely geophysical/geochemical feedback loops. However, in the absence of a biosphere, these networks are not processing information in both the Shannon and semantic senses. On a planet without life, e.g. orbiting an M-star, O2 levels might be similar to those of an inhabited planet, but the O2 cannot act as a signal by geophysical/geochemical processes.
A biosphere, however, represents a complex network of feedback loops which can be seen as taking changing O2 levels as a signal. Such changes hold semantic information for the biosphere (Kolchinsky and Wolpert, Reference Kolchinsky and Wolpert2018) triggering responses that change the biospheric state. The presence of semantic information flows enables a biosphere to chart a contingent path through the available phase space of planetary states. Unique planetary states are selected that could not have been reached without its action. Perturbations in planetary conditions become significant and have meaning to the biosphere only within the context of the information represented by the existing state, which itself was reached through an evolutionary history. That is, like other biological systems at lower scales, we conjecture that the biosphere's evolution is ‘state-dependent’, with rules that emerge dependant on those states (Goldenfeld and Woese, Reference Goldenfeld and Woese2011; Adams et al., Reference Adams, Zenil, Davies and Walker2017). This is the dynamic we expect for planetary intelligence.
It is noteworthy that in today's biosphere there are semantic information flows, which act locally and yet can yield feedback and controls on larger scales. An obvious example is that information encoded in the arrangement of bases in a genome, which is minuscule in physical size compared to the planet, can nonetheless specify the control of metabolic pathways that shape global biogeochemical cycles.
In another example, arbuscular mycorrhizal fungi inhabit the root systems of 80% of land plant species (Simard et al., Reference Simard, Perry, Jones, Myrold, Durall and Molina1997). They are mutualistic symbionts that develop extensive, below-ground networks influencing uptakes and transfer of nutrients to their hosts. Because of their geographic extension, these networks may link contiguous plants to one another via their root systems. The global distribution of these symbioses is likely integral to understanding the present and future functioning of global scale biomes like forest ecosystems (Steidinger et al., Reference Steidinger, Crowther, Liang, Van Nuland, Werner, Reich, Nabuurs, de-Miguel, Zhou, Picard, Herault, Zhao, Zhang, Routh and Peay2019). Given their importance, and the apparent ability of these networks to direct nutrients to parts of the forest under stress, the question of self-recognition has been explored. Indeed, recent results show that the root systems of plants belonging to different species, genera and families may be connected by means of mycorrhizal networks, which can create indefinitely large numbers of below ground fungal linkages within plant communities (Giovannetti et al., Reference Giovannetti, Avio, Fortuna, Pellegrino, Sbrana and Strani2006). Thus, there may be pathways through which semantic information flows across these large-scale biomes. They, in turn, may be part of an emergent cascade to planetary scales of feedbacks and controls that could be considered cognitive in the autopoietic sense.
Finally let us consider the question of boundaries and signals. Before the GOE, O2 existed only as a trace gas in Earth's atmosphere. Through the collective action of cyanobacteria, the GOE was triggered and O2 became a principal component in Earth's biogeochemical networks. In terms of autopoiesis, one can argue that this led to the development of an ozone layer which became significant for the subsequent evolution of the biosphere. The thin band of atmosphere where ozone is maintained depends on the continued functioning of the biosphere. It may, perhaps, be seen as a simple boundary or photo-chemical membrane of the biosphere for which the signal is incoming sunlight. In addition, many planets have so-called ‘cold-traps’ in their atmospheres where the temperature switches from decreasing with height to increasing with height. On Earth, this temperature inversion occurs at a relatively low altitude, at the boundary between the troposphere and the stratosphere. Earth has not lost its oceans, as likely occurred on Venus, in part because rising water vapour condenses and rains back to the surface at the cold trap. The presence of oxygen in the atmosphere is a key reason for the location of the cold trap making it potentially another simple version of a signal-sensitive boundary that formed via the collective action of the planet's biota. While these examples are obviously highly speculative, they illustrate how the general principles we described in section ‘Theoretical Preliminaries’ may yield guidance in thinking about planetary intelligence.
Thus, we can imagine a transition in the evolution of a planet from one with an immature biosphere without strong networked feedbacks on the geospheres to a mature biosphere in which life becomes a dominant player in the evolution of the planet. Such a transition would be associated with the appearance of truly global feedbacks of semantic information, CAS behaviour and autopoiesis as shown schematically in Fig. 2. On Earth, this transition would have occurred in the Archean at its boundary with the Proterozoic.
Planetary intelligence with a technological species: generic Anthropocene
The development of agriculture after the last ice age, followed by the construction of cities – and the empires required to support them – were the initial stages in building a technological system that eventually reached across the planet. With the discovery and application of fossil fuels began an industrial age, which over just a few centuries rewired the Earth systems' coupled networks.
In 2002, Crutzen and Stoermer proposed that human-induced changes to these systems initiated a new geological epoch which they called the ‘Anthropocene’ (Crutzen, Reference Crutzen, Steffen, Jäger, Carson and Bradshaw2002). While some researchers question if the Anthropocene can be precisely defined via stratigraphy (Zalasiewicz, Reference Zalasiewicz2015), substantial evidence exists that Earth has already crossed a boundary where key measures show large-scale human imprints. As two examples, consider that more than 50% of the Earth's land surface area has been altered for human uses (Hooke and Martín-Duque, Reference Hooke and Martín-Duque2012; Zalasiewicz, Reference Zalasiewicz2015), and current anthropogenic flows of phosphorus are more than a factor of 5 above ‘natural’ rates (8 Tg P year−1 anthropogenic versus 1.1 Tg P year−1 natural).
One can, therefore, define the Anthropocene more generally as a new epoch in which human effects dominate many of the coupled Earth Systems. Indeed, recognition that human activities alter Earth's climate has prompted debate concerning ‘planetary boundaries’ (Lenton et al., Reference Lenton, Held, Kriegler, Hall, Lucht, Rahmstorf and Schellnhuber2008; Rockström et al., Reference Rockström, Steffen, Noone, Persson, Chapin, Lambin, Lenton, Scheffer, Folke, Schellnhuber, Nykvist, De Wit, Hughes, van der Leeuw, Rodhe, Sörlin, Snyder, Costanza, Svedin, Falkenmark, Karlberg, Corell, Fabry, Hansen, Walker, Liverman, Richardson, Crutzen and Foley2009; Barnosky et al., Reference Barnosky, Hadly, Bascompte, Berlow, Brown, Fortelius, Getz, Harte, Hastings, Marquet, Martinez, Mooers, Roopnarine, Vermeij, Williams, Gillespie, Kitzes, Marshall, Mindell, Revilla and Smith2012) which are limits in various quantities and processes required to keep the anthropogenic forcing (Steffen et al., Reference Steffen, Richardson, Rockström, Cornell, Fetzer, Bennett, Biggs, Carpenter, de Vries, de Wit, Folke, Gerten, Heinke, Mace, Persson, Ramanathan, Reyers and Sörlin2015) within ‘safe operating limits’.
Thus, by the beginning of the 21st century, the systems Homo sapiens had constructed were planetary. There were human beings on every landmass and our artefacts, from microscopic plastic debris to released fossil CO2, stretched from the deep ocean to the upper atmosphere, and even to the Moon and other planetary bodies. More importantly, as studies like those on planetary boundaries demonstrate, human industrial activity had become a force in the operation of the planetary system. We will return to the question of the timescales of this forcing, but here we note that the global impact of human activities requires both a level, and depth of organization that brings us back to our definitions of planetary intelligence.
Recall that we argued any form of planetary intelligence should be seen as the emergence of a planetary scale CAS which is itself a series of layered networks regulating the planetary state via flows of sematic information. There can be no doubt that aspects of this description fit our current technological, energy-intensive planetary civilization. A considerable literature has grown around the study of the networks which comprise human activity. In particular, the term ‘Technosphere’ (Herrmann-Pillath, Reference Herrmann-Pillath2018) has been used to explicitly denote the planetary character of human activity and influence, or more generally the activity of an intelligent species that constructs technology. Peter Haff, who first proposed the term, defines the Technosphere (Haff, Reference Haff2012, Reference Haff2014a, Reference Haff2014b) as ‘the interlinked set of communication, transportation, bureaucratic and other systems that act to metabolize fossil fuels and other energy resources’. This definition includes flows of material, energy and information. Additionally, by including bureaucratic (i.e. governance) networks in the definition, a Technosphere inherently includes semantic information.
The view of civilization as a CAS with various sublevels of structure is evidenced by proposals that these subdomains represent their own planetary systems, e.g. economic activity/organization is often referred to as an ‘Econosphere’ (Logan, Reference Logan2014). The use of the suffix ‘sphere’ in such work is an explicit acknowledgement that consideration of a given systems' structural and evolutionary characteristics can be considered as an explicitly planetary phenomenon.
There is, however, one important caveat in considering the Anthropocene as evidence for Earth currently existing in a state of active planetary intelligence. For all its reach, what Homo sapiens have constructed with our industrial civilizations appears inherently unstable. If we consider civilization as a Technosphere (human population plus technological support systems) coupled to the other planetary systems (biosphere, atmosphere, etc.), we can frame questions of stability in terms of these coupled systems' forcing and response times.
In Frank et al. (Reference Frank, Carroll-Nellenback, Alberti and Kleidon2018), a dynamical systems approach was applied to the interaction of an energy-harvesting civilization and the host planet from which that energy was harvested. This work showed that parameters associated with both the planet's sensitivity to forcing and the civilization's population growth/decline, as a result of energy harvesting, determined the long-term viability of coupled systems. The governing equations were highly abstracted and constituted a ‘toy’ model. A second study (Savitch et al., Reference Savitch, Frank, Carroll-Nellenback, Haqq-Misra, Kleidon and Alberti2021), which used an energy balance climate model, provided an explicit formalism for the forcing on the coupled planet-civilization which was described via a sensitivity parameter.

Here t growth and t climate are the characteristic times for planetary population growth and natural climate response. Their ratio, γ, can be defined in terms of: S climate, the climate sensitivity to doubling CO2; $P_{{\rm C}{\rm O}_2}$, the per-capita production of CO2; Nmax, the planet's maximum population (i.e. its carrying capacity); B nat, the natural birth rate without enhancements from technology; and ΔT the acceptable range of temperature change for civilization (related to death rate).
For γ > 1 the population grows and drives climate feedbacks thereby producing climate forcings at a faster rate than the planet's own internal mechanisms (i.e. processes like weathering, volcanism, etc.). Figure 4 shows one such model from Savitch et al., Reference Savitch, Frank, Carroll-Nellenback, Haqq-Misra, Kleidon and Alberti2021. As Fig. 4 shows, in this regime, the activities of the civilization push the planet into new climate states on timescales measured in a few generations. The global average temperature (T p) in these new states are beyond what the technosphere can handle (i.e T p > T o + ΔT where T o is the planetary temperature before the technosphere emerged). This leads to a rapid reduction in the population where ‘rapid’ can also be defined in terms of a few generations. If the die-off in the technosphere is large enough in a short enough time (again measured in terms of generations) then a complex technological civilization may not be capable of maintaining itself.

Fig. 4. Trajectories for population (green) and global mean temperature (orange) versus time from a coupled planet-civilization dynamical systems model (Savitch et al., Reference Savitch, Frank, Carroll-Nellenback, Haqq-Misra, Kleidon and Alberti2021). The model is run for an Earth analogue beginning with planetary conditions (atmospheric composition, etc.) at the year 1850 CE. The model tracks the civilizations' population growth including enhancements in birth rates due to energy harvesting from the planet as well as enhancements in the death rate due to climate changes driven by that energy use. A 1-D Energy Balance Model (EBM) is used to track changes in the global mean temperature. The model shows the development of a climate-driven ‘Anthropocene’ where the population's exponential growth (whose rate is determined by its technologically driven energy harvesting) is truncated by rising temperatures. The Anthropocene begins around 2800 CE in this model and within three centuries the population has declined by half.
While our current, early-Anthropocene phase displays key features of a planetary intelligence, e.g. an emergent CAS composed of multi-layered networks of semantic information flows, it appears to lack the critical characteristic of autopoietic self-maintenance. Recall that a system will be autopoietic if it is both self-creating and self-maintaining. Self-maintenance requires operational closure such that the system can create the processes and products that are themselves necessary for maintaining those processes and products, thus allowing the system to persist. But by driving the coupled Earth systems beyond their safe-operating boundaries (i.e. a Holocene climate state), early-Anthropocene human activity is threatening/degrading, rather than maintaining, these processes and products. Thus, we might consider the current Earth state as representing an ‘immature’ technosphere in which the full suite of properties we would associate with planetary intelligence have yet to emerge (Figs. 1 and 2).
The consideration of boundaries and signals is also useful for thinking about the early-Anthropocene as an immature technosphere relative to the properties of planetary intelligence. First, we note that it was a threat to the ozone layer via CFCs that drove an early and successful attempt at planetary regulation. This effort is of particular interest given that, as highlighted in section ‘Theoretical preliminaries’, the ozone layer may be an artefact of a biospheric cognitive CAS. Secondly, early efforts to construct a planetary asteroid defence can also be seen in terms of the planetary boundaries. Here asteroids larger than a certain size are the signal to which the boundary marking the planetary intelligence must respond. Thus, the astrophysical effort at finding such bodies marks an early attempt by human civilization to both build such a boundary and establish a working mature technosphere, which can then develop self-maintaining planetary intelligence.
From these perspectives, we see how the concept of planetary intelligence is both descriptive and proscriptive. Indeed, in considering our current predicament it can, perhaps, help to explicate the steps and states needed to go from an unstable early-Anthropocene civilization (γ > 1) to a stable, sustainable mature Anthropocene civilization (γ < 1). This possibility is the focus of the next section.
Planetary intelligence and long-lived civilizations
The long-term evolution of technological civilizations, no matter where they appear in the Universe, has been an enduring question in Astrobiological studies. The most famous attempt to categorize such evolution was the Kardeshev Scale (Cirkovic, Reference Cirkovic2015). Based solely on energy harvesting capacities, it classified civilizations on their ability to tap the full energy budget incident on a planet (Type I), generated by the planet's host star (Type II) or by the sum of all stellar energy in the host galaxy (Type III). Human civilization's entry into the Anthropocene, and the potential existential threat it represents, demonstrates that energy-harvesting capacities alone are not enough to meaningfully characterize the evolution of technospheres. One lesson of the Anthropocene appears to be the importance of developing global regulatory feedback loops across the whole of the host world's coupled planetary systems. In this way, it is useful to consider the establishment of mature planetary intelligence as a potential necessary condition for the existence of long-lived technospheres.
In Frank et al. (Reference Frank, Carroll-Nellenback, Alberti and Kleidon2018), a classification for planets was proposed based on the degree of thermodynamic complexity in the coupled systems (Frank et al., Reference Frank, Carroll-Nellenback, Alberti and Kleidon2017). A Class I world, like Mercury, has no atmosphere and so can only reradiate low entropy incoming stellar flux as a higher entropy, lower temperature blackbody. With the addition of an atmosphere, Class II worlds can tap free-energy gradients generated by incoming solar radiation (i.e. temperature differences between the surface and atmosphere) to do work and generate dissipative structures/processes like convective circulation and evaporation/condensation cycles. Class III worlds include ‘thin’ biospheres which can locally modify conditions tapping free energy (such as chemical gradients) generated by abiotic processes. On Class IV worlds, the biosphere is ‘thick’ (or ‘mature’) meaning it generates a complex network of processes that exert strong global forcings on the other planetary systems (Fig. 2). Higher levels of dissipation, and therefore disequilibrium, are expected in going from Class I to Class IV worlds. Such a ‘run’ of disequilibrium is, in fact, seen in the solar system in going from Venus, Mars and Titan to Earth (Krissansen-Totton et al., Reference Krissansen-Totton, Bergsman and Catling2016; Frank et al., Reference Frank, Carroll-Nellenback, Alberti and Kleidon2017). Models also show that in moving from the Archean (a thin immature biosphere) to the current thick, mature biosphere, the Earth System has also seen a temporal rise in disequilibrium (Krissansen-Totton et al., Reference Krissansen-Totton, Olson and Catling2018).
The final classification in this scheme was a Class V planet which included a civilization (i.e. a technosphere, Figs. 1 and 2), that had come into a long-term, stable relationship with the other coupled systems. By extending the properties/features of the other classes to a world with a technosphere, Frank et al. (Reference Frank, Carroll-Nellenback, Alberti and Kleidon2018) sought to articulate the characteristics of energy-intensive civilizations that had reached biogeochemical and biogeophysical steady-states with their host worlds (i.e. mature technospheres). The deployment of planetary scale cooperative dynamics with the biosphere was imagined to be one aspect of achieving these states. One example considered was the large-scale ‘greening’ of deserts to make the biosphere more diverse and productive for its own functioning (Becker et al., Reference Becker, Wulfmeyer, Berger, Gebel and Münch2013; Bowring et al., Reference Bowring, Miller, Ganzeveld and Kleidon2013).
From the perspective of the goals of this paper, the generation of robust and stable steady-states between technospheres, biospheres and the other coupled systems would involve the clearest example of planetary intelligence. Here the collective agency of the individual components of the technosphere and biosphere are marshalled for explicitly planetary scale goals. Like the early stage Anthropocene, a Class V world includes a technosphere giving it the first set of characteristics in our definition of planetary intelligence: emergence; networks of semantic information flow; the operation of the technosphere as a CAS including the functioning of signal-sensitive boundaries. Unlike the early stage Anthropocene (which we have argued is an immature Technosphere) the mature technosphere on a Class V planet would have achieved operational closure by deliberately adapting its own activities to function within the limits (temporal and spatial) of the other planetary systems. Thus, a mature technosphere would not function independently from the other systems. Instead, it would have adapted to function within the boundaries of a newly enlarged whole that includes its own activities. In short, a class V planet would exhibit planetary scale intelligence (cognitive activity) and, as such, would be autopoietic.
Grinspoon (Reference Grinspoon2016) has argued that Class V worlds could represent the beginning of planet's entering not just a new geological epoch, as in the Anthropocene, but a new eon, which could continue for hundreds of millions of years or more. Just as the other recognized eon boundaries in Earth history can be seen to represent transitions in the functional relationships between the biosphere and the rest of the Earth system, this ‘sapezioc’ eon would involve the application of not just cognition via semantic information flows operating on planetary scales but of wisdom in the sense of ‘the ability to act with judgment born of experience’. Thus, planets in a sapezoic phase would be those in which wise self-management (i.e. the civilization's construction of the technosphere) and wise planetary management are one and the same. The mechanisms for self-management must themselves be collective and global in scale. (Arguably, a benevolent dictator would not constitute a planetary intelligence because the control is local.)
Once again, we should consider the question of feedback timescales. In Fig. 5, we present a schematic diagram of timescales for feedback or ‘interventions’ at work in the different kinds of planets we have been discussing in this paper. These can be described either in terms of the five classes discussed in Frank et al. (Reference Frank, Carroll-Nellenback, Alberti and Kleidon2017) or as is done in this paper via the immature/mature biosphere/technosphere distinctions. For so-called ‘mature biospheres’, the feedbacks represent networks operating via the coupled planetary systems across a range of timescales from decades (DMS ocean temperature regulation) to CH4 climate regulation across millions of years. Note these may or may not be explicitly Gaian in terms of producing a homeostatic regulation. For ‘immature technospheres’, the feedbacks or interventions will be inadvertent. They are the unintentional consequences of the civilization's activity occurring in decades to century timescales. For ‘mature technospheres’, however, the interventions will be intentional. They will be purposely Gaian and designed to maintain the sustainability of both the biosphere and the technosphere as a coupled system. At the short end, ozone replenishment and climate mitigation would occur on decades to century timescales. Terraforming of uninhabited worlds (if possible) is estimated to require up to 1000-year timescales. Planetary defence from asteroids would require the development of systems that would operate over timescales for ‘city buster’ impacts (>1000 years). At the longest timescales and highest technological capacity, intentional changes in stellar evolution (if possible) to prolong habitability would occur over millions of years.

Fig. 5. Timescales for interventions at different proposed levels of planetary intelligence. For so-called ‘mature biospheres’, feedbacks or interventions occur across a range of timescales from decades (DMS ocean temperature regulation) to millions of years for CH4 climate regulation. For ‘immature technospheres’ where the feedbacks or interventions are inadvertent, timescales occur on decades to century timescales. For ‘mature technospheres’ interventions are intentional and designed to maintain the sustainability of both the biosphere and the technosphere as a coupled system. Ozone replenishment and climate mitigation would occur on decades to century timescales while intentional changes in stellar evolution (if possible) would define the longest timescales at tens to hundreds of millions of years.
We make no absolute claims at this point as to the underlying cognitive nature of species that could create a planetary intelligence, but a minimal criterion might be that they should be social. It is possible that only species emerging from particular evolutionary paths, such as eusociality (ants, termites, etc., on Earth), are capable of bringing such global scale cooperative behaviour into existence. Since planetary intelligence acts as a CAS, the existence of a ‘central authority’ is not necessary. A hallmark of a CAS is the mechanism by which local interactions can give rise to global structures and behaviour (Levin, Reference Levin2005). Top-down causation, where the high-level emergent structures alter local behaviour is also seen by some to be essential to CAS operations (Levin, Reference Levin1998). There is considerable literature on how different forms of governance, including democracies, can function as a CAS (Buckley, Reference Buckley1998; Bednar and Page, Reference Bednar and Page2016, Geiselhart, Reference Geiselhart2007). Many of these provide examples of how planetary intelligence could emerge without a single planetary authority serving as the means to agency.
Finally, we consider the role of boundaries and signals in the establishment of planetary intelligence at this level. One might expect a Class V civilization to have the technological capacities to develop a fully functioning asteroid defence. More importantly, though the near-space environment could prove to be a vital part of the integration of the technosphere with the biosphere (Fig. 1(d)). Certainly, one expects sophisticated forms of remote sensing to be part of the tool kit deployed for such integration. But beyond simple planetary scale self-monitoring, one can also imagine that remote interventions may also be part of the toolkit used to develop sustainability. Past these kinds of science fiction-esque visions, the idea of boundaries and signals can take on a less concrete manifestation. The very idea that a technosphere must operate within the safe operating boundaries of the biosphere/geosphere it is embedded in means that new levels of monitoring and response must be developed and deployed on planetary scales.
Inherent to all the discussions above is the possibility that Earth is not the only planet on which a technosphere emerges. Thus, discussions of planetary intelligence may also prove useful to characterizing, and searching for, technosignatures. This domain of Astrobiology has recently seen a resurgence of activity growing alongside traditional SETI studies (Genio and Wright, Reference Genio and Wright2018; Lingam and Loeb, Reference Lingam and Loeb2019; National Academies of Sciences, Engineering, and Medicine et al., 2019). In this regard, the expected age of civilizations whose technosignatures we might detect is of issue. Recently, Kipping et al. (Reference Kipping, Frank and Scharf2020) have shown that, in a galaxy hosting an exponential distribution of civilization ages, long-lived civilizations will be favoured in detection efforts (Fig. 6). Balbi (Reference Balbi2018) and Balbi and Cirkovic (Reference Balbi and Cirkovic2021) have also explored similar trends. The existence of such a ‘contact inequality’ (similar to the well-known income inequality in economics) means that if we find evidence of other civilizations, they may likely be those who have passed through their own versions of an immature technosphere (Frank et al., Reference Frank, Carroll-Nellenback, Alberti and Kleidon2018). Such worlds would then represent a form of Class V planet. The longer lived of these would be representative of sapezoic transitions (at least with regard to planetary stewardship). Given the potential of a sapeozoic era lasting for geologically or cosmologically relevant timescales, it may be that the observable civilizations in the universe are heavily dominated by those which have made such a transition. On the other hand, the demands of sustainability may require that the energy use and other planetary perturbations enacted by such a long-lived civilization are more subtle than those ‘super-civilizations’ imagined in the early days of SETI (Baum et al., Reference Baum, Haqq-Misra and Karmosky2012).
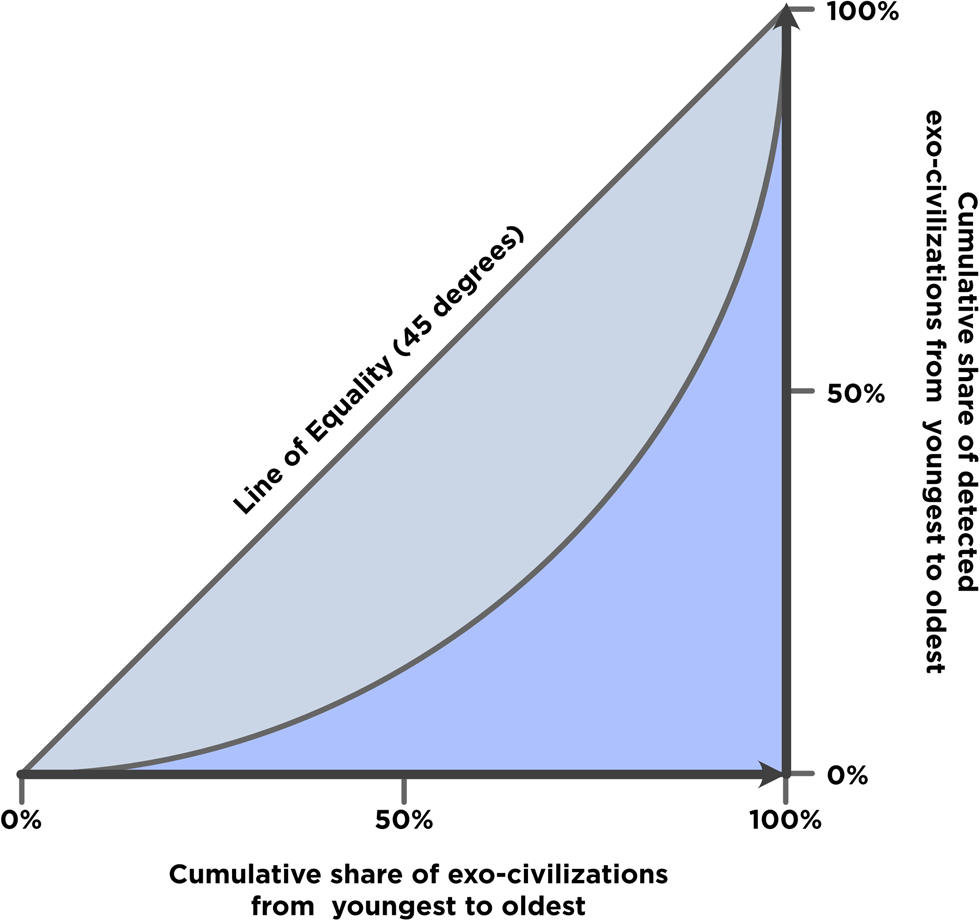
Fig. 6. ‘Contact Inequality’ curve for detection of exo-civilizations. The x-axis represents the age of the civilizations looking to make detections. The y-axis represents the age of the detected civilization (i.e. exo-civilizations). When, on average, civilizations find evidence of others of comparable age, the relation falls along the diagonal line. Using Bayesian methods, Kipping et al. (Reference Kipping, Frank and Scharf2020) demonstrated that the actual detection curve will likely follow a convex curve implying that detected civilizations will be older than the civilizations carrying out the search. Thus, for reasons of long-term sustainability, these older detected civilizations may have already passed through the transition in planetary intelligence to a mature technosphere discussed in the text.
Conclusions and summary
Humanity currently sits at a precipice: our collective actions clearly have global consequences, but we are not yet in control of those consequences. A transition to planetary intelligence, as we described here, would have the hallmark property of intelligence operating at a planetary scale. Such planetary intelligence would be capable of steering the future evolution of Earth, acting in concert with planetary systems and guided by a deep understanding of such systems. If other civilizations that may exist in the universe also undergo such a transition, we would expect to see a marked difference in terms of the signatures of planets with sustainable, global intelligence versus those that have not transitioned to this phase of planetary evolution. Indeed, if planetary intelligence is a requirement for the longevity of planetary-scale civilizations, as we conjecture, we would expect most intelligence we observe in the universe to have gone through this transition.
A critical question is how viewing intelligence as a planetary scale process can help us adapt to and learn to harness the changes we are driving for our own long-term sustainability. Of course, the first question to ask is sustainability for whom? ‘Civilization’ at present is highly unequal in terms of those populations who have the greatest agency in effecting planetary change and those who are the most vulnerable to the consequences of planetary instabilities. Humans, or our descendants in the far future, may be very different than we are at present. Thus, the question of planetary intelligence is as much an ethical and moral one, as it is a scientific one. It implicitly assumes there is collective action that can operate for collective good, at the scale of global dynamical processes. As we have pointed out, what is best for individuals is not always optimal for collectives (e.g. cheating in evolutionary biology). Thus, the transition to planetary intelligence will have to overcome some of the same selfish challenges that evolution has faced repeatedly in the >3.5 billion year history of life on this planet. In fact, we can view the transition to global intelligence as a major transition in evolution, but one that must occur at the planetary scale (Furukawa and Walker, Reference Furukawa and Walker2018).
However, unlike other major transitions in the history of life on Earth, the transition to planetary intelligence is marked by lower-level components (e.g. us) who have some awareness of what is happening. By contrast, it is difficult to conclude that individual cells were aware of, or had a choice in, their joining together to enact multicellularity. Global transitions are already happening affecting almost everything about our daily lives, from what we eat and where, to our social behaviours and economic activity. Often the global features that regulate our behaviour as individuals are mediated by bottom-up action only, that is they are emergent properties of our complex global system. They are not necessarily steered at that global level however. Thus, we are in some sense partway through a major transition, where we have relinquished some of our individuality and behaviours, but we have not yet emerged on the other side where we are in it for the collective good.
To conclude, an exploration of an exploration of planetary intelligence can draw together three domains of study: the evolution and function of Earth's biosphere; the current emergence of the technosphere in the Anthropocene; and the astrobiology of worlds inhabited by technologically capable exo-civilizations. We hope that future work might articulate the properties and applications of Planetary Intelligence in more detail.
Acknowledgements
We gratefully acknowledge many conversations with colleagues including Gourab Ghoshul, Marina Alberti, Axel Kleidon, Jacob Haqq-Misra, Sadie Frank, Kim Stanley Robinson, Harrison Frank., Gavin Schmidt, Ravi Kopparapu, Jason Wright, Caleb Scharf, David Catling, Woody Sullivan, David Kipping, Jonathan Carroll-Nellenback, Evan Thompson and Marcelo Gleiser. This work was supported by NASA grant #80NSSC20K0622.
Conflict of interest
None.