Introduction
The biotic origin of microbialites, such as stromatolites, dendrolites, thrombolites, leiolites and the ‘microbially-induced sedimentary structures’ or MISS (Noffke, Reference Noffke2010), is nowadays widely accepted and recognized in situ by their morphologies and structures (Reitner et al., Reference Reitner, Quéric and Arp2011; Riding, Reference Riding, Reitner and Thiel2011; Noffke, Reference Noffke2015). Although all these structures result from the build-up of clastic particles of rock, they are mediated by living microbial mat, which can be detected in living specimens. However, in organo-sedimentary structure examples, their recognition must be necessarily based on the merging of peculiar macrostructures at micro, meso and macro scales. Possible signature for biotic origin occurs when macrostructures are incompatible with purely sedimentological (i.e. abiotic) processes for the given environment. When Kalkowsky in early 20th century suggested the biotic origin of stromatolites from the Buntsandstein (lower Triassic) of northern Germany (Kalkowsky, Reference Kalkowsky1908), these peculiar carbonate features were regarded by the scientific community as an abiotic product or the consequence of diagenetic processes. In years when geobiological analysis could not rely upon advanced geochemical and microbiological methods, Kalkowsky's intuition was based solely on morphological evidence and on sedimentological criteria. In Mars exploration, we are currently in a state similar to the one at the times of Kalkowsky. Modern laboratory equipment could potentially recognize or rule out ancient or present life on Mars if it could be transported to the Mars surface. However, obvious limitations permit recent landers and rovers to perform some mineralogical and chemical analysis, a few rudimentary biological experiments, and to take a limited number of images, which adds up to the impossibility of direct human examination of the rocks.
Thus, in searching for life on Mars, it is impossible to use the same degree of precision that we can practice on Earth. This is unfortunate, because the most likely form of ancestral life on Mars may have been similar to microbialitic structures on Earth, rather than evolved forms of life, which, however, cannot be excluded from the outset. A fertile way to proceed in search of possible life on Mars would thus be morphometric, i.e. to search for peculiar morphologies and structures from image analysis at the macro, meso and micro scale that may be reminiscent of primitive life forms on the Earth. The results of these morphological and morphometric approaches should be considered relevant, especially when they are obtained in a frame of various and convergent collected clues and when also exist a reasonable assessment on the sedimentary environment in relation to the possible genesis of the structures under examination. Previous works have already shown the occurrence of possible structural matches between terrestrial microbialites and Martian sediments, prevalently at the micro and meso scale, based on visual recognition (Rizzo and Cantasano, Reference Rizzo and Cantasano2009, Reference Rizzo and Cantasano2017; Noffke, Reference Noffke2015), geochemistry (Ruff and Farmer, Reference Ruff and Farmer2016) and mathematical analysis of the images (Bianciardi et al., Reference Bianciardi, Rizzo and Cantasano2014, Reference Bianciardi, Rizzo, Farias and Cantasano2015). Here we shall focus on morphological evidence at the micro and meso scale, and especially at the macro scale (meaning at scales between centimeters and few meters), from images taken from the rovers. We discuss how images may be used to pinpoint some unusual morphologies that could potentially be interpreted as biogenic. In doing so, we apply the same philosophy as Kalkowsky's, presenting a procedure for the selection of images from the rovers (by no means exhaustive) on Mars that may be related to a possible biotic (microbial) origin.
Methods
The methodology adopted in this work consists in analysing blow-ups of wide range images from the rovers on Mars Spirit, Opportunity and Curiosity, identify rocks that may resemble biotic macrostructures, in particular stromatolites, and work out possible abiotic explanations for these structures. If no certain explanation is found at this stage, the essence of this processing may lead to candidates for microbialitic Martian life. Images for the analysis retrieved from the large database of the rovers at NASA website have been examined thoroughly. We sped-up the examination of multiple shots or sequences of images differing little from one another. Images, especially in large-field photographs, have been enlarged to examine blocks of the order 20 cm if in the foreground, and about 2 m in the background. Promising features are so studied in detail and if closer examination reveals forms potentially akin to microbialite structures (including stromatolites, leiolites, thrombolites and dendrolites, as well potential microalgae and/or calcimicrobes), the image has been selected and set aside. In this way, some hundreds of blown-ups have been selected. In a second run, a more critical examination is employed to select further more representative images. At the end of the process, about 20 images more significant have endured in the selection. For each of these images, possible abiotic explanations are discussed. If no alternative abiotic explanation is found, the remaining images were considered as possible candidates for Martian stromatolites.
Morphological criterion for life recognition: the analysed cases
The images in Fig. 1(a)–(c), respectively, from the rovers Curiosity, Opportunity and Spirit, show ring-shaped outcroppings on the barren terrain. While the example of Fig. 1(a) shows a donut-shaped body, with nearly perfect border, the ones of Fig. 1(b) and (c) are flattened to the ground, and show evident concentric onion-like stratification. We suggest that such structures belong to the same class, the difference between the donut-shaped in Fig. 1(a) and the two flat morphologies in Fig. 1(b) and (c) being that the donut-shaped is an earlier form in a process of weather levelling to ground.
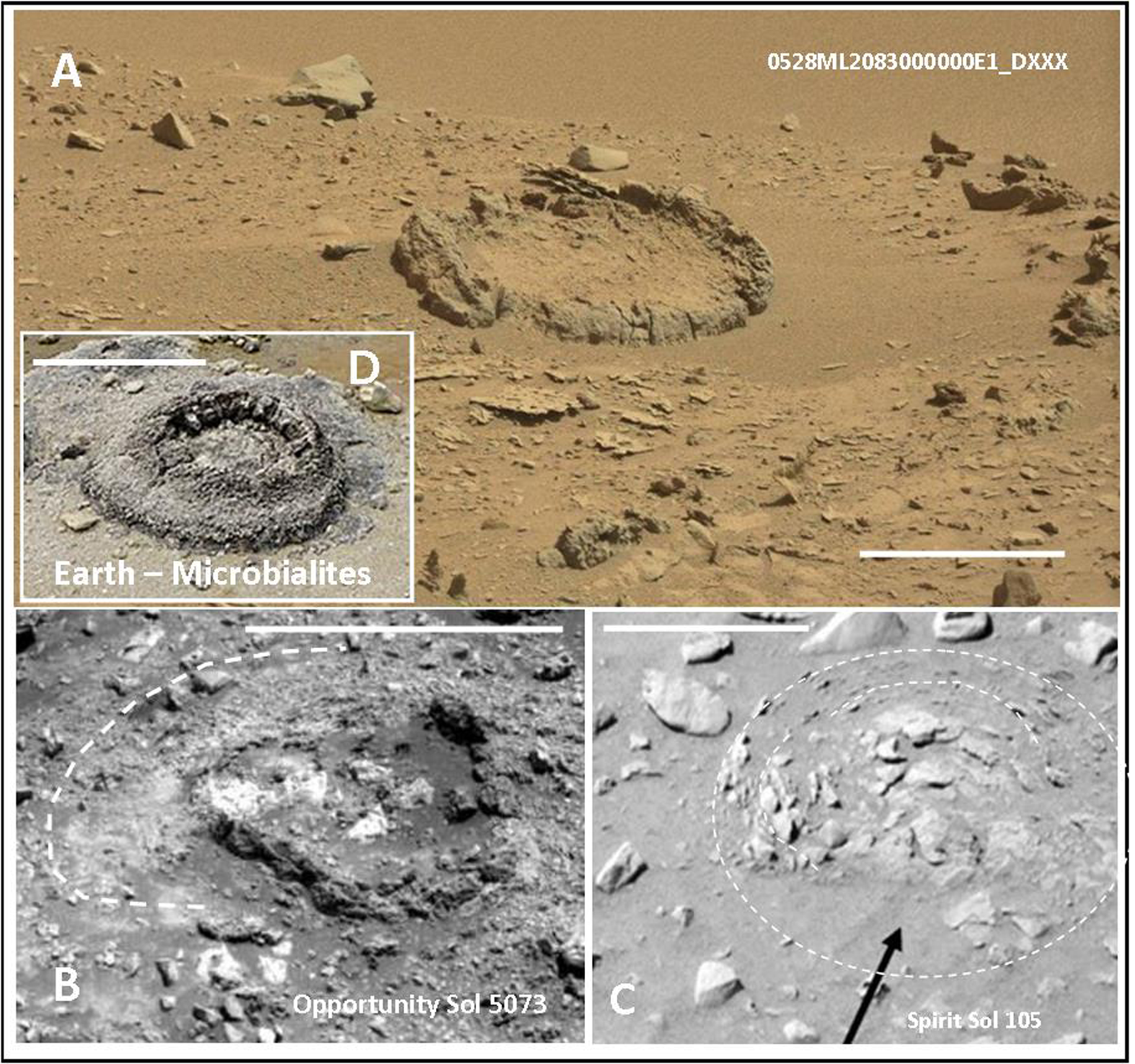
Fig. 1. Rovers observation of a peculiar hemispheric/rounded/flattened outcropping (frames (a–c)), similar to a well-known terrestrial microbialite structure ((d); Lake Thetis, Western Australia). The image in (d) reproduced under the license Wikimedia Creative Commons (Creator Bahnfrend). On Earth, when eroded, such stromatolites may give similar patterns to the one of Fig. (a–c). White bars: about 0.5 m.
Two different abiotic explanations for these morphologies can be put forward. Firstly, they resemble the peculiar, rounded New Zealand spheres known as ‘moeraki boulders’ (Boles et al., Reference Boles, Landis and Dale1985), a type of septarian concretion formed during the first diagenesis of a mudstone. Although it has commonly been assumed that concretions grew incrementally from the inside outwards, the fact that radially oriented cracks taper towards the margins is taken as evidence that the periphery was stiffer than the inside, presumably due to a gradient for cement precipitated, and to an expansion process by an abnormal internal growing. It is unclear whether septarians result from microbial activity as seems to be the case for other spherical concretion like moqui and their likely Martian counterpart ‘blueberries’ (Chan et al., Reference Chan, Johnson, Beard, Bowman and Parry2006). Notably, the inner part of such boulders or its cracks filling is composed of yellow-brown iron minerals of limonite group, in many cases, a product of microbial activity (Potter-McIntyre et al., Reference Potter-McIntyre, Chan and McPherson2014) and its septarian structure is reminiscent of a thrombolitic structure. Often such structures also show a rough stratification, which adds further suspect of a microbialitic association.
Another possible abiotic explanation for hemispheroidal-symmetric structures calls into question the onion-like exfoliations typical of boulders exposed to rapid temperature excursions, a common feature of desert areas. In Antarctica, exfoliation becomes active when the heating rate of the boulder surface exceeds values about 2°C min−1 (Hall and André, Reference Hall and André2001), which at the latitudes of Gusev may occur either at sunrise, or when boulders are shadowed by local hills (Leask and Wilson, Reference Leask and Wilson2003). Thus, a Martian sun much fainter than on Earth is not a reason to exclude exfoliation processes for such features. However, exfoliation does not explain either the reason why the borders are more resistant like in the example of Fig. 1(a), or the complete flattening of outcrops in Fig. 1(b) and (c). Moreover, if exfoliation were the cause, a field of exfoliated boulders should likely be observed rather than few, scanty examples. In terrestrial deserts, exfoliation becomes widespread in the affected area, and this should be even more so on Mars, where the petrographic composition is uniform and the thermodynamic effect of the atmosphere plays a minor role. Yet, the area in Gusev crater is littered with boulders of a size comparable to the ones in Fig. 1 that did not initiate the process of exfoliation.
These macrostructures are in fact similar to a certain class of stromatolite domes that initially form as spherical or hemispherical bodies, and then develop internal cracks and evolve laterally. Because the top of the microbial mat cannot grow higher than the local water level where water absence or stagnation makes the topmost mat die, they develop a dip in the middle. Examples exist at all scales, from the small circle of Lake Thetis (Grey et al., Reference Grey, Moore, Burne, Pierson and Bauld1990) to the giant stromatolites (several meters across) of the Eocene of Colorado (Awramik and Buchheim, Reference Awramik and Buchheim2015). The forms of Fig. 1(a) and (c) also resemble micro-atolls observed in shallow, warm waters (Scoffin et al., Reference Scoffin, Stoddart and Rosen1978). On Earth, micro-atolls are composite structures of diameters ranging from one to a few meters developing from one or the association of taxonomically diverse organisms such as algae, serpulids, corals or bivalves (Meltzner and Woodroffe, Reference Meltzner, Woodroffe, Long and Horton2015). Similar to ring-shaped stromatolites, the form of micro-atolls is a consequence of larger exchange of nutrients and more vigorous growth at the periphery, whereas starvation occurs in the central stagnating waters. Clearly, we are not claiming that similar evolved organisms or communities may have thrived on Mars. Rather, we recognize a universal mode of growth of certain biotic communities, especially of microbial type, occurring when the building of their own edifice is driven by seeking for new space with the constraint of shallow water. The erodible nature of sediments accumulated by microbialites compared to hard rock explains well the erosion to the ground shown in Fig. 1(a)–(c).
The problem opened up by the presence of the spherical or hemispherical structures shown in Fig. 1 is immediately connected with that posed by the discovery in nearby areas of the Gale crater (sols 914–919) of bulgy, dome-like structures (in Fig. 2(a), such domes are highlighted by arrows). Also in this case, it is observed that the post-genetic deformations of the sedimentary sequences tend to form sub-spherical structures (Fig. 2(a) and (b)). Dome swellings appear to be distributed at random, and some of which are affected by cross-shaped cracks indicating a swelling (inset a1). Evidence points at their formation during early diagenesis, in materials with plastic consistency. These localized (decimetric) and random post-genetic deformations are incompatible with purely abiotic, lacustrine sedimentation. A physically quiet lacustrine environment and the dispersing medium of a lake, confirmed by the mm-laminated sequences, cannot alone generate morphological anomalies and localized swellings. They could, however, be explained by the presence of bacterial colonies in lumps and agglomerates with gas production, as happens in the microbial mats on Earth. In fact, such randomly-distributed small domes are comparable to the well-documented field of microbial organo-sedimentary structures (e.g. Licari and Cloud, Reference Licari and Cloud1972 for the Proterozoic of Australia).
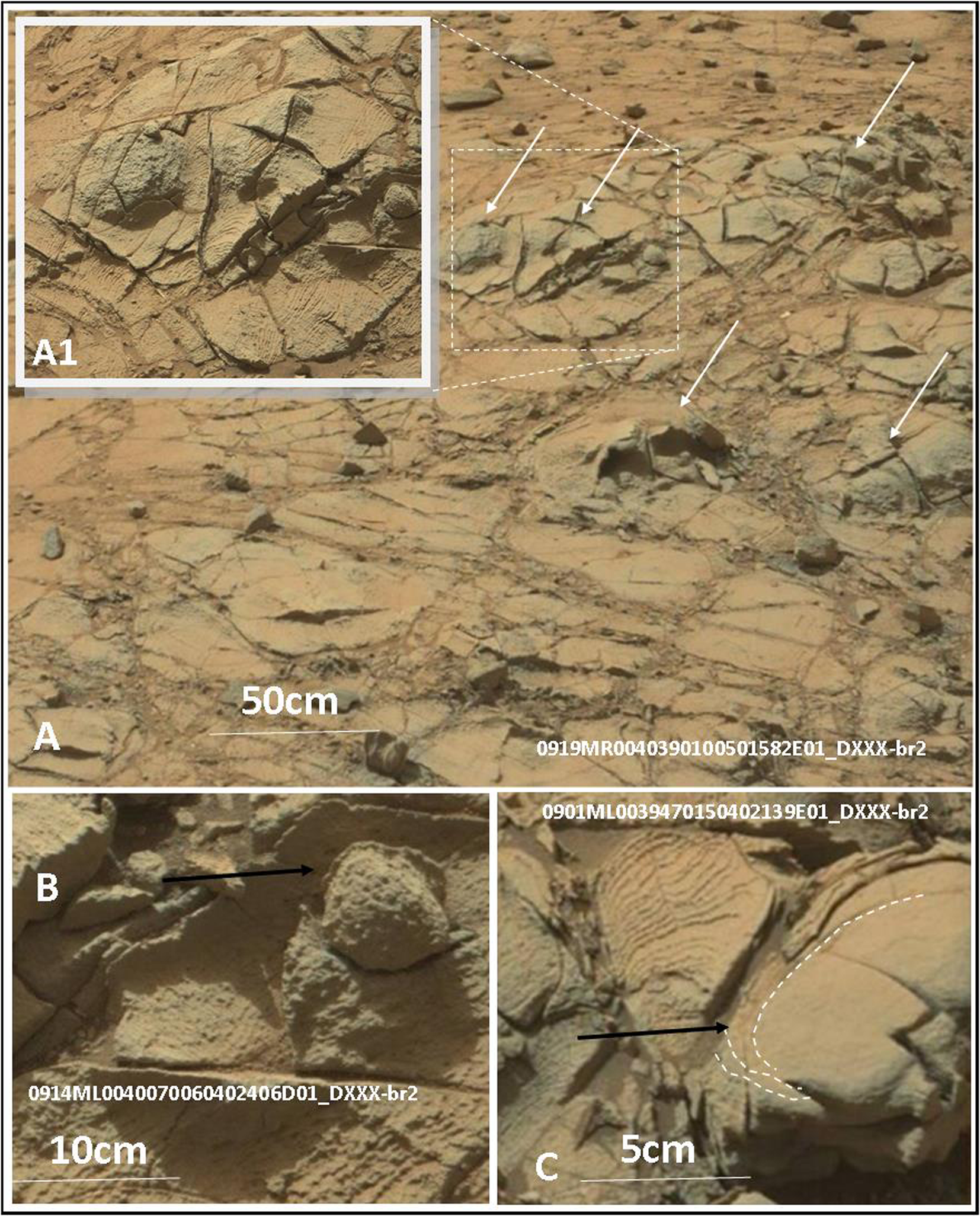
Fig. 2. (a) Small bumps photographed by Curiosity on mudstone. (a1): magnified detail of the frame shown in (a). Note also the peculiar cross-shaped cracks, denoting local post genetic swelling, whose distribution appears to be widespread and involving lamination (frame (b) and (c)). (b) One bump with enigmatic laminations around. (c) More laminations. Significant outlines are highlighted in (c) on laminae setting as dashed lines.
Figure 3(a) shows another example of a ‘bumpy’ structure seen by Curiosity. Bumps protrude (possibly due to higher resistance to erosion) in a fairly regular random-spot fashion from a steep mound. One such bump is shown in more detail in Fig. 3(b). Notice the crustose surface and the irregular edges (Fig. 3(c)). Further enlargement shows the presence of elongated, white spots randomly distributed (Fig. 3(d) and (e)). These spots are tapered, ending in a point at both ends and irregularly curved. At first, they may resemble plagioclase phenocrysts in basaltic or trachyte lavas. However, phenocrysts typically exhibit more tabular and straight crystal surfaces, while such curved spots are more similar to certain microbiological settings like encrusted filaments of blue-green algae (the arrow shows one of these curved spots). In particular, they are reminiscent of Cyanophyta or of certain green algae, such as the mm-sized fragments of Gymnocodiaceae or some cisted forms of Euglenaceae (Riding, Reference Riding and Riding1991; Hindàk and Wolowski Hindàkovà, Reference Hindàk and Wolowski Hindàkovà2000; Flugel, Reference Flugel2010).

Fig. 3. (a) A steep mound seen by rover Curiosity on sol 1256 shows small protuberances on the surface. (b, c) Enlargement of one of the protuberances. (d, e) Detail taken with the Mars Hand Lens Imager (MAHLI) camera; slightly contrasted and amplified b/n images. Fig. (e) shows elongated, white curved spots ending on both sides in a point, interpreted as biogenic forms.
Thrombolites consist mostly of non-laminated small masses created by cyanobacteria clotted together in a crustose surface (Kennard and James, Reference Kennard and James1986; Bridge and Demicco, Reference Bridge and Demicco2008). They may form large mounds and are typically found in saline environments and lagoons. Thrombolites often build bread-shaped small edifices and might be recognized also based on their morphology. Several examples of thrombolitic structures and crusts were also observed at meso scale on Mars and reported in previous works (Rizzo and Cantasano, Reference Rizzo and Cantasano2017, their fig. 9, and fig. 13 frames VI–X). In this work, they found shapes reminiscent of possible thrombolite structures; some of which show large lumps inserted in thin-leaf sediments. Similarly, Fig. 4 shows a comparison between a biogenic build-up of a thrombolite (deposited in a quiet environment) and a blow-up of an image shot by Curiosity, showing a crust over thin-laminated sediment. The possible physical or chemical processes involved in such thrombolitic crusts over or inside thin-laminated sediment are hardly explicable. Irrespective of the details in the development of thrombolites, from a morphological point of view, the perfect similarity of the details is striking: the jagged appearance of the edges, the fissured pattern, the clotted structure and the fragmentation in separated plaques. On Earth, similar structural settings are often the product of cohesive microbial mats covering a surface, consecutively disturbed by alternating cycles of desiccation and watering. Note that this picture was shot a few meters away from the case shown in Fig. 3, thus reinforcing the opinion of a common microbiotic environment.

Fig. 4. Curiosity's observation of a peculiar crust outcropping, taken on Sol 890 (a); frame (a1) shows a blow-up of one of the putative fossilized thrombolite. Image Mastcam, NASA/JPG-Caltech/MSSS. For a better morphological comparison with terrestrial microbialites, the inset frame (b) shows a similar terrestrial thrombolites crust (Australian stromatolite).
Figure 5 shows three significant examples of peculiar lamination observed in Gale crater, already noticed in previous works (Rizzo and Cantasano, Reference Rizzo and Cantasano2017, their figs. 4 and 11). The outcrop appears as a succession of sub-horizontal mm-layers with domal (Fig. 5(b) and (c)) setting and swelling (Fig. 5(a)) upsetting an otherwise parallel deposition. Note that while in Fig. 5(b) and (c), the layers have been eroded along a two-dimensional surface, in Fig. 5(a), a post-depositional basal erosion shows the deposit in its pristine three-dimensional setting. It is remarkable in frames (b) and (c) the presence of a wavy lamination and laminae (L1 and L2) having lateral continuity but forming a domal structure inside, which is characterized by a local increase in the number of the laminae (on frame (c): from two to the edge ‘P’ to five in the central part). Because on Mars there has been no significant compressive tectonic activity, non-horizontal, abiotic folded layers can be the product either of aeolian deposition or of some other local process such as slumping or sedimentation along a local delta. Aeolian deposits on both Earth and Mars appear, however, as parallel packs of layers that abruptly change the deposition angle, which corresponds to an episode of wind turn; here, in addition, sediments appear locally disturbed on a much smaller scale (centimetric) than wind action would require. While a post-genetic process could explain the wavy lamination, the increase in the number of laminae is difficult to explain, and is one of the features of lateral variations in stromatolites. Such evident heteropy could probably explain the domal growth we observe on the nearest outcropping shot by Curiosity.

Fig. 5. Peculiar curved mm-lamination, forming domal structures (arrow lines mark the occurrence of domal structures), observed by Curiosity on sol 1348 (a), sol 1724 (b) and sol 1961 (c). White bar is about 1 cm.
Similarly, structures like ‘roll up’ or strongly wrapped/curved single layers (Noffke, Reference Noffke2015; see also Rizzo and Cantasano, Reference Rizzo and Cantasano2017, their figs. 13, subframes 1a–c, and 14), respectively, on the top and inside undisturbed sedimentary sequence, cannot be explained with the normal deposition of granular sediments. These structures are the consequences of very high adhesion/plasticity of the layers, a property that is typical of microbial mat and is due to organic polymers secerned by bacteria (Extracellular Polymeric Substance, EPS). As well certain sediments, like clays, when alkaline water mix with cation-rich groundwater could increase their plasticity and have the same behaviour like EPS in microbial mats/films and finally on see microbialitic fabrics. But we have to consider that the environment on Mars during Noachian was neutral and more similar to actual Earth.
Stromatolites often grow along columnar structures when the flow energy of water is relatively low, and in some case, lamination may assume conical or cylindrical shape, often arranged in branched bodies. Figure 6 shows the images shot by Curiosity of a deposit at the border of the ancient Gale lake. In such pictures and in the following figures, white dotted lines, arrows and black thick lines correspond to visible features, and were drawn on blown-up images. The pictures of Fig. 6 show a set of laminated bodies next to each other and arranged in a conical shape. The clay laminae bordering the conical shape appear more consistent, probably due to higher carbonate amount. The conical bodies are in relief and show an external hole. The foils and cones they form are irregularly undulating and have no iso-orientated streaks; this is particularly evident in Fig. 6(a). For this reason, we believe that they cannot be assimilated to the morphologies generated by impact fractures and known in the literature as ‘shatter cones’ (Sagy et al., Reference Sagy, Fineberg and Reches2004); doubtful occurrences of real shatter cones in adjacent areas are now under examination. In Fig. 6(b) and (c), one can observe similar arrangements, but the cones appear tighter and longer; the lamination is evident, forming at times aligned bridges between the different conical structures (dark lines). Also in this case, the laminae are more consistent, rolled and in relief, forming holes on the top, well detectable on magnified images. Cones show a tendency to form branched structures; no far and in laterally setting the same lamination to assume a disordered pattern, becoming deeper and exhibiting convolute layers.

Fig. 6. Pictures (a–c) on the left (shot by Curiosity on sols 56–72) show laminae arranged in a conical shape (cones marked by numbers), with holes in the centre. (a) Cones stem from common bridges, made up of laminae. The structure repeats itself, with four cones fully developed in this example and showing large holes inside. Likewise, in (b) and (c), cones appear more stretched, and joined by repeated levels of laminae; they show on the top laminae windings with small holes in the centre. On the right column (d–f), a synthetic representation of the laminae (dashed lines), shaping cones (thin black lines) and holes (whitish round areas). Thick dark lines show bridges between laminae. White bar is about 5 cm.
In the same area, on sol 107, the rover recorded laminated, cylindrical structures, with holes piercing the surface (Fig. 7); the occurrence of laminae in such a tiled cylindrical structure is evident (white dotted lines were inserted by following the border of laminae in good resolution amplified images), as well the occurrence of holes inside; these last well shown on frame 7(a). All the described structures of Figs. 6 and 7, both conical or cylindrical, show a combination of features (laminae arrangement, cylindrical shape and holes), which are too complex to be ascribed to abiotic processes. In terrestrial sediments, cylindrical holes can be generated by the expulsion of gases or liquids; for example, inside the intertidal zones for variation of interstitial water pressure, or in saturated soils subject to seismic shocks; or could be also diagenetic due to underground flows. But all these cases give rise to very different morphologies, exhibiting isolated and/or repeated structures but side by side. The presence of laminae around the holes, their slim structure and the side-by-side setting dislocation has no terrestrial parallels, and cannot be explained by high-energy flowing or by abiogenic diagenetic processes. Overall such structures are really illogical. Such evidence becomes more strong and interesting looking at the nearest outcrop (on sol 78) of Fig. 8. All these structures resemble the terrestrial stromatolite Conophyton, a taxon from sol 70 on to this image, taken on sol 306, the rover Curiosity kept zigzagging around this level, nicknamed ‘Sheepbed’, finding similar outcrops along its path.

Fig. 7. (a) Cylindrical-shaped laminated structures with central holes (see arrows; detail on blow-up (a1)). (b) Another frame showing elongated laminae, shaped as elongated cylinders. White bar is about 5 cm.

Fig. 8. Peculiar conical structures shot by Curiosity at mudstone outcropping on sol 78. (a1) Magnified detail of the frame shown in (a). Significant outlines highlighted in (b) and (c) on laminae setting as dashed lines. Note the holes (frames (a) and (b), down on the left) at the base of the rock. Black arrows indicate the neat basal structure: conical-cylindrical shapes, whose complexity could be reminiscent of biological processes. White bar is about 5 cm.
Figure 8(a1) shows a columnar feature consisting in a funnel-shaped base (black arrow) surmounted first by a set of laminae (about six) having similar shape and comparable diameter, and then by a succession of few narrow cones. Figure 8(b) highlights the main morphological lines of this feature. A certain degree of cylindrical symmetry is noticeable if the whole assembly is imagined leaning on its right side from an initial upright position. The morphology becomes even more notable if we observe it from a more general context of the boulder to which the frame 8(a1) belongs, as shown in Fig. 8(a). The funnel morphology is evidently part of a group of several columnar, parallel structures (Fig. 8(c)). This macrostructure, built by wrapped and stacked laminae, is geometrically similar to those described in the previous Figs. 6 and 7. Such peculiar and complex bodies are reminiscent of microbialitic forms that grew parallel to each other, and in particular the widespread genus Conophyton consisting of superposed cones. We find some similarity of this group with the toppled reef of Conophyton reported in fig. 4a of Kah et al. (Reference Kah, Bartley and Stagner2009) or to some green dasyclad fossil algae (Flugel, Reference Flugel2010).
Discussion
Between 3.7 and 3 billion years ago, as a consequence of a wet and warm global environment (Kargel, 2004), Mars had important lakes and possibly a large ocean (Parker et al., Reference Parker, Grant, Franklin, Cabrol and Grin2010). The possible presence of lacustrine sediments in craters and chasms visible with high-resolution satellite images is now fully acknowledged (Cabrol and Grin, Reference Cabrol and Grin2010). In parallel, the concept of lithopanspermia according to which rocky material may be exchanged between planets and distant areas of the same planet due to meteoritic impact has been recognized as a possible or even likely process for the exchange of life at planetary distances (Gladman, Reference Gladman1997). Thus, it is conceivable that such favourable Martian environment, similar to the current terrestrial milieu, may have been contaminated by some primitive life forms. McKay and Stoker (Reference McKay and Stoker1989) proposed that the presence of stromatolites was a logical hypothesis on early Mars, and the presence of stromatolites hosted in ancient Martian lakes was apparently confirmed by images from orbiters. Russell et al. (Reference Russell, Ingham, Zedef, Maktav, Sunar, Hall and Fallick1999), inspired by a former study by Williams and Zimbelman (Reference Williams and Zimbelman1994), and based on Viking images, proposed a stromatolite origin for a peculiar whitish formation inside Pollack crater in Terra Sabaea. Modern HiRISE and CTX images with a far better resolution show the presence of complex sub-units, not at variance with stromatolite bodies. However, although stromatolites may give rise to extremely long structures well analysable by orbiters, only rovers can examine single building blocks at the meter scale. Recently, rovers confirmed that the small areas visited by them (Meridiani Planum, Gusev Crater and Gale Crater) were water-rich during the Hesperian period (also as ice-covered lakes). Comparison with terrestrial findings shows that this is exactly the kind of sedimentary environment where one would expect ancient life to have thrived on Mars (Grotzinger et al., Reference Grotzinger, Gupta, Malin, Rubin, Schieber, Siebach and Calef2015). Therefore, in this work, we have collected some peculiar hemispheroidal-symmetric, elongated, conical and laminar structures resembling terrestrial stromatolites observed by the three rovers on Mars. The question is: how can we interpret such structures as genuinely biotic assemblies?
Even in terrestrial rocks, it is not always obvious to discriminate between certain microbialitic and abiotic structures based solely on the morphology if a closer examination at the micro scale is lacking. Comparative analysis at the micro, macro and mega scale, supplemented by sedimentological consideration, may lead to more certain results, and in fact, it is used on Earth for the ‘in situ’ recognition of these types of sedimentary structures (Altermann, Reference Altermann2008). At the micro scale, for example, voids or fenestrae embedded on marine sedimentary layers can be explained by gas production by microbial activity inside the different microbialitic microstructures (Bridge and Demicco, Reference Bridge and Demicco2008). Similar voids, plastic deformations and ‘construction morphologies’, resembling stromatolites, dendrolites and thrombolites, have been recognized on micro-images by all the rovers on Mars (Bianciardi et al., Reference Bianciardi, Rizzo and Cantasano2014; fig. 7 in Rizzo and Cantasano, Reference Rizzo and Cantasano2017).
Other structures of ‘constructive’ type that on Earth are formed by continuous, biologically-related building up that assume peculiar shapes at different scales (columnar, spherical, filamentous, lamp- or donut-shaped) have a counterpart on Mars (Bianciardi et al., Reference Bianciardi, Rizzo, Farias and Cantasano2015; Rizzo and Cantasano, Reference Rizzo and Cantasano2017). An example is the spherical concretions of hematite (the well-known ‘blueberries’) often cited as evidence for sea-lacustrine environments on Mars (Moore, Reference Moore2004). Blueberries show complex structures closer to the biogenic world (Rizzo and Cantasano, Reference Rizzo and Cantasano2017); they are much more similar to terrestrial ‘moqui’ concretion spherules, of possible biotic origin (Chan et al., Reference Chan, Johnson, Beard, Bowman and Parry2006; Weber et al., Reference Weber, Spanbauer, Wacey, Kilburn, Loope and Kettler2012). While for many structures there is always the possibility of a double interpretation (abiotic/biotic), this becomes more difficult for the cases shown in Figs. 6–8 of this work, as it is not possible within normal sedimentation of laminated clays to imagine the formation of cones and complex structures such as those shown here.
However, it must be considered that some structures, such as those of Fig. 6 at first glance, are unclear and could-like messy rock; but upon a careful analysis of rocks in the area, one can note both the presence of very disturbed and convoluted outcrops as well of conical forms, having laminae and central voids. Evidence, the latter, that arises from an initial subjective interpretation, but then appears validated by the precise and faithful reporting of laminae and void position, precisely located and reported on amplified images. The occurrence of single conical forms, isolated by erosion and widespread on the surface, was shown in previous work (Rizzo and Cantasano, Reference Rizzo and Cantasano2017). The presence of circular voids surrounded by a more resistant structure (such a shell) was also reported for the image of Fig. 7 in the report of NASA website.
All these evidence, if in the one hand testify possibly microbialitic morphological features, on the other hand the disorder of sediment structures poses the problematic presence of alternative hypotheses; as are those connected to shock events, the abiogenic shutter coins, although not typical.
As is known, stromatolites structures are often disputed. Many researchers hypothesize (or demonstrate) the ‘null hypothesis’ for laminae contortion of stromatolites, by a self-organized sedimentary structures (Brasier, Reference Brasier, Golding and Glikson2011); others, because stromatolites only rarely contain fossil microbes and their biogenicity is tacitly assumed on the basis of morphological comparisons with modern, demonstrably biological, structures, believes that their laminated structures are not a proof of their biogenicity (Grotzinger and Rothman, Reference Grotzinger and Rothman1996); then the double hypothesis (biogenic or abiogenic) for laminated structures like stromatolites is always possible.
However, we have to consider that the discovery of stromatolites was not born from morphological parallels to the living ones, but from sedimentological observations: from a reasoned analysis, based on illogical and unacceptable structures, which were in contrast with the principles of Sedimentology. Such an approach is especially valid for some meso and macro known stromatolitic structures; the same that are normally used for their ‘on field detecting’. Logics can also be applied to Martian sediments, as we performed for the selected cases presented in this work. This does not exclude the search based on chemical rather than morphological signatures, such as the presence of carbonates or silica (Tewari, Reference Tewari, Chela-Flores and Raulin1998; Ruff and Farmer, Reference Ruff and Farmer2016).
Recent studies with CRISM infrared spectrometer and HiRISE (High-resolution imaging science experiment) on board Mars Reconaissance Orbiter have provided evidence for a carbonate-rich unit in Jezero crater (Horgan et al., Reference Horgan, Anderson, Dromart, Amador and Rice2020), and for silica in a fluvial delta within the same crater (Tarnas et al., Reference Tarnas, Mustard, Lin, Goudge, Amador, Bramble, Kremer, Zhang, Itoh and Parente2019). This is particularly interesting, since Jezero is the landing site for the rover of the forthcoming NASA mission Mars 2020.
Thinking about possible life in ancient Mars (Joseph et al., Reference Joseph, Dass, Rizzo, Cantasano and Bianciardi2019), and perhaps also today (Bianciardi et al., Reference Bianciardi, Miller, Straat and Levin2012), we can consider the existence of a possible carbon cycle on Mars that could be linked somehow to the presence of a biota. On Earth, there are three interrelated carbon-bearing reservoirs: the lithosphere, the hydrosphere and the atmosphere. On these, the biosphere play a subtle role in upsetting the balance between the other sources (we could consider, also, a carbon input to the Earth from meteorites and other extraterrestrial material). Likely, on Mars, if we could be able to determine if an equilibrium exists/existed between its carbon reservoirs or, vice versa, whether there is/was an imbalance of those ones, in the latter case we might ascribed it to the influence of a biosphere, in the past or, perhaps, nowadays, also. e.g. the balance of the carbon isotopes on Mars. When living beings use carbon along their metabolic pathways, they preferentially use 12C over 13C (δ13C). Carbon with a high ratio of 12C compared to 13C is therefore an indicator of living processes. In effect, on Earth, δ13C originated from a biota, being depleted from the heavier isotopes, varies from −3.45 to −0.88%, according to the different biochemical pathways (O'Leary, Reference O'Leary1988). So, on Earth, carbon enriched in 12C has been identified in very ancient rocks from Greenland, dated back 3.85 billions of years ago, giving us the earliest evidence for life on Earth (Mojzsis et al., Reference Mojzsis, Arrhenius and McKeegan1996). And what about the planet Mars? We have some data on our hands, regarding Mars and isotope composition in its rocks. Some of the meteorites that have fallen on Earth came from the Red Planet. Results derived from Martian meteorites, although they are not sedimentary rocks, such as the chassignites, the shergottites and the nakhlites indicate strongly variable values of δ13C, ranging from −2.4 to +4.1%, the former value consistent whit the presence of organic material built by living beings (Grady and Wright, Reference Grady and Wright2006). However, the issue is very delicate and alternative hypotheses are possible (Hu et al., Reference Hu, Kass, Ehlmann and Yung2015; Etiope, Reference Etiope2018). If the ExoMars 2020 rover, or preferably when humans will go on Mars, will be able to deeply analyse the isotopic composition of some of the rocks which are highly suggestive for stromatolites and/or fossils, as here discussed, we may have further compelling evidences for the existence of life in the past of Mars.
Conclusions
Purely morphological reasoning has proven fertile in Sedimentology, because it was using the morphological rationale that in 1908, Kalkowsky discovered the biotic role in the build-up of stromatolites. At present, we are in a similar position regarding the recognition of putative biotic morphologies on Mars. Thus, one should not dismiss possible biotic morphologies only because based solely on morphological evidence. This is especially true when morphologies with many peculiar and convergent biotic characteristics are present, which on Earth cannot be attributed to the normal sedimentation of physical–chemical origin. In fact, we have shown that several images at the metric scale might be signatures of past microbic life on Mars. Images show evidence of microbialites in a wide spectrum of geometrical structures already known on Earth: domal, conical, spherical, hemispherical and columnar. Abiotic explanations for these forms have been discussed, but appear contrived and less natural. In particular, the structure of Fig. 8 with its conical base from which superimposed laminae depart is the most difficult to explain with abiotic processes. Moreover, such images should be judged bearing in mind that the presence of microbialites in Martian lakes is not only a logical possibility for Noachian and Early Hesperian on Mars, but has probably been already observed by rovers and orbiters, even though recognition as such is still debated and not prevalent. Stromatolites are, in fact, among the best candidates to search for extraterrestrial life if inspection is limited to macroscopic image analysis (Domagal-Goldman et al., Reference Domagal-Goldman, Wright, Adamala, Arina de la Rubia, Bond, Dartnell and & Singer2016). One should also be receptive to the possibility of forms different from the terrestrial ones. Even on Earth, modern stromatolites form only a small subset of the enormous variety of stromatolite forms in the organo-sedimentary record (Bosak et al., Reference Bosak, Knoll and Petroff2013).
To summarize, this study shows a selection of Martian macrostructures, some of which may be of biological origin, and advocates microbialites and fossil algae as among the best candidates to search for life on the surface of Mars.
Acknowledgements
Fabio Vittorio De Blasio provided assistance in writing the manuscript, suggested the possible interpretations alternative to biogenic ones and discussed the history of water on Mars. Nicola Cantasano provided valuable information on fossil algae.