With an increasing prevalence of 16 % worldwide and 18 % in Brazil, gestational diabetes mellitus (GDM) is a complex metabolic disorder whose pathophysiology is not completely understood(1,2) . Fetal growth abnormalities are commonly reported, including large-for-gestational age and growth-restricted babies. Furthermore, pregnant people who develop GDM have an increased risk of developing type 2 diabetes mellitus and cardio-metabolic disorders postpartum(Reference Reece3–Reference Kramer, Campbell and Retnakaran5). In addition to alterations in glucose homeostasis and insulin resistance, hypertriglyceridaemia and elevated non-esterified fatty acids are observed in the circulation of pregnant people with GDM, particularly in those who are obese(Reference Ryckman, Spracklen and Smith6). These alterations in glucose and lipid metabolism usually resolve postpartum but may contribute to the development of type 2 diabetes.
The placenta appears to play an active role in the pathophysiology of GDM as placental DNA methylation is associated with insulin sensitivity(Reference Hivert, Cardenas and Allard7). Additionally, the expression of placental genes related to fatty acid esterification and lipid droplet accumulation is higher(Reference Radaelli, Leqercq and Vasrastehpour8), and higher amounts of microRNAs targeting insulin resistance pathways and lipid metabolism have been reported(Reference Lei, Ping and Ling9). However, how these molecular changes translate into changes in placental metabolites have not yet been fully addressed. Therefore, investigation of placental metabolic pathways that are altered in GDM is essential to understand the pathophysiology of this disease.
Alterations in sphingolipid metabolism have been implicated in the pathophysiology of many metabolic diseases, including diabetes(Reference Borodzicz, Czarzasta and Kuch10,Reference Bhagirath and Summers11) . In particular, ceramide species with C16:0 and C18:0 participate in liver dysfunction, insulin resistance and glucose intolerance(Reference Matsuzaka, Kuba and Koyasu12,Reference Turpin, Nicholls and Willmes13) whereas those with very long carbon chains, such as C24:0 and C24:1, seem to exert opposite effects(Reference Keppley, Walker and Gademsey14). Sphingolipid metabolism during pregnancy has not been fully addressed; however, a recent study with 800 pregnant people in the UK identified lower plasma concentrations of sphingomyelin (SM) species 32:1, 41:2 and 42:3 early in the second trimester to be related to GDM development later in pregnancy(Reference Furse, White and Meek15). How, and to what extent, alterations in plasma sphingolipids (SP) are associated with alterations in sphingolipid metabolism in the placenta, particularly in pregnancies complicated with GDM, are not known. In this respect, alterations in placental ceramide (Cer) metabolism have been linked to inflammation and mitochondrial dysfunction in GDM pregnancies, and both an overall higher placental ceramide content(Reference Mejia, Hirschi and Tsai16) and a decrease in specific ceramide species have been reported(Reference Abbade, Klemetti and Farrell17). Altogether, these data suggest that sphingolipids participate in the pathophysiology of GDM and highlight the need for further investigation of alterations in placental sphingolipid metabolism.
Acylcarnitines are metabolites linked to insulin resistance, and distinct acylcarnitine species reflect not only mitochondrial fatty acid oxidation but also amino acid metabolism and intermediary metabolism(Reference Schooneman, Vaz and Houten18). There are few reports of alterations in acylcarnitine species in pregnancies complicated with GDM. Medium-chain fatty acylcarnitine (MCFA-carnitine) and short-chain fatty acylcarnitine (SCFA-carnitine) species were shown to be higher in the plasma of pregnant people with GDM(Reference Batchuluun, Al Rijjal and Prentice19) and lower amounts of a particular acylcarnitine, hydroxy-isovaleryl-carnitine, in the first trimester of pregnancy have been shown to be a predictor of GDM(Reference Nevalainen, Sairanen and Appelblom20). In pre-gestational obesity, placental lipid accumulation is related to decreased mitochondrial β-oxidation and lower contents of total free carnitine and acylcarnitine(Reference Calabuig-Navarro, Haghiac and Minium21–Reference Powell, Barner and Madi23); however, the acylcarnitine species in placentas from pregnancies complicated with GDM are yet to be determined.
To address these important aspects, the present study aimed to comprehensively characterise the lipid species in placentas from pregnancies complicated with GDM by mass spectrometry lipidomics, with a particular focus on sphingolipids and acylcarnitines in a semi-targeted approach.
Experimental methods
Materials
All solvents were Chromasolv LC-MS grade. Methanol, chloroform, ultrapure water and analytical standards were purchased from Sigma Aldrich (Merck KGaA).
Study design and participants
This current study was part of a prospective cohort at the Maternidade Escola of the Universidade Federal do Rio de Janeiro (UFRJ). This cohort took place from July 2017 to March 2019 and the recruitment period lasted until December 2018. The term women was used to accurately reference the study’s findings, as only cisgender women participated in the present study. Women who were up to 28 weeks of pregnancy, between 18 and 45 years old, intended to deliver at the Maternidade Escola, were non-smokers, had a singleton pregnancy, were free of infectious and chronic diseases (except obesity) and had a BMI > 18·5 kg·m-2 were included. Sixty-four women who met the eligibility criteria agreed to participate in the study and a total of forty-three women completed the follow-up until delivery. For this study, we prioritised the homogeneity of placental samples and those with detected fetal and newborn complications were not included, resulting in a final sample size of thirteen placentas. A flow diagram of participants’ recruitment for the cohort and final sample size is shown in Supplementary Fig. 1.
GDM diagnosis followed the criteria proposed by the American Diabetes Association(1) and was performed between the 24th and 28th weeks of gestation. Clinical and socio-demographic characteristics and neonatal and obstetric outcomes of subjects were collected from medical records. Dietary intake was obtained during consultations in the second and third trimesters by trained professionals (see Supplementary method S1 for a detailed description of the dietary intake assessment). GDM management included nutritional counselling for all and insulin therapy for the majority of women participating in the study (see the ‘Results’ section).
Ethical approval
This study was conducted according to the guidelines laid down in the Declaration of Helsinki, and all procedures involving human subjects/patients were approved by the Research Ethics Committee (CEP) of the Maternidade Escola-UFRJ and registered at the Research Ethics National Council (CONEP), under nº. 66949217.0.0000.5275 (Certificate of presentation for ethic appreciation- CAAE). The study was registered at The Brazilian Clinical Trials Registry (REBEC; RBR-3xbpwqy) and clinicaltrials.org (NCT 05174728). Written consent was obtained from all subjects.
Biological samples
Placental samples were collected at delivery from thirteen women according to a standardised protocol(Reference Burton, Sebire and Myatt24). Briefly, villous samples (1–2 cm3) were taken at four random sites, representative of the whole tissue, from the maternal surface after the removal of the basal plate and decidua. Villi were washed twice in ice-cold phosphate-buffered saline at 4°C, cut into smaller pieces (50–100 mg), placed into cryovials (all four sites combined) and immediately frozen in liquid nitrogen. All samples were stored at −80°C until analysis.
In this study, placental samples from healthy (nGDM; n 7) and GDM pregnancies (GDM; n 6) were used. Placental samples from women who developed pre-eclampsia or hypertensive disorders, hyper/hypothyroidism or other pregnancy complications (e.g. cholestasis, chorioamnionitis, fetal and newborn abnormalities) and clinical dyslipidemia were not included.
Placental open profile and semi-targeted lipidomics
Internal standards for open profile lipidomics
A mixture of twenty-four deuterated lipids (online Supplementary Table S1) representative of the following lipid categories and classes was used as internal standards (I.S.) for placental lipid profile analysis: (1) glycerophospholipids category: phosphatidic acid (PA), phosphatidylcholine (PC), phosphatidylethanolamine, phosphatidylinositol, phosphatidylserine (PS); (2) sphingolipids category: sphingomyelins, ceramides and (3) glycerolipids category: triacylglycerols (TAG) and fatty acids. Stock solutions were prepared as follows: 10 mg of each standard was dissolved in 1·0 ml of methanol, apart from TAG (45:0) and TAG (48:0), which were dissolved in 1·0 ml of chloroform:methanol (1:1, v/v) and TAG (54:0), that was solubilised in chloroform:methanol (2:1, v/v). Subsequently, all stock I.S. solutions were mixed and diluted (100 ×) with isopropanol:acetonitrile:water (IPA:ACN:H2O, 2:1:1, v/v/v) to prepare an I.S. mixed working solution that was used as a reconstituting solution for the extracted and dried-down samples.
Internal standards used for acylcarnitine analysis
A stock solution containing eight deuterium-labelled acylcarnitines (200 μmol/l each) was used for acylcarnitine analysis (Cambridge Isotope Laboratories, Inc.): d9-carnitine, d3-acetylcarnitine, d3-propyonilcarnitine, d3-butyrylcarnitine, d9-isovalerylcarnitine, d3-octanoylcarnitine, d9-myristoylcarnitine and d3-palmitoylcarnitine. This stock solution was diluted 20 × with a methanol:water solution (4:1 v/v) to prepare a working solution that was added to the samples.
Placental lipid profile by high-resolution ion-mobility lipidomics
Lipids were extracted using a modified Folch method(Reference Folch, Lees and Sloane Stanley25) and one technical replicate was performed for each sample. The non-polar extract in chloroform was dried under nitrogen and suspended with the I.S. working solution in IPA:ACN:H2O (2:1:1, v/v/v) (I.S. mix composition in Supplementary Table S1). Quality control samples consisted of a pooled sample containing 10 μl aliquots of each sample. Quality control samples were analysed in three technical replicates.
All samples were analysed in positive and negative ionisation modes. An Agilent 6560 Ion Mobility Quadrupole Time-of-Flight mass spectrometer coupled with an Agilent 1290 ultra-HPLC system was used to combine the separation power and selectivity of LC, ion mobility and MS techniques. The Dual Agilent Jet Stream Electrospray Ionisation Source was operated separately in positive and negative ion modes. A detailed description of the LC-MS analyses is provided in Supplementary method S2. Data pre-processing, including mass and collisional cross section re-calibration and feature finding, was carried out using the packages ion mobility-MS Reprocessor, ion mobility-MS Browser and Mass Profiler from the MassHunter suite vB.08.00 (Agilent Technologies). The resulting data matrices were processed using a KNIME pipeline comprising both KNIME native nodes and integrated R scripts(Reference Liggi, Hinz and Hall26). Feature annotation was performed based on the AccurateMassSearch node of the OpenMS library.
All intensities were normalised to the wet weight of each placental sample. Annotated lipid species were classified into lipid categories, according to the International Committee for the Classification and Nomenclature of Lipids: fatty acyls, glycerolipids, glycerophospholipids, SP and sterol lipids. Each lipid category was subdivided into classes and subclasses(Reference Fahy, Subramaniam and Murphy27). Data from the quality control samples were used to calculate the CV % of annotated lipid species.
Analysis of acylcarnitines in placenta by Ultra-HPLC/MS/MS
A Thermo Scientific UHPLC + Vanquish series coupled with a TSQ Quantiva mass spectrometer (Thermo Fisher ScientificUSA) was used with an electrospray ion source, operated simultaneously in positive and negative ion modes. The organic phase was dried and reconstituted in 50 µl of the I.S. working solution in a methanol:water solution (4:1 v/v). The electrospray voltages were set to 3500 V (positive ion mode) and 2500 V (negative ion mode). Nitrogen at 48 mTorr and 420°C was used as the drying gas for the solvent evaporation. The organic phases were analysed with an ACE Excel 2 C18 PFP column (100 A°, 150 × 2·1 mm, 5 µm) conditioned at 40°C. The mobile phase consisted of (A) 0·1 % formic acid in water and (B) 0·1 % formic acid in methanol. The mobile phase was pumped at a flow rate of 500 µl/min programmed as follows: initially at 99·5 % of A for 1 min, then subjected to a linear decrease from 99·5 % to 0 % of A in 9 min maintained 100 % of B for 2 min, then brought back to the initial composition after 0·1 min. Xcalibur software (Thermo Fisher Scientific) was used for data acquisition and processing. Metabolite’s annotation was performed using targeted MS/MS analysis.
Prior to the statistical analysis, all intensities were normalised to the wet weight of each placental sample. Acylcarnitines were classified according to their carbon-chain length into short (≤ C6), medium (C8–C12) and long-chain (> C14).
Statistical analyses
Data distribution was analysed using the Shapiro–Wilk test and median and interquartile intervals, and the Mann–Whitney test was used for data with asymmetrical distribution or mean and standard deviation and Student’s t-test for data with normal distribution. Categorical variables were compared using the Fisher’s exact test with absolute (n) and relative (%) frequencies. Partial least-squares discriminant analysis (PLS-DA) was performed using MetaboAnalyst 5.0 software (https://www.metaboanalyst.ca/) after data transformation using log10. The variable importance in the projection (VIP) score was used to identify the lipid features with the greatest discriminatory effect between groups. Univariate analyses were performed using unpaired Student’s t-test with the log-transformed dataset. Multiple regression analyses were used to investigate the combined effect of GDM, pre-gestational BMI and maternal age on placental tissue content of sphingolipids and residual plots were further assessed to determine if they were randomly distributed using GraphPad Prism v9.0 (GraphPad Software).
Sample size was determined by the feasibility of recruitment and eligibility criteria and minimum detectable effect size under student’s t test is 1·63. In this context, we define effect size as the difference between the means divided by the pooled standard deviation (Cohen’s d), number of observations per sample n 7, significance level (Type I error probability = 0·05), power of test (1 minus Type II error probability = 0·8) for a two-sided type of test. Minimum detectable effect size under Mann–Whitney’s non-parametric test is 2·02 for the same conditions as previously, except for the number of observations per sample which has been assigned to n 5 to account for the decreased power of the non-parametric test (15 % penalisation).
Results
General characteristics of the women and the newborns
Table 1 shows the characteristics of the women and newborns participating in the study. Pregnant women who developed GDM were significantly older (P = 0·047), while the median gestational age at birth was similar between the groups – 40 weeks in the nGDM and 38·6 weeks in the GDM group (P = 0·09). Women who developed GDM entered pregnancy with a significantly higher BMI than those in the nGDM group. According to BMI classification, in the nGDM group ∼ 70 % of women were lean (18 < BMI < 25) and ∼ 30 % presented with overweight/obesity (25 < BMI < 40), whereas in the GDM group 100 % of women presented with overweight/obesity. Women in the GDM group showed a tendency (P = 0·05) to gain less weight during pregnancy than those in the nGDM group.
Table 1. Women and newborn characteristics

nGDM, healthy pregnancies (see ‘Methods’ section for eligibility criteria); GDM, gestational diabetes mellitus.
* Mann–Whitney test was used for comparing median values.
† Fisher exact test for comparing frequencies.
‡ Fasting and post-prandial glucose levels were calculated using the average results from at least two tests, measured during the last 2–3 months prior to delivery.
Diabetes management was achieved by diet alone (17 % of women, n 1) and diet + insulin (83 % of women, n 5). Metformin and other glucose-lowering drugs were not used for GDM management. This corresponded to a well-controlled cohort as fasting and post-prandial glucose levels of women with GDM, measured during the last 2–3 months of pregnancy, were within the normal range(28). Indeed, similar birth weight and length were observed between the nGDM and GDM groups and these parameters were also within the appropriate range(29). Lastly, no differences were observed in the mode of delivery between the nGDM and GDM groups, despite the fact that 83 % of women in the GDM group had an elective caesarean section compared with 43 % in the nGDM group (P = 0·27). Taken together, the similarities between the groups concerning gestational age at birth and mode of delivery are an important strength of the present study, since they indicate that the placental samples were subjected to the same conditions, except for differences in maternal age, pre-gestational BMI and the development of GDM.
Dietary records showed similar intakes of energy, carbohydrates, protein and lipids between the groups (online Supplementary Table S2). Therefore, the lower gestational weight gain observed in women with GDM may be explained, in part, as a consequence of nutritional counselling, which possibly improved dietary intake compared with the pre-pregnancy period.
Placental open profile lipidomics reveals similar relative amounts of lipid categories in nGDM and gestational diabetes mellitus pregnancies
Open-profile high-resolution lipidomics of the seven placental samples from the nGDM and six from the GDM group resulted in 1227 features, of which 68·5 % (840 features) were annotated (online Supplementary Table S3). Among the annotated features, 77 % were categorised into five lipid categories. All annotated species were found in both nGDM and GDM groups. The average CV % in the quality control samples of all annotated lipid species was 13·84 (+ 4·60, sd). This analytical variation was considered as acceptable for an open profile non-targeted high-resolution lipidomic method.
The annotated species were separated into the lipid categories glycerophospholipids, sphingolipids, non-sterified fatty acids, glycerolipids and sterols(Reference Liebisch, Vizcaíno and Köfeler30). Acylcarnitine species were also detected in the open profile analysis. Glycerophospholipids were the most abundant category corresponding to 65·6 % and 65·1 % in nGDM and GDM, respectively, followed by sphingolipids (9·9 % nGDM and 9·8 % GDM), non-sterified fatty acids (8·0 % nGDM and 7·9 % GDM), glycerolipids (6·4 % nGDM and 6·3 % GDM), sterols (1·6 % nGDM and 1·2 % GDM) and acylcarnitines (0·2 % nGDM and 0·3 % GDM). All lipid categories showed similar relative abundance between the GDM and control groups (online Supplementary Fig. 1).
We also addressed the effect of labour on the composition of placental lipids by comparing placentas from vaginal and caesarean section deliveries in the control group. The results indicate similar contents of all the aforementioned lipid categories, irrespective of the mode of delivery (online Supplementary Fig. 3).
Gestational diabetes mellitus pregnancies promoted alterations in placental sphingolipids
To assess the overall possible changes in placental lipid profile associated with GDM, we performed the supervised multivariate statistical method PLS-DA using all annotated features from the high-resolution ion-mobility lipidomics. We observed a clear separation between groups, as shown in the PLS-DA scores plot, where the first two principal components accounted for 53·3 % of the differences in placental lipid metabolites between nGDM and GDM (Fig. 1(a)). Cross-validation showed robustness, as indicated by the high R 2 (0·8534) in principal component 2.
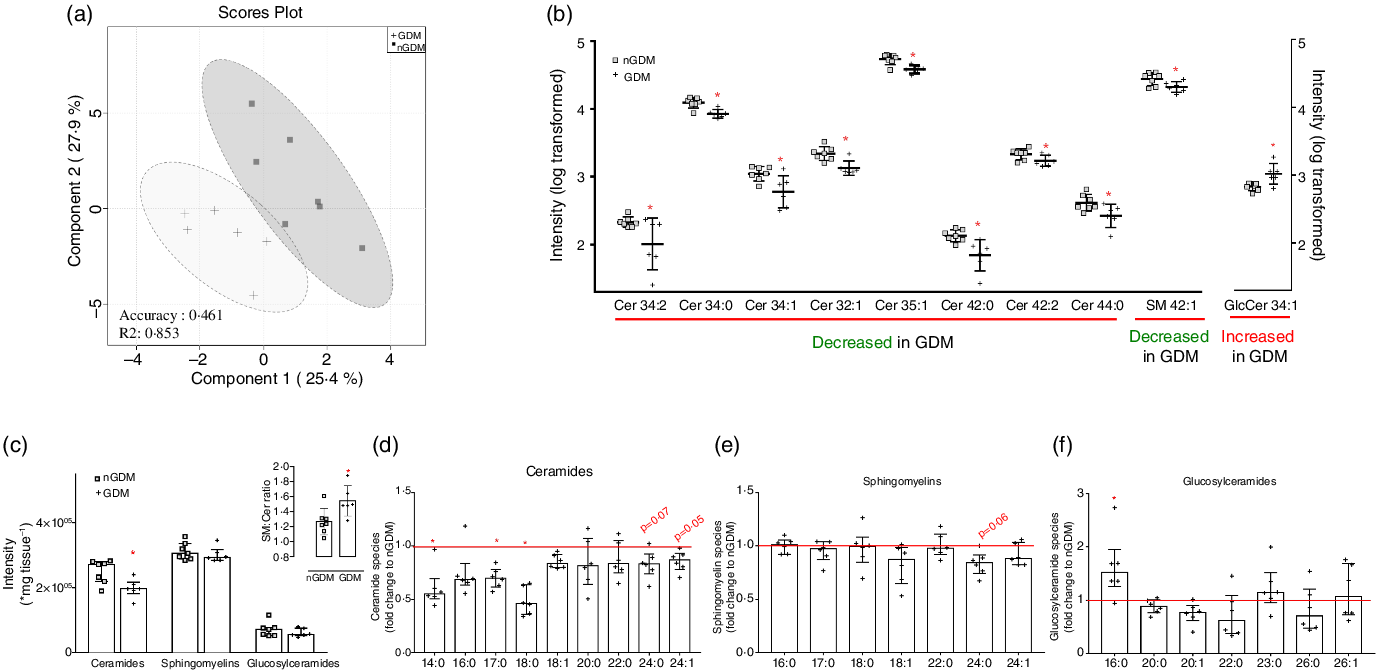
Fig. 1. Discriminant lipid profile by high-resolution ion-mobility lipidomics highlights alterations in sphingolipids in placentas from GDM pregnancies. (a) 2D scores plot of PLS-DA for principal components 1 and 2 shows discriminant placental lipid profile between nGDM (□) and GDM (+) groups. PLS-DA was performed in the Metaboanalyst platform, using data from open-profile high-resolution ion-mobility lipidomics. R 2 measures how good the fit is, where the value 1 would reflect perfect fitting. (b) Sphingolipids with significant discriminant power according to VIP scores (≥0·95) of PLS-DA. Univariate statistics showed a significant lower content of eight ceramide species and one sphingomyelin specie and a significant higher content of one glucosylceramide specie in placentas from GDM pregnancies compared with controls. Sphingolipids species are represented by total number of carbons:number of double bonds. (c) Sum of total ceramides, sphingomyelins and glucosylceramides analysed; inset: Sphingomyelin-to-ceramide ratio. The ratio was calculated considering the sum of annotated sphingomyelins and ceramides according to the open profile high-resolution ion-mobility lipidomics; (d) Total ceramides, (e) Total sphingomyelins and (f) Total glucosylceramides in placentas from GDM pregnancies, expressed as fold change to the nGDM group. nGDM – healthy pregnancies (see ‘Methods’ section for eligibility criteria); GDM – gestational diabetes mellitus. Cer – Ceramides; SM – Sphingomyelins; GlcCer – Glucosylceramides. Data were log-transformed for multivariate and univariate statistics and expressed as the average + standard deviation. *Significant difference between groups by unpaired Student’s t test (P < 0·05). PLS-DA, partial least-squares discriminant analysis; VIP, variable importance in the projection.
VIP scores ≥ 0·95 indicate that glycerophospholipids (61 features) and sphingolipids (38 features) contributed most to the discriminatory lipid profile (online Supplementary Table S4).
Among the thirty-eight sphingolipid species with the highest VIP scores, twenty-five ceramides, three sphingomyelins and four glucosylceramides were further analysed. All ceramide species had negative loading factors, two sphingomyelins presented negative loadings and one positive loading factor and one glucosylceramide presented a positive loading factor comparing the GDM and nGDM groups. All loading factors were in principal component 1 (online Supplementary Table S4). The lower abundance of eight ceramide species and one sphingomyelin, and the higher abundance of one glucosylceramide specie in placentas from pregnancies complicated with GDM compared with nGDM were confirmed by univariate statistics (Fig. 1(b)).
To further investigate the alterations in placental sphingolipid metabolism, we compared the sum of all ceramides, sphingomyelins and glucosylceramides and observed a significantly lower abundance of total placental ceramide content in GDM pregnancies than in nGDM pregnancies (Fig. 1(c)). Additionally, the sphingomyelin-to-ceramide ratio was significantly higher in GDM pregnancies (Fig. 1(c), inset). Next, we evaluated the sum of ceramides, sphingomyelin and glucosylceramides, according to the composition of their acyl chains. The overall abundance of different ceramide species was lower in placentas from GDM pregnancies compared with controls (Fig. 1(d)). In particular, significantly lower contents of ceramides C14:0, C17:0 and C18:0 species were observed. Additionally, the long-chain fatty-acyls species C24:0 (P = 0·07) and C24:1 (P = 0·05) tended to be lower in GDM. Regarding sphingomyelin, a statistical trend was observed only for the C24:0 species (P = 0·06; Fig. 1(e)), which was reduced in GDM. Interestingly, the comparison of glucosylceramides showed a significantly higher amount of C16:0 species in GDM than in controls (Fig. 1(f)). Taken together, these results suggest that placentas from GDM pregnancies present an overall lower abundance of ceramides, which could be related to an increased conversion of ceramides to sphingomyelins.
The relative contents of ceramides, sphingomyelins, and glucosylceramides to the total sphingolipids were also analysed (Fig. 2). The relative abundance of the C17:0 ceramide species was lower in the GDM group, and the C24:1 ceramide species were the most abundant in both groups (median 10·53 % nGDM and 11·09 % GDM) (Fig. 2(a)). As for the relative abundance of sphingomyelin species, the C22:0 was the most abundant in both groups (27·86 % nGDM and 32·35 % GDM). The relative contents of C16:0 and C22:0 sphingomyelin species were significantly higher in the GDM group than in nGDM group (Fig. 2(b)). Glucosylceramides were the least abundant class and were mostly composed of C16:0 and C26:0 species. The C23:0 species were higher in the GDM group (Fig. 2(c)).
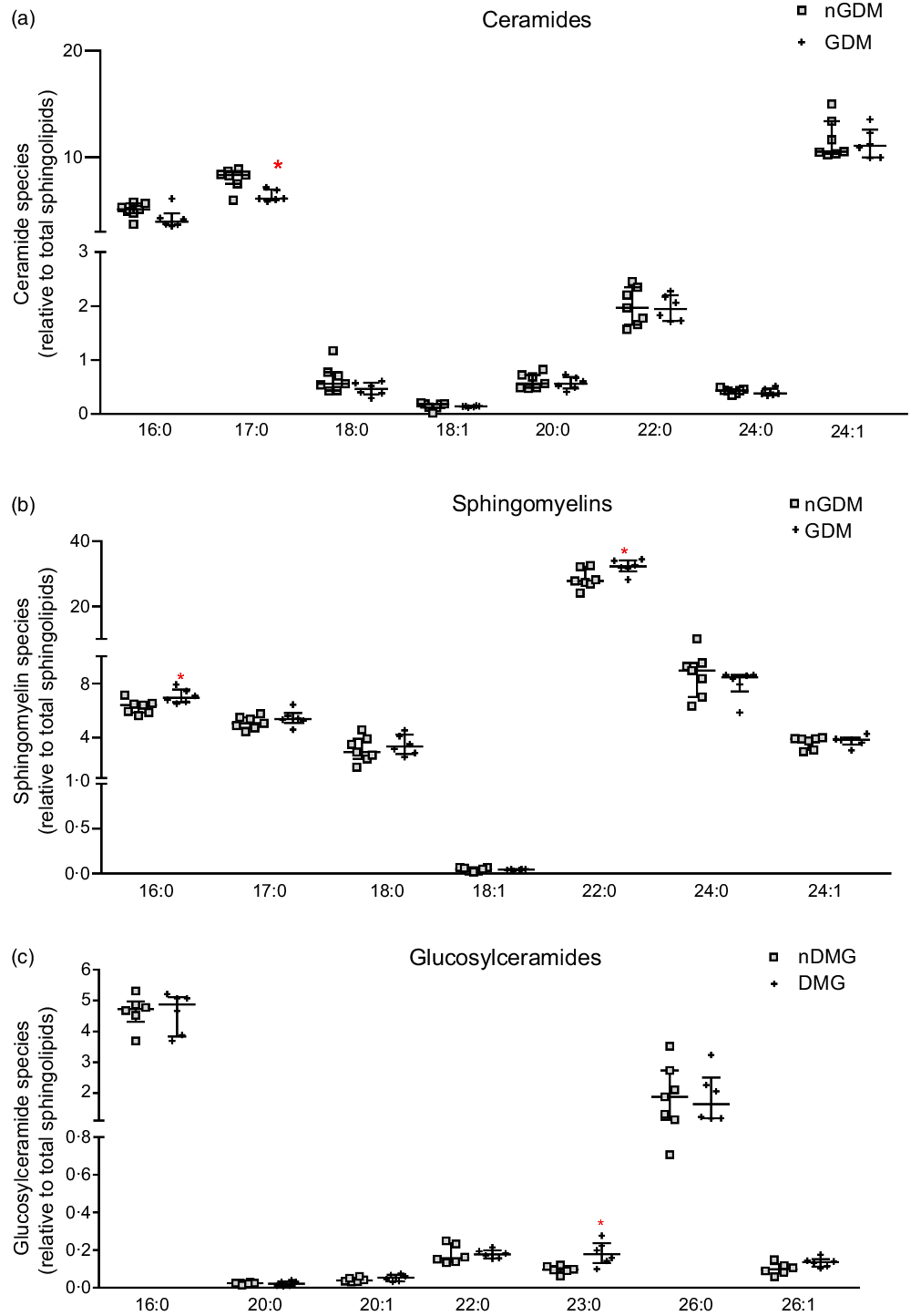
Fig. 2. Abundance of placental ceramides, sphingomyelins and glucosylceramides species relative to total sphingolipids. (a) ceramide species; (b) sphingomyelin species; (c) glucosylceramide species. Relative abundance of sphingolipids species are represented as relative to total annotated sphingolipids according to the open profile high-resolution ion-mobility lipidomics. For this purpose, the sphingoid backbones 18:0, 18:1 and 18:2 were considered. nGDM – placentas from healthy pregnancies (see ‘Methods’ section for eligibility criteria); GDM –gestational diabetes mellitus. Data are represented as average + standard deviation. *Significant difference between groups by unpaired Student’s t test (P < 0·05). GDM, gestational diabetes mellitus.
Because both pregnant people pre-gestational BMI and GDM can regulate placental sphingolipids, and that all women in the GDM group had pre-gestational overweight/obesity, we used multiple linear regression analyses to investigate this association (Table 2). An additional variable included in this analysis was maternal age, as women who developed GDM were older than controls. GDM was associated with a decrease in total ceramide content and of ceramides C14:0, C16:0, C17:0, C24:0 and C24:1 (negative β-coefficients; Table 2), irrespective of maternal pre-gestational BMI or maternal age. Significant models were observed only for ceramides and not for sphingomyelin and glucosylceramides (Table 2). Considering that pre-gestational BMI affects the development of GDM, the fact that GDM was the sole determinant of placental ceramide content in the regression models reinforces that pre-gestational obesity and GDM impose different placental responses when it comes to lipid metabolism, particularly sphingolipids.
Table 2. Multiple linear regression models for the assessment of predictors of placental sphingolipids and ceramide species

GDM, gestational diabetes mellitus.
* Dependent variables. Total sphingolipids, ceramides, sphingomyelins, glucosylceramides and ceramides species (C14:0,C16:0,C24:0 and C24:1) are expressed in relative abundance.
† Independent variables. Pre-gestational BMI is a continuous variable measured in kg/cm2; Maternal age is a continuous variable expressed in years; GDM is a categorical predictor variable with no units. This variable was coded using the absence of GDM as the reference level when performing the dummy coding. β coefficient represents the average difference of specific sphingolipids, ceramides, sphingomyelins, glucosylceramides and ceramides species between women with and without GDM, after taking into account differences in pre-gestational BMI and maternal age.
‡ Model significance. For total ceramides and ceramide species models, there was a significant negative association with GDM, denoted as negative β coefficients independent of maternal age and pre-gestational BMI, denoted as non-significant (ns) P-values.
PLS-DA indicated a substantial contribution of glycerophospholipids to the discriminatory lipid profile between placentas from nGDM and GDM pregnancies (online Supplementary Table S4). However, only minimal differences were observed in the univariate comparisons (online Supplementary Fig. 2). Phospholipids composed by odd-chain fatty acids, namely 37:1 species of PA, PS and PC, were among the metabolites with the highest VIP scores (online Supplementary Table S4). We then analysed the contents of PA 37:1, PS 37:1 and PC 37:1 (online Supplementary Fig. 2(a)), the sum of 37:1 PA + PS + PC species (online Supplementary Fig. 2(b)) and the ratios 37:1 PC:PA, 37:1 PS:PA, and 37:1 PC:PS (online Supplementary Fig. 2(c)) and no differences were found between the GDM and nGDM groups.
Acylcarnitines
Semi-targeted UHPLC/MS/MS was used for the analysis of placental fatty acylcarnitines, and thirty-six species were identified: eleven SCFA-acylcarnitines, eight MCFA-carnitines and seventeen long-chain fatty acylcarnitines (LCFA-carnitines). For brevity, a subset of acylcarnitines is shown and discussed, and a complete list of placental acylcarnitines is presented in Supplementary Table S6 (analytical information) and Table S7 (group comparison). Total acylcarnitine and free carnitine contents did not differ between groups (Fig. 3(a) and (b)). However, important differences in acylcarnitine composition were detected in placentas from GDM pregnancies compared with controls. The sum of SCFA-carnitines was similar, whereas MCFA- and LCFA-carnitines were significantly lower in GDM (Fig. 3(c), (e) and (g)). Propionyl-carnitine (C3) tended to be higher in placentas from GDM pregnancies (P = 0·05; Fig. 3(c) and (d)). And except for C8, C8:1, C10:2, C16:2 and C20:4, MCFA- and LCFA-carnitines were lower in the GDM group (Fig. 3(d), (f) and (h), online Supplementary Table S5).
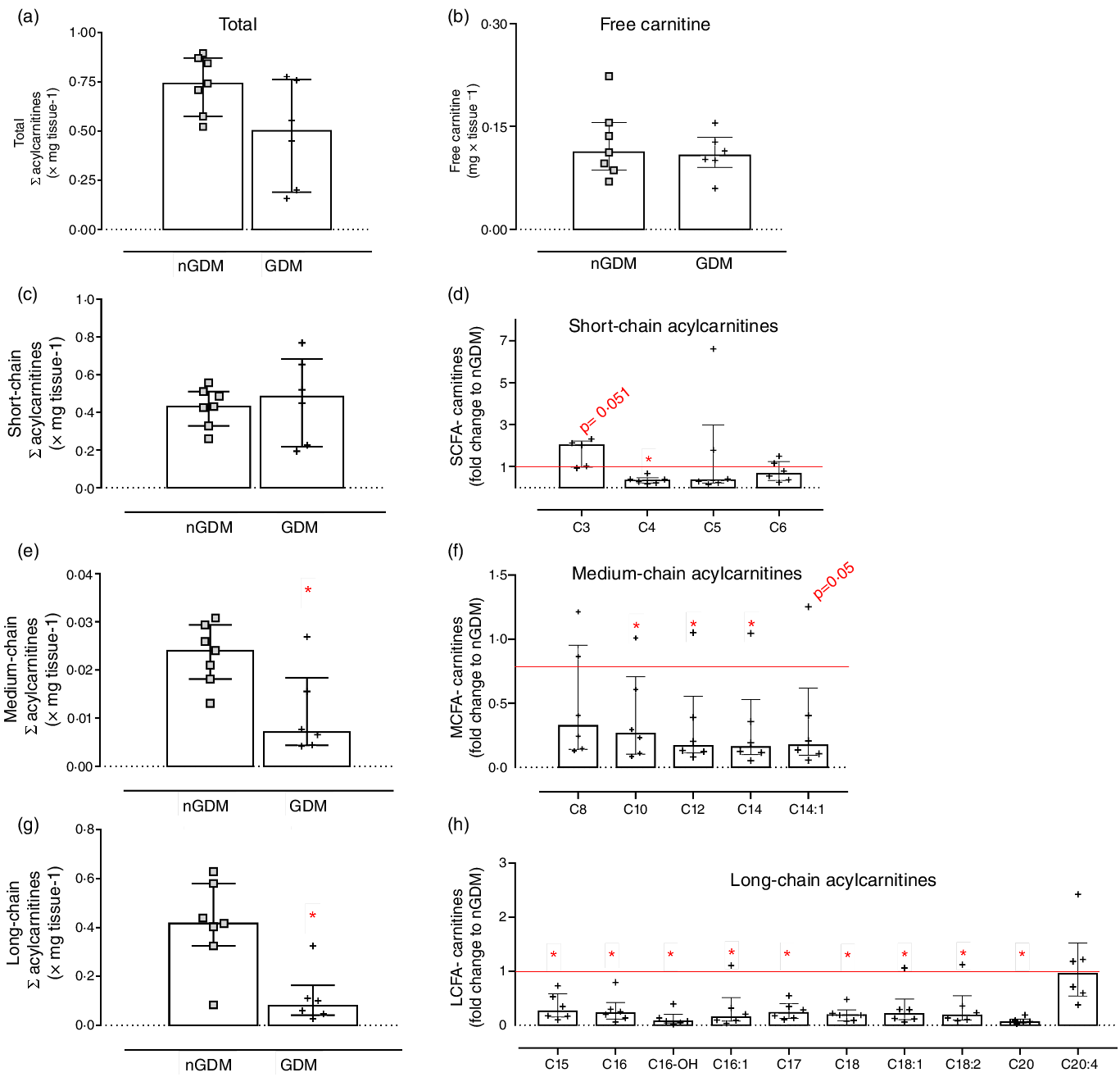
Fig. 3. Placental acylcarnitines identified by UHPLC/MS/MS. (a) Sum of acylcarnitines species; (b) Free carnitine; (c) Sum of short-chain fatty acylcarnitines: chain length up to 6 carbons; (d) Short-chain acylcarnitines in the GDM group relative to nGDM; (e) Medium-chain fatty acylcarnitines: chain length from 8 to 12 carbons; (f) Medium-chain acylcarnitines in the GDM group relative to nGDM; (g) Long-chain acylcarnitines: chain length ≥ 14 carbons; (h) Long-chain acylcarnitines in GDM group relative to nGDM. nGDM – placentas from healthy pregnancies (see ‘Methods’ section for eligibility criteria); GDM –gestational diabetes mellitus. Data represented by median with interquartile range; *Significant differences between groups by Mann–Whitney test (P < 0·05). GDM, gestational diabetes mellitus.
In the GDM group, the ratio of LCFA-carnitines-to-total acylcarnitines was lower (Table 3), whereas the ratio of C3-to-(C15 + C17) was higher (Table 3) than in the controls. These results clearly show that placental acylcarnitine metabolism is altered in GDM pregnancies in particular, LCFA-carnitines were substantially reduced compared with the nGDM group.
Table 3. Acylcarnitine ratios
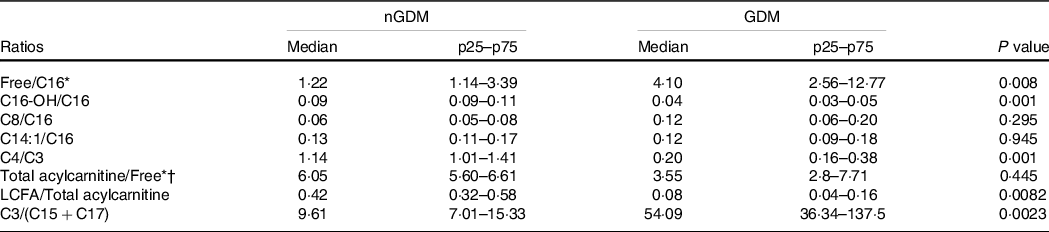
nGDM, healthy pregnancies (see ‘Methods’ section for eligibility criteria); GDM, gestational diabetes mellitus; LCFA-carnitine, long-chain fatty acylcarnitine.
* Free: free carnitine.
† Total acylcarnitine: sum of acylcarnitine species.
Data are expressed as median (p25–p75); Comparative analysis between groups by Mann–Whitney test.
Lastly, we also addressed the effect of labour on the composition of placental acylcarnitines by comparing placentas from vaginal and caesarean section deliveries in the control group. As for the lipid species, there were no differences in placental acylcarnitines attributable to the mode of delivery (online Supplementary Fig. 5).
Discussion
In this study, open profile lipidomics enabled comprehensive characterisation of placental lipid profiles in pregnancies complicated with GDM. The results indicated no major disruption in lipid metabolism, although placentas from women with GDM showed alterations in sphingolipids, mostly lower ceramide content and singular alterations in sphingomyelin and glucosylceramide species. Additionally, semi-targeted lipidomics revealed a strong effect of GDM on the placental acylcarnitine profile, particularly lower contents of MCFA- and LCFA-carnitines species.
Previous reports have shown accumulation of triacylglycerols in placentas from GDM pregnancies(Reference Visiedo, Bugatto and Sánchez31,Reference Hulme, Nicolaou and Murphy32) . This was not confirmed by our data, despite the fact that all women in the GDM group were overweight/obese. This observation was consistent with findings from our previous study, which showed that placentas from pregnant people with pre-gestational obesity did not present signs of lipid accumulation(Reference Belcastro, Ferreira and Saraiva33). Therefore, our data indicate that obesity, whether aggravated or not by GDM, is not associated with increased placental lipid accumulation in well-controlled cohorts of women receiving nutritional counselling and insulin therapy.
Sphingolipids are composed of a sphingoid backbone that in mammalian cells is generally 18:0, 18:1 or 18:2, attached to a fatty-acyl chain(Reference Cheng, Singh and Sharma34). The biological function of sphingolipids depends on the composition of their fatty-acyl chains(Reference Fahy, Subramaniam and Murphy27,Reference Hannun and Obeid35,Reference Del Gaudio, Sasset and Lorenzo36) . Interestingly, downregulation of the pathways related to sphingolipid metabolism in the postpartum period of healthy people was associated with the development of type 2 diabetes mellitus(Reference Khan, Manialawy and Obersterescu37). It remains to be determined whether the alterations in placental sphingolipids observed in our study are associated with an increased risk for future development of type 2 diabetes.
Multivariate analysis showed that sphingolipids significantly contributed to the discriminatory lipid profile of placentas from GDM pregnancies compared with controls. Multiple regression analysis also confirmed a strong negative association between GDM and placental ceramide levels, irrespective of maternal age and pre-gestational BMI. In agreement with our study, placentas from pregnant people with GDM show lower amounts of total ceramides and of C16:0, C18:0 and C24:0 ceramide species, possibly due to a decreased content of ASHA1, the enzyme responsible for ceramide catabolism into sphingosine and fatty acids(Reference Abbade, Klemetti and Farrell17). The lower ceramide content could be regarded as a protective mechanism against their harmful cytotoxic effects, particularly C16:0 and C18:0 species, implicated in insulin resistance(Reference Matsuzaka, Kuba and Koyasu12,Reference Turpin, Nicholls and Willmes13) . Therefore, we hypothesised a protective mechanism for the C16:0 ceramides, which possibly responded positively to insulin and dietary treatment in regulating glucose homeostasis. Indeed, in placentas from pre-eclampsia, higher contents of ceramide species C16:0 and C18:0 have been implicated in oxidative stress and cellular death(Reference Del Gaudio, Sasset and Lorenzo36,Reference Ausman, Abbade and Ermini38) . These results agree with the detrimental effects of C16:0 and C18:0 ceramide species and highlight that GDM and pre-eclampsia might be associated with distinct alterations in placental lipid/sphingolipid metabolism, at least in insulin- and diet-treated GDM patients.
Phospholipids and sphingolipids are the major lipid components of cell membranes(Reference Bieberich39). Lipids in cell membranes can form micro- and nano-domains with protein aggregates, building functional platforms such as lipid rafts enriched with cholesterol and sphingolipis(Reference Rondelli, Pršić and Deboever40). Such rafts, have been observed in the human placenta(Reference Godoy and Riquelme41), are important sites for ligand–receptor interactions and are involved in cell signalling and secretory activities(Reference Simons and Ikonen42). Their functional properties are modulated by the composition of the fatty-acyl chain (both the length and degree of unsaturation) attached to the lipid species(Reference Van Meer, Voelker and Feigenson43,Reference Slotte44) . Particularly, it has been demonstrated that sphingomyelin and ceramide species with C24:0 and C24:1 strongly affect the physical properties of membranes, increasing their binding capacity(Reference Vázquez, Daza and Pavinatto45) and also participate in microdomains and signalling properties(Reference González-Ramírez, García-Arribas and Sot46). The functional significance of the decrease in total sphingomyelin species containing C24:0, and C24:0 and C24:1 ceramide species we observed in GDM placentas needs to be addressed; however, one might speculate that it indicates placental dysfunction and altered signalling properties of rafts enriched with very-long-chain sphingomyelins and ceramides. These changes may be linked to the altered placental responses to insulin and glucose homeostasis. In this context, it has been shown that very-long-chain ceramides have protective effects on the liver in diet-induced steatohepatitis mouse models(Reference Raichur, Wang and Chan47) and have anti-obesogenic effects by modulating PPARα and PGC1α expression, increasing fatty acid oxidation, and improving the acylcarnitine profile(Reference Keppley, Walker and Gademsey14).
Glucosylceramides have also been implicated in insulin resistance and pharmacological inhibition of glucosylceramide synthase enhances insulin sensitivity in adipocytes in culture and rodent models(Reference Aerts, Ottenhoff and Powlson48). Indeed, our results showed that glucosylceramide species 34:1 and C16:0 were significantly higher in placentas from GDM pregnancies.
This is the first study to perform a thorough characterisation of acylcarnitines in human placentas in GDM, and the results are consistent with previous reports on placentas from pregnancies complicated by obesity alone(Reference Calabuig-Navarro, Haghiac and Minium21,Reference Powell, Barner and Madi23) . Alterations in acylcarnitine species have been implicated in insulin resistance, obesity and diabetes in humans and animal models, where a higher amount of MCFA- and LCFA-carnitines are indicative of incomplete and defective mitochondrial fatty acid oxidation(Reference Schooneman, Vaz and Houten18,Reference Mihalik, Goodpaster and Kelley49) . Our results showed that placental MCFA- and LCFA-carnitines were significantly lower in GDM. It should be noted that all women in our GDM group presented with overweight or obesity, reinforcing the notion that placental responses to GDM and obesity share many common pathways(Reference Pantham, Aye and Powell50).
A recent report showed that carnitine palmitoyltransferase-1α, an enzyme that shuttles long-chain fatty-acyls into the mitochondria for β-oxidation, was decreased at both the mRNA and protein levels in cytotrophoblast cells isolated from placentas from GDM pregnancies compared with controls(Reference Valent, Choi and Kolahi51). Therefore, the decreased acylcarnitines we observed might indicate a limitation in β-oxidation flux associated with the carnitine palmitoyltransferase-1α step. Indeed, the higher free carnitine-to-C16 ratio in GDM corroborates a limitation of the carnitine palmitoyltransferase-1α shuttle. The lower LCFA-carnitine-to-total acylcarnitine ratio possibly reflects the lower availability of LCFA-carnitines and indicates that there is no accumulation of acylcarnitine species. Additionally, the significantly lower contents of C16-OH and C14:1 acylcarnitines and the lower C16-OH-to-C16 ratio in GDM suggest a limitation in the first steps of mitochondrial oxidation of very long-chain acyl fatty acids in placentas from GDM pregnancies.
There is evidence that the synthesis of very-long-chain C24 sphingolipids, mediated by ceramidase isoform 2, is regulated by the availability of long-chain fatty acids, mediated by fatty acid elongase 1(Reference Ohno, Suto and Yamanaka52). Therefore, it is possible that the overall decrease in LCFA-carnitines and long-chain sphingolipids share common dysregulated pathways linked to endoplasmic reticulum stress, which was previously shown to be present in GDM placentas by our group(Reference Yung, Alnæs-Katjavivi and Jones53).
The main limitation of the present study is the sample size, which impacts on the statistical power. Thus, results should be interpreted with caution, particularly the multivariate and regression analyses should be considered as an approach to screen for potential biomarkers and predictors of altered placental lipid metabolism. Due to the limited sample size, we were not able to address sex-linked differences in placental lipid metabolism in pregnancies complicated with GDM, as the frequency of female and male foetuses did not allow robust statistical analysis. Sexual dimorphism has been shown to play an important role in placental acylcarnitine metabolism in obesity(Reference Bucher, Montaniel and Myatt22,Reference Powell, Barner and Madi23) and deserves further investigation. Nonetheless, this is a well-controlled cohort as volunteers with GDM presented fasting and post-prandial glucose levels within the normal range and placental samples were subjected to the same conditions, and the only differences were maternal age, pre-gestational BMI and having GDM. Therefore, our data are biochemically sound and highlight the need for mechanistic studies into the role of altered placental sphingolipids and acylcarnitines handling in the molecular pathophysiology of GDM-induced complications of pregnancy. Considering the heterogeneity of the placenta in terms of cell types, an interesting approach would be the investigation of the distribution of lipid species taking into account this aspect. Additionally, the role of cellular organelles in placental lipid handling should be addressed, as it has been shown that decreased ceramide content in mitochondria is associated with altered mitochondrial dynamics in GDM pregnancies(Reference Mejia, Hirschi and Tsai16).
Conclusion
The reduced contents of sphingolipids and acylcarnitines in placentas from women with GDM indicates important alterations in placental lipid metabolism. Considering the role of mitochondria and the endoplasmic reticulum in handling acylcarnitines and sphingolipids, as well as the role of sphingolipids in membrane function, these results are indicative of multi-compartment dysregulation in placentas from GDM. The lipidomic results highlight the need for mechanistic studies on the role of altered fatty acid metabolism and lipid handling in the molecular pathophysiology of pregnancy complications induced by GDM, as they may affect fetal growth and development.
Acknowledgements
The authors acknowledge the contribution of the women who participated in this study, without any direct compensation. We would like to thank the reviewers for their thoughtful suggestions.
This research was funded by Fundação Carlos Chagas Filho de Amparo à Pesquisa do Estado do Rio de Janeiro (FAPERJ; E-026/203.254/2017 for Tatiana El-Bacha), Isaac Newton Trust research grant for Tatiana El-Bacha (18.07-k), Global Challenge Research Fund (ref. 102 642/A19819) for Tatiana El-Bacha, Alexandre G. Torres, and Graham J. Burton, and Coordenação de Aperfeiçoamento de Pessoal de Nível Superior–CAPES-Brasil (Finance code 001), Brazil. Gabriela D. A. Pinto, Carolina S. Ferreira. Deborah de A.B. Guimarães and Desirée. L. Reis were recipients of CAPES scholarship, Vanessa A. Goes and Layla G. Ranquine were recipients of CNPq scholarship (grants GM and GD). Claudio J. Struchiner, Alexandre G. Torres and Tatiana El-Bacha were recipients of research fellowships from CNPq (grant PQ) and FAPERJ (grant CNE).
Conceptualisation: T. E.-B., A. G. T. and G. J. B.; Methodology: A. M., C. L. and J. L. G.; Formal analysis: G. D. A. P., A. M., C. L., C. S. F., V. A. G., D. A. B. G., L. G. R., D. L. R., C. J. S., A. G. T. and T. E.-B.; Investigation: G. D. A. P., A. M., C. L., C. S. F., V. A. G., D. A. B. G., L. G. R., D. L. R., C. J. S. and T. E.-B.; Resources: T. E.-B., A. G. T., C. J. S., G. J. B., J. L. G.; Data curation: A. M., C. L. and J. L. G.; Writing—original draft preparation: G. D. A. P. and T. E.-B.; Writing—review and editing: all authors; Visualisation: all authors; Supervision: T. E.-B., A. G. T. and G. J. B.; Project administration: T. E.-B. and A. G. T.; Funding acquisition: T. E.-B., A. G. T., G. J. B., J. L. G. All authors contributed to data interpretation and performed final editing checks and approved the final manuscript. All authors have read and agreed to the published version of the manuscript.
The authors declare no conflict of interest. The funders, public funding agencies from Brazil, had no role in the design of the study; in the collection, analyses or interpretation of data; in the writing of the manuscript; or in the decision to publish the results.
Supplementary material
For supplementary material/s referred to in this article, please visit https://doi.org/10.1017/S000711452200397X