1. Introduction
Glaciations have had major impacts on the evolution of life on planet Earth (Hoffmann et al. Reference Hoffmann, Condon, Bowring and Crowley2004, Reference Hoffman, Abbot, Ashkenazy, Benn, Brocks, Cohen, Cox, Creveling, Donnadieu, Erwin, Fairchild, Ferreira, Goodman, Halverson, Jansen, Le Hir, Love, Macdonald, Maloof, Partin, Ramstein, Rose, Rose, Sadler, Tziperman, Voigt and Warren2017). Neoproterozoic glaciations most certainly played a major role as a bottleneck for the rise of the Ediacaran biota (Narbonne & Gehling, Reference Narbonne and Gehling2003). Cryogenian (c. 850–635 Ma) strata record the most extreme climate episodes in Earth’s history. The widespread occurrence of low-latitude glacial deposits fundamentally led to the hypothesis of Snowball Earth (Kirschvink, Reference Kirschvink, Schopf and Klein1992; Hoffman et al. Reference Hoffman, Kaufman, Halverson and Schrag1998; Kirschvink et al. Reference Kirschvink, Gaidos, Bertani, Beukes, Gutzmer, Maepa and Steinberger2000). The Marinoan-aged Snowball Earth (Hoffman et al. Reference Hoffman, Kaufman, Halverson and Schrag1998) has been bracketed between c. 635 Ma and c. 639 Ma (Hoffmann et al. Reference Hoffmann, Condon, Bowring and Crowley2004, Reference Hoffman, Abbot, Ashkenazy, Benn, Brocks, Cohen, Cox, Creveling, Donnadieu, Erwin, Fairchild, Ferreira, Goodman, Halverson, Jansen, Le Hir, Love, Macdonald, Maloof, Partin, Ramstein, Rose, Rose, Sadler, Tziperman, Voigt and Warren2017; Prave et al. Reference Prave, Condon, Hoffmann, Tapster and Fallick2016). Cryogenian global glacial events were followed by the Ediacaran Gaskiers glaciation recognized in Newfoundland, with age constraints of 581–579 Ma (Pu et al. Reference Pu, Bowring, Ramezani, Myrow, Raub, Landing, Mills, Hodgin and Macdonald2016). Equivalents with similar isotope age constraints have been described from New England (Thompson et al. Reference Thompson, Ramezani and Crowley2014) and Baltica (Bingen et al. Reference Bingen, Griffin, Torsvik and Saeed2005). The timing of the Gaskiers glaciation might be used to divide the Ediacaran period into Lower and Upper Ediacaran (Xiao et al. Reference Xiao, Narbonne, Zhou, Laflamme, Grazhdankin, Moczydlowska-Vidal and Cui2016). The most significant difference between life before and after this glacial event is the absence of most of the iconic Ediacara biota below and their massive rise and radiation after the Gaskiers glaciation (Narbonne et al. Reference Narbonne, Laflamme, Trusler, Dalrymple and Greentree2014; Xiao & Narbonne, Reference Xiao, Narbonne, Gradstein, Ogg, Schmitz and Ogg2020). There is no evidence for a global Gaskiers ice age at c. 581–579 Ma, as its record is limited to the West Avalonian part of the Appalachians (Youbi et al. Reference Youbi, Ernst, Söderlund, Boumehdi, Ait Lahna, Tassinari, El Moume, Bensalah, Adatte, Bond and Keller2020). However, there is strong evidence that a younger glacial period occurred during late Ediacaran time (560–570 Ma). Geological traces of this event are known from several places in West Africa (e.g. Vernhet et al. Reference Vernhet, Youbi, Chellai, Villeneuve and El Archi2012) and the Cadomian orogenic belt in peri-Gondwanan Europe (Linnemann et al. Reference Linnemann, Pidal, Hofmann, Drost, Quesada, Gerdes, Marko, Gärtner, Zieger, Ulrich, Krause, Vickers-Rich and Horak2018). A compiled dataset points to at least 14 occurrences of Ediacaran glacial deposits worldwide (Youbi et al. Reference Youbi, Ernst, Söderlund, Boumehdi, Ait Lahna, Tassinari, El Moume, Bensalah, Adatte, Bond and Keller2020), which range in age from c. 630 Ma to 560 Ma. In this study, all Ediacaran glacial deposits younger than the Gaskiers and Rocky Harbour formations (c. 581–579 Ma; Pu et al. Reference Pu, Bowring, Ramezani, Myrow, Raub, Landing, Mills, Hodgin and Macdonald2016) are referred to as the Upper Ediacaran Glacial Period, which had a strong impact on the biota 30 to 20 Ma prior to the onset of the Cambrian period, currently dated at 538.8 Ma (Linnemann et al. Reference Linnemann, Ovtcharova, Schaltegger, Gärtner, Hautmann, Geyer, Vickers-Rich, Rich, Plessen, Hofmann, Zieger, Krause, Kriesfeld and Smith2019).
Here, we present new sedimentological and geochronological data from the diamictite-bearing sedimentary succession of the upper Ediacaran Granville Formation in the Armorican Massif (Cadomian orogenic belt of peri-Gondwanan Europe, France; Fig. 1). Such sedimentary units are part of the upper Granville Formation and are characterized by uniquely glaciomarine features. Their maximum depositional ages are provided by laser ablation inductively coupled plasma mass spectrometer (LA-ICP-MS) U–Pb concordia ages of the youngest detrital zircon populations from these clastic sedimentary rocks. In addition, U–Pb LA-ICP-MS detrital zircon ages were used as provenance indicators. Recognition of the glacigenic nature of the Granville Formation is not new, being first suggested by Wegmann (Reference Wegmann1951) and Wegmann et al. (Reference Wegmann, Dangeard and Graindor1950), and later by Graindor (Reference Graindor1957, Reference Graindor1965) and Doré (Reference Doré, Hambrey and Harland1981). This idea was rejected by Eyles (Reference Eyles1990), who countered that the Granville tillite was instead a mass flow deposit. A detailed analysis by Letsch et al. (Reference Letsch, Large, Buechi, Winkler and von Quadt2018 b) pointed out that there was a significantly increased interest in turbidity currents and mass flow processes in the second half of the twentieth century. This change of paradigms led sedimentologists to question the glacigenic nature of many potential tillites, which resulted in a hyper-scepticism of just what diamictites represent. Accordingly, many researchers suggested that diamictites are a product of debris flows (e.g. Schermerhorn, Reference Schermerhorn1974) rather than being related to glaciations. Despite the rejection of the glacigenic nature of the ‘Granville tillite’ by Eyles (Reference Eyles1990), several authors listed it as ‘true tillite’ without any field description and discussion in view of the existing controversy (e.g. Youbi et al. Reference Youbi, Ernst, Söderlund, Boumehdi, Ait Lahna, Tassinari, El Moume, Bensalah, Adatte, Bond and Keller2020). In this paper, we prefer an alternative assessment and present new data that offer in-depth support for the glaciomarine nature of larger parts of the upper Granville Formation, which crops out in the region around the city of Granville (Normandy, Armorican Massif). Maximum depositional ages of detrital zircons in this formation allow us to constrain the succession to late Ediacaran and post-Gaskiers time. Further, we discuss how the sediments of the Granville Tillite Member of the upper Granville Formation record the occurrence of an Upper Ediacaran Glacial Period and its relationship to the strong negative δ13C excursion of the Shuram–Wonoka anomaly. A correlation to related glaciations in Cadomia and adjoining areas helps to improve our understanding about the nature of the Upper Ediacaran Glacial Period.

Fig. 1. Location of glaciomarine deposits in the Cadomian basement of Central and Western Europe: AM – Armorican Massif; BM – Bohemian Massif; FCM – French Massif Central; IM – Iberian Massif; M – Moravo-Silesian unit; S – Sudetes. Variscan zones: CIZ – Central Iberian; CZ – Cantabrian; GTOM – Galicia-Tras os Montes; MZ – Moldanubian; OMZ – Ossa Morena; PL – Pulo do Lobo; SPZ – South Portuguese; SXZ – Saxo-Thuringian; TBU – Teplá-Barrandian unit; WALZ – West Asturian–Leonese. Upper Ediacaran glaciomarine deposits in the Cadomian orogen: a – Granville (Armorican Massif); b – Weesenstein and Clanzschwitz (Bohemian Massif); c – Orellana (Iberian Massif) (modified from Linnemann et al. Reference Linnemann, Pidal, Hofmann, Drost, Quesada, Gerdes, Marko, Gärtner, Zieger, Ulrich, Krause, Vickers-Rich and Horak2018).
2. Geological framework
The Cadomian orogenic belt forms a superterrane along the northern periphery of the West African continent that was active in late Ediacaran time (Fernandez-Suarez et al. Reference Fernandez-Suarez, Gutiérrez-Alonso, Jenner and Tubrett2000; Linnemann et al. Reference Linnemann, Gehmlich, Tichomirowa, Buschmann, Nasdala, Jonas, Lützner, Bombach, Franke, Haak, Oncken and Tanner2000, Reference Linnemann, McNaughton, Romer, Gehmlich, Drost and Tonk2004, Reference Linnemann, Gerdes, Hofmann and Marko2014; Nance et al. Reference Nance, Gutiérrez-Alonso, Keppie, Linnemann, Murphy, Quesada, Strachan and Woodcock2010). The most prominent outcrops of this superterrane occur in modern peri-Gondwanan Europe (Iberia, France, Bohemia, basement of the Pyrenees and Alps; Fig. 1). The so-called ‘Cadomian basement’ is made up of volcano-sedimentary successions and plutonic rocks ranging in age from c. 580 Ma to 537 Ma (Linnemann et al. Reference Linnemann, Gerdes, Hofmann and Marko2014). The overall setting is interpreted to be a geotectonic assemblage of magmatic arcs and back-arc basins (Linnemann, Reference Linnemann, Vickers-Rich and Komarower2007). A recent plate tectonic analogue of this Ediacaran-aged sequence is the western Pacific Ocean (Murphy & Nance, Reference Murphy and Nance1991). The largest part of the sedimentary record of Cadomia was evidently deposited in a back-arc setting (Linnemann, Reference Linnemann, Vickers-Rich and Komarower2007; Linnemann et al. Reference Linnemann, Gerdes, Hofmann and Marko2014). Maximum depositional ages of detrital zircons from several locations point to the opening of the Cadomian back-arc basin in a time window of c. 570–565 Ma, and final post-deformational Cadomian orogenic processes are characterized by a strong plutonic event at c. 540–537 Ma. (e.g. Linnemann et al. Reference Linnemann, Gerdes, Hofmann and Marko2014). Glaciomarine strata in the Cadomia sequence are reported from the Bohemian Massif (Saxo-Thuringian Zone) and Iberia (Central Iberian Zone), which have maximum depositional ages of c. 565 Ma (Linnemann et al. Reference Linnemann, Pidal, Hofmann, Drost, Quesada, Gerdes, Marko, Gärtner, Zieger, Ulrich, Krause, Vickers-Rich and Horak2018). Recent field observations and U–Pb zircon data show a strong linkage of Cadomia with coeval Neoproterozoic crustal units in the Anti-Atlas of Morocco (Vernhet et al. Reference Vernhet, Youbi, Chellai, Villeneuve and El Archi2012; Letsch et al. Reference Letsch, El Houicha, von Quadt and Winkler2018 a; Errami et al. Reference Errami, Linnemann, Hofmann, Gärtner, Zieger, Gärtner, Mende, Kabouri, Gasquet, Ennih, Murphy, Strachan and Quesada2021).
The classic area of outcrop recording the Cadomian orogeny and emplacement of the Cadomian basement, respectively, is the Armorican Massif in northern France (Bertrand, Reference Bertrand1921) (Fig. 1). The first description of an unconformity related to Cadomian orogenic processes was that in Normandy by Bunel (Reference Bunel1835), and much later by Graindor (Reference Graindor1957). An active margin setting for Cadomian basement rocks, including the entire Armorican Massif, was proposed by various French authors (e.g. Chantraine et al. Reference Chantraine, Auvray, Brun, Chauvel, Rabu and Keppie1994). This Armorican Massif can be subdivided into the major North-Armorican, Central-Armorican and South-Armorican domains (Ballèvre et al. Reference Ballèvre, Bosse, Ducassou and Pitra2009). All three domains were formed by the Variscan orogenic processes during Pangaea assembly and show widespread occurrences of an inherited pre-Variscan consolidated Cadomian basement (Ballèvre et al. Reference Ballèvre, Bosse, Ducassou and Pitra2009). In addition, there is also an exotic terrane in the Armorican Massif represented by the northwestern situated Léon Domain, which shows a possible relationship to the Mid-German Crystalline Rise (Faure et al. Reference Faure, Sommers, Melleton, Cocherie and Lautout2010).
Our scientific target, the Granville Formation, was previously highlighted by Dupret (Reference Dupret and Zoubek1984). The formation is part of the Cadomian basement in the North-Armorican domain and has been described as a low-grade, metamorphosed, late-orogenic succession of siliciclastic rocks. The Granville Formation is tectonically isolated and fault-bound from adjoining tectono-stratigraphic units. The presence in the Granville Formation of black chert pebbles constrains the Granville succession to the upper part of the Brioverian Supergroup (Chantraine et al. Reference Chantraine, Auvray, Brun, Chauvel, Rabu and Keppie1994). Wegmann et al. (Reference Wegmann, Dangeard and Graindor1950, Reference Wegmann1951) and Graindor (Reference Graindor1957, Reference Graindor1965) suggested a glacigenic origin for the black chert, pebble-bearing strata of the Granville Formation. Eyles (Reference Eyles1990), to the contrary, suggested the sedimentary deposits of the Granville Formation were debris flows and ‘gravel turbidites’ with no glacial influence.
3. Sedimentology and glaciomarine features
To address this issue of origin of the contested sediments, our field investigations focused on the area around the city of Granville (Normandy, Armorican Massif; Figs 1, 2). A steep, rocky coast along the Atlantic Ocean formed by strong tides and heavy storms offers excellent and fresh outcrops of the Granville Formation (Fig. 2). Except for limited and often temporary outcrops (owing to construction in the city area), the greatest part of the Granville Formation crops out along the coast to the west of the city of Granville. Our recent mapping and documentation of several sections in this area has revealed that this formation can be subdivided on an informal level into a lower and an upper part, here named as the lower and upper Granville formations (Fig. 2). The lower Granville Formation primarily is composed of a dark greenish-grey, immature, massive and thick-bedded quartzite, best exposed along the coast west of the Musée Christian Dior and north of the Rue de la Plage (Fig. 2). The best exposed outcrops of the upper Granville Formation occur at Cap Lihou and Pointe du Lude (Fig. 2). The entire formation has a general NE–SW-directed strike and dips between 30 and 45 degrees towards the NW (Fig. 2). Likely glacigenic deposits occur in the upper Granville Formation, which is composed of a diverse siliciclastic sedimentary sequence including mudstones, quartzites, diamictites, conglomerates and sandy turbidites. For this study our team mapped and sampled in detail the lithologies in the upper Granville Formation at two locales: section 1 at the Rue de Cap Lihou and section 2 at Pointe du Lude (Fig. 2). Both sections can be linked to each other using a marker horizon consisting of a prominent conglomerate (Figs 2, 3). This is the only conglomerate bed in the formation and thus a unique tool for correlation and reconstruction of the depositional setting (Figs 3, 4).

Fig. 2. Geological map from the area of the city of Granville (Armorican Massif) showing the distribution of the Granville Formation, the location of documented sections and the position of a marker horizon. 1 – upper Granville Formation; 2 – lower Granville Formation; 3 – marker horizon (conglomerate); 4 – beach and outcrops exposed at low tide; 5 – strike and dip; 6 – strike-slip fault.
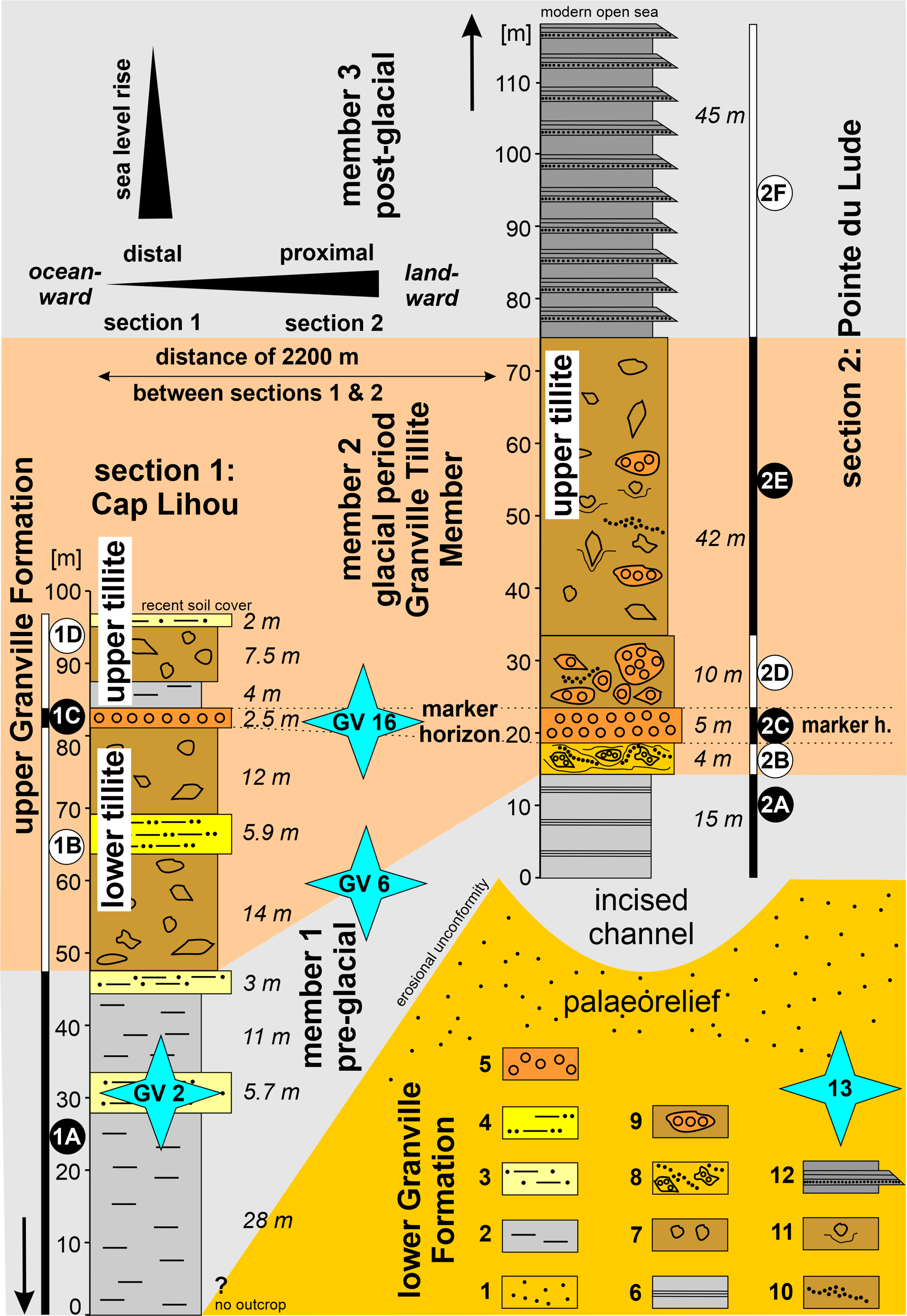
Fig. 3. Lithological columns of two documented sections of the Granville Formation situated at the road-cut in the Rue de Cap Lihou (section 1) and at the cliff at Pointe du Lude (section 2). 1 – massive quartzite of the lower Granville Formation; 2 – mudstone; 3 – intercalation of thin-bedded quartzite and mudstone; 4 – thick-bedded quartzite; 5 – conglomerate (marker horizon); 6 – varve-like very thin-bedded and partial rhythmic arranged turbidites; 7 – diamictite composed of a greywacke-matrix, pebbles up to 6 cm in diameter; 8 – unit of subglacial sediment mingling and ice-coupled glacigenic deformation structures affecting siliciclastic deposits; 9 – fragments of conglomerate (erratic blocks) embedded in a matrix of diamictite; 10 – pockets of sand and fine-grained conglomerate in a matrix of diamictite; 11 – frequent occurrence of dropstones; 12 – sandy turbidites; 13 – sample location.

Fig. 4. Detailed geological map of the lower and upper Granville formations of section 2 at Pointe du Lude south of the Rue de la Douane (city of Granville). 1 – massive quartzites of the lower Granville Formation; 2 – varve-like very thin-bedded and partially rhythmic arranged turbidites; 3 – macro-scale glacigenic deformation structures affecting siliciclastic deposits; 4 – conglomerate (marker horizon); 5 – fragments of conglomerate (erratic blocks) embedded in a matrix of diamictite; 6 – pockets of sand and fine-grained conglomerate in a diamictite matrix; 7 – frequent occurring dropstones; 8 – diamictite composed of a greywacke-matrix and pebbles (in part dropstones) up to 6 cm in diameter; 9 – sandy turbidites of member 3 of the upper Granville Formation.
Owing to limited exposure, the contact between the lower and upper Granville formations is not exposed in section 1. On an informal level, the upper Granville Formation is subdivided into three members with several units based on its diverse lithological composition (Fig. 3). Unit 1A of the section is ∼48 m thick and predominantly composed of mudstones alternating with thin- and thick-bedded quartzites (Fig. 3). This unit is interpreted as a pre-glacial member 1 of the upper Granville Formation. The overlying 32 m thick unit 1B is a diamictite with a 5.9 m thick layer of platy thin-bedded quartzite in the middle. The diamictite matrix is composed of greywacke and mudstone. Matrix-supported pebbles embedded in the diamictite include granite, sandstone, chert, quartzite and vein quartz. The size of the well- to sub-rounded pebbles ranges from several millimetres up to 6 cm. Some of these pebbles are associated with typical impact marks normally associated with diamictites, having a glaciomarine tillite origin. A 2.5 m thick conglomerate horizon (unit 1C) overlies the lowest diamictite of section 1. The conglomerate is component supported, with sub- to well-rounded pebbles averaging 2–5 cm in diameter. The clast composition is similar to that of the underlying diamictite. The overlying units begin with a 4 m thick mudstone containing rare dropstones followed by another 7.5 m thick diamictite with similar features to the lower diamictite in section 1. The uppermost layer of unit 1D is a 2.5 m thick, well-bedded quartzite overtopped by a modern soil (Fig. 3). The conglomerate (unit 1C) lies between the lower and the upper tillites (Fig. 3). Both tillites (units 1B, 1D) and the conglomerate in between make up member 2 of the upper Granville Formation, for which the term Granville Tillite Member is suggested (Fig. 3).
Section 2 crops out at the Pointe du Lude cliffs, offering outstanding exposure at low tide (Figs 3, 4). The northernmost part of the cliff section (Fig. 4) provides the best exposure of members 1–3 of the upper Granville Formation. The outcrops exposed along the western cliff offer excellent exposure of members 1–3 at low tide. Here, at the southernmost part at the coast and directly downhill north from the Musée Christian Dior, the contact between the lower and upper Granville formations (Fig. 4) is exposed. Section 1 differs in its lower part from section 2. It begins at beach level immediately south of the switchback of the Rue de la Douane with mudstones where, in contrast, such a contact with the underlying lower Granville Formation is not exposed in the northern cliff (Fig. 4). The mudstones in this lower unit 2A are ∼15 m thick (Figs 3, 4) and consist of extremely thin-bedded, fine-grained turbidites (Fig. 5a) partly exhibiting a rhythmic arrangement (Fig. 5b), likely reflecting a seasonal and/or process-controlled sedimentation regime. The mudstones appear to be equivalent to member 1 of section 1 (Fig. 3).

Fig. 5. Primary sedimentary structures and features of the Granville Tillite Member (upper Granville Formation) in section 2 (Pointe du Lude). (a) Thin-bedded turbidites of member 1 (upper Granville Formation, unit 2A, length of hammer head = 16 cm). (b) Detailed view on strata shown in (a): very thin-bedded and cyclic arranged turbidites (unit 2A, diameter of coin = 2.3 cm). (c) Conglomerate (marker horizon) containing pebbles of black chert (unit 2C, length of hammer head = 16 cm). (d) Sub-rounded ice-rafted fragment of conglomerate (erratic block) embedded into diamictite (unit 2D, length of hammer = 33 cm). (e) Dropstone of an angular fragment of a granite pebble (unit 2E, diameter of coin = 1.9 cm). (f) Ice-rafted debris and a dropstone of a well-rounded pebble of coarse-grained sandstone (unit 2E, diameter of coin = 2.3 cm). (g) Dropstone of a well-rounded quartzite pebble (unit 2E, diameter of coin = 1.9 cm). (h) Sketch of image shown in (g) that illustrates more clearly the different lithologies, bedding and structures made by the impact of the dropstone.
The mudstones do not crop out along the western cliff (Fig. 4) and pinch out to the SW. Along the western cliff, unit 2B directly overlies the monotonous, massive, thick-bedded quartzite of the lower Granville Formation (Fig. 4). Units 2B to 2E (Fig. 3) up-section reflect a dramatic reduction in thickness compared to that present in outcrops of the northern to western part of the cliff (Fig. 4). The map in Figure 4 showcases the spatial arrangement of the sedimentary units interpreted as deposits formed in a glacial valley incised into the massive quartzite of the lower Granville Formation. Such a valley could have been formed by fluvial erosion of the lower Granville Formation prior to glaciation, so reflecting an erosional unconformity between the lower and upper Granville formations. The suggested base of the incised valley and an assumed onlap contact of member 1 are marked in Figure 4.
The 4 m thick unit 2B (Fig. 3) lies above the mudstones (unit 2A) exposed on the northern cliff and above the massive quartzite of the lower Granville Formation exposure along the western cliff. Unit 2B hosts features indicating a strong glacigenic overprint of proglacial sediments, such as fluvial conglomerates and sandstones (Fig. 6). Deformation appears to have occurred during ice–sediment coupling associated with changing porewater pressures and strain rates. Ring-like arranged turbate structures occur in a mixture of mudstone matrix and fragments of in situ brecciated conglomerates (Fig. 6a, b). Furthermore, there are imbricated deformation structures of sandstone penetrating into the conglomerates during soft sediment deformation processes caused by ice–sediment coupling (Fig. 6c, d). Also present are imbricated deformation structures of load-structures formed during such soft sediment deformation processes (Fig. 6d). Such phenomena can be explained by ice-load and flow-directed stress (Fig. 6d). Turbate structures and imbricated deformation structures induced by ice-load and ice–sediment interactions during glacier growth, overstepping proglacial sediments, have been reported over the last decade with respect to fossil occurrences (Ravier et al. Reference Ravier, Buoncristiani, Guiraud, Menzies, Clerc, Goupy and Portier2014) and are clearly observable in recent glacial settings (Skolasińska et al. Reference Skolasińska, Rachlewicz and Szczuciński2016). We suggest that unit 2B was formed contemporaneously with the deposition of the lower tillite (unit 1B) exposed in section 1 (see stage 1 in Fig. 8) and formed by the glacial advance below the glacier. Unit 2B appears to have been formed owing to the overriding by the proglacial sediments in a channel (Fig. 8, stage 1). Unit 2B offers insight into the interaction of glacial tectonics and temperature effects. Conglomerate blocks in Unit 2B show shearing (‘bookshelf tectonics’). Shear planes are restricted to the blocks and do not cross-cut the surrounding sedimentary matrix. In the muddy matrix often a schlieren-like arrangement of irregular conglomerate shreds is visible (Fig. 7). Such structures point to an in situ fluidization of the conglomerate block resulting in a visible mingling of conglomerate schlieren and mudstone. The situation suggests rip-up and re-embedding of a frozen block of gravel, its ice-coupled deformation in the plastic sediment and final fluidization by marginal melting and mingling with the sedimentary substrate (Fig. 7).

Fig. 6. Glacigenic deformation structures of proglacial sedimentary infill of a glacigenic channel incised into the massive quartzite of the lower Granville Formation. The outcrop is situated at the northern end of the cliffs at Pointe du Lude. Deformation originated during ice–sediment coupling associated with porewater pressures and strain rates. (a) Turbate structures in a mixture of rotated mudstone balls and ring-like arranged fragments of in situ brecciated conglomerate (unit 2B, length of hammer = 33 cm). (b) Small turbate structures in a mixture of a mudstone matrix and well- to sub-rounded pebbles (unit 2B, diameter of coin = 1.9 cm). (c) Imbricated deformation structure of mudstone penetrating into a conglomerate during soft sediment deformation processes caused by ice–sediment coupled shearing (unit 2B, length of hammer = 33 cm). (d) Load structure overprinted by micro-thrusts and imbricated deformation under ice-coupled strain. Please note: micro-thrusts do not cut the entire rock because they were formed in a soft sediment prior to lithification (unit 2B, diameter of coin = 2.3 cm).

Fig. 7. Detailed view on a marginal area of a block of a conglomerate embedded in unit 2B (section 2, Pointe du Lude, upper Granville Formation). The conglomerate block shows shearing (‘bookshelf tectonics’). Shear planes are restricted to the block and do not cross-cut the sedimentary matrix around the block. In the muddy matrix a schlieren-like arrangement of irregular conglomerate shreds is visible. Length of hammer head is 16 cm.

Fig. 8. Model with four stages illustrating the deposition and tectono-sedimentary regime of the lower and upper Granville formations. Positions of sections 1 and 2 are indicated. Deformation of proglacial deposits in a channel incised into the massive quartzite of the lower Granville Formation is indicated by signatures for imbricated deformation (A) and turbate structures (B).
The conglomeratic marker horizon exposed in section 1 (unit 1C) is also present in section 2, represented by a 5 m thick conglomerate (unit 2C; Fig. 3). Sub- to well-rounded pebbles range in size from 1 to 5 cm and are composed predominantly of sedimentary components (quartzites, greywackes, black cherts) and vein quartz (Fig. 5c). Granite pebbles and other types of igneous lithologies are rare. Taking into account the reconstruction of the geological history recorded in both sections prior to the deposition of the conglomeratic marker horizon, we suggest that deposition of this conglomerate occurred after glacial retreat. Thus, we propose that this unique conglomerate, a true marker horizon, was deposited as a massive beach gravel-conglomerate resulting from an interaction of meltwater which produced outwash fans in front of the retreating ice sheet. Likely these fans were reworked then by the surf along the beach. Ongoing sea level rise may have intensified this process (Fig. 8, stage 2). Similar observations of recent settings are known from the coasts along the margins of glaciated areas (Davis & Fitzgerald, Reference Davis and Fitzgerald2004).
A second ice advance and ongoing subsidence due to ice and sediment load on the shelf apparently led to the formation of the upper tillite, which occurs in both of the measured sections (Figs 3, 8, stage 3). In section 2, the lower 10 m of the upper tillite (unit 2D; Figs 3, 4) contains large, sub-rounded to angular, up to metre-sized blocks of the underlying conglomerate (Fig. 5d). Such blocks most likely were formed as rip-up clasts from the conglomeratic marker horizon during glacier advance, which were then subsequently transported as boulders in the tillite matrix (Fig. 8, stage 3). Up-section a 42 m thick second tillite occurs (unit 2E, Fig. 3), which contains abundant ice-rafted dropstones underlain by clear impact structures (Fig. 5e–h). Most of the dropstones have sedimentary lithologies (Fig. 5). One exceptional angular fragment of a granite (Fig. 5e) witnesses fragmentation by stress induced by the glacial ice before the granite became embedded into the tillite as an ice-rafted dropstone. This feature documents the increasing involvement of floating ice and/or icebergs into the sedimentary regime. Unit 2E of the upper tillite also contains rare rip-up clasts of conglomerate fragments and sand pockets, fine-grained conglomerate and pebbles in a diamictite matrix, commonly referred to as ice-rafted debris. Layers made of clasts and sand forming pockets were evidently originally concentrated on the surface of melting icebergs and ice floes and subsequently sank en masse to the sea floor.
The upper tillite in section 2 is overlain by a succession of sandy turbidites, which form unit 2F and the post-glacial member 3 of the upper Granville Formation at Pointe du Lude (Figs 3, 4). One can trace that part of the section for 45 m at low tide before it dips shallowly into the ocean (Fig. 4). The occurrence of post-glacial turbidites above the glacigenic sedimentary rocks suggests a significant deepening of the basin, but this is difficult to explain by sea-level rise caused only by melted ice and subsidence. Thus, we suggest that an additional deepening was forced by rifting in the Cadomian back-arc basin associated with the establishment of submarine turbidite fans (Fig. 8, stage 4).
4. U–Pb ages and provenance
Unfortunately, no ash beds or any significant fossils are known from the Granville Formation. Thus, dating can only be estimated based on included detrital zircons. Based on three samples of siliciclastic rocks from section 1, detrital zircons from heavy mineral concentrates were extracted and analysed employing U–Pb LA-ICP-MS age dating. Heavy mineral separation and isotopic analyses were carried out at the GeoPlasmaLab of the Senckenberg Collections of Natural History Dresden. The analyses of U–Th–Pb isotopes were performed with a RESOlution 193 nm excimer laser (Applied Spectra) connected with a single-collector ICP-MS ELEMENT 2XR (Thermo Fisher Scientific). More details of the analytical procedure, co-ordinates of sample locations and isotope data are available in the online Supplementary Material. Concordia age plots were generated by making use of Isoplot 4.15 (Ludwig, Reference Ludwig2008). Kernel density estimation plots were produced using the detzrcr package for the statistical program R 3.6.1. by Anderson et al. (Reference Andersen, Kristoffersen and Elburg2018).
Sample GV2 (platy quartzite) was taken from the pre-glacial member 1 of the upper Granville Formation. Samples GV6 (greywacke, tillite matrix) and GV16 (conglomerate, marker horizon) were collected from member 2 (Granville Tillite Member, section 1). The positions of the samples in the section are shown in Figure 3.
Maximum depositional ages from all three samples were calculated based on the youngest detrital zircon populations and all were in the same range. Concordia ages varied slightly from 561 ± 3 Ma (n = 11, GV16) via 562 ± 3 Ma (n = 12, GV 2) to 564 ± 8 Ma (n = 3, GV6) (Fig. 9). Thus, sedimentary deposits of the upper Granville Formation are younger than c. 562 Ma. The upper limit of its depositional age is limited by the massive granitoid plutons in the Mancelian region intruded into the upper Brioverian supergroup at c. 550–540 Ma (D’Lemos et al. Reference D’Lemos, Strachan, Topley, D’Lemos, Strachan and Topley1990). The age of the glacial period expressed in the tillites of the upper Granville Formation is bracketed in a time period of c. 562–540 Ma and falls into the latest Ediacaran. Similar age constraints are known from the two other occurrences of glaciomarine tillites in Cadomia situated in Iberia and Bohemia (Linnemann et al. Reference Linnemann, Gerdes, Hofmann and Marko2014, Reference Linnemann, Pidal, Hofmann, Drost, Quesada, Gerdes, Marko, Gärtner, Zieger, Ulrich, Krause, Vickers-Rich and Horak2018).

Fig. 9. Maximum depositional ages (MDA) of samples GV2, GV6 and GV16 (lower Granville Formation) derived from U–Pb LA-ICP-MS ages of the youngest detrital zircon populations of each sample.
U–Pb ages derived from detrital zircons are useful tools for provenance studies, important for our understanding of patterns of crustal growth in the hinterland. All three samples of clastic sediments from the upper Granville Formation show a very similar distribution pattern of zircon populations (Fig. 10) typical for Cadomia and its West African hinterland (Linnemann et al. Reference Linnemann, Gerdes, Hofmann and Marko2014, Reference Linnemann, Pidal, Hofmann, Drost, Quesada, Gerdes, Marko, Gärtner, Zieger, Ulrich, Krause, Vickers-Rich and Horak2018). The U–Pb ages of the detrital zircons from this study can be grouped in six major categories (Fig. 10): (i) late Ediacaran zircon ages related to the Cadomian orogeny sensu stricto (c. 580–560 Ma), (ii) early Ediacaran zircons that have an affinity to pre-Cadomian (Pan-African?) magmatic activity (c. 680–580 Ma), (iii) zircon grains showing an age range of c. 900–680 Ma most probably derived from West African magmatic arcs, dykes and oceanic complexes, (iv) zircons formed during the activity of post-Eburnean arcs and magmatic events (c. 1950–900 Ma), (v) zircons related to the Eburnean Orogenic event of the West African Craton (c. 2200–1950 Ma) and (vi) pre-Eburnean, mostly Archaean zircon grains related to Liberian and Leonian orogenic events and the old nuclei of the West African Craton (Potrel et al. Reference Potrel, Peucat and Fanning1998). Exact percentages of these populations are available from Figure 10. The Palaeoproterozoic and Archaean pattern is typical of a West African Craton provenance (e.g. Linnemann et al. Reference Linnemann, Gehmlich, Tichomirowa, Buschmann, Nasdala, Jonas, Lützner, Bombach, Franke, Haak, Oncken and Tanner2000, Reference Linnemann, Gerdes, Hofmann and Marko2014; Abati et al. Reference Abati, Aghzer, Gerdes and Ennih2010, Reference Abati, Aghzer, Gerdes and Ennih2012). Neoproterozoic zircon populations are diagnostic of provenance from the Cadomian orogen and from the Pan-African basement of the Trans-Saharan Belt or a related area (Linnemann, Reference Linnemann, Vickers-Rich and Komarower2007; Linnemann et al. Reference Linnemann, Ouzegane, Drareni, Hofmann, Becker, Gärtner and Sagawe2011; Drost et al. Reference Drost, Gerdes, Jeffries, Linnemann and Storey2011). The rare Mesoproterozoic zircons might well have been derived from the cratonic neighbours of the West African Craton, which were at c. 570–560 Ma the Amazonian or Sub-Saharan Cratons (e.g. Gärtner et al. Reference Gärtner, Villeneuve, Linnemann, El Archi and Bellon2013).

Fig. 10. Age pattern of U–Pb LA-ICP-MS detrital zircon ages of samples GV2, GV6 and GV16 (lower Granville Formation) based on Kernel density estimation plots (Anderson et al. Reference Andersen, Kristoffersen and Elburg2018).

Fig. 11. Timing of the Upper Ediacaran Glacial Period in the Cadomian orogenic belt and related areas and its calibration to the δ13C curve: in black after Halverson et al. (Reference Halverson, Hoffman, Schrag, Maloof and Rice2005); in orange modification based on new age constraints for the Shuram–Wonoka anomaly by Rooney et al. (Reference Rooney, Cantine, Bergmann, Gómez-Pérez, Al Baloushi, Boag, Busch, Sperling and Strauss2020). Geochronological constraints of the glacial deposits are shown in the upper right of the figure. Bracketed numbers correspond to references. Same numbers mark the palaeogeographical position in the palinspastic map represented in the lower right. Latin numbers: 1 – this study; 2 and 3 – Linnemann et al. (Reference Linnemann, Pidal, Hofmann, Drost, Quesada, Gerdes, Marko, Gärtner, Zieger, Ulrich, Krause, Vickers-Rich and Horak2018); 4 – Errami et al. (Reference Errami, Linnemann, Hofmann, Gärtner, Zieger, Gärtner, Mende, Kabouri, Gasquet, Ennih, Murphy, Strachan and Quesada2021); 5 – Vickers-Rich et al. (Reference Vickers-Rich, Kozdroj, Kattan, Leonov, Ivantsov, Johnson, Linnemann, Hofmann, Al Garni, Al Qubsani, Shamari, Al Barakati, Al Kaff, Ziolkowska-Kozdroj, Rich, Trusler and Rich2013); 5 – Etemad–Saeed et al. (Reference Etemad-Saeed, Hosseini-Barzi, Adabi, Miller, Sadeghi, Houshmandzadeh and Stockli2016). Roman numbers: I – orogenic belts marginal to Gondwana (peri–Gondwanan Neoproterozoic rocks of the Avalonian and Cadomian orogens and related areas in Turkey, the Aegean, the Dobrogea and Iran); II – Neoproterozoic rocks of the Pan–African orogens in the interior of Gondwana; III – Mesoproterozoic orogenic belts; IV – cratonic areas (Palaeoproterozoic to Archaean) (modified from Linnemann et al. Reference Linnemann, Gehmlich, Tichomirowa, Buschmann, Nasdala, Jonas, Lützner, Bombach, Franke, Haak, Oncken and Tanner2000).
5. Discussion and conclusion
Sedimentary features, aspects of basin development, the plate tectonic framework and U–Pb ages, all considered together, allow the reconstruction of Ediacaran glacial processes, palaeogeography and timing of orogenic processes in that part of the Cadomian orogen, which is preserved in the Granville Formation (North-Armorican Domain, Normandy, Armorican Massif). Sedimentary and other features of the deposits further allow tracing a glacial period within sedimentary rocks related to the Cadomian orogen. Indicative of a glaciomarine deposition are ice-rafted dropstones, ice-rafted debris and deformation structures, the direct result of ice-load and ice–sediment interactions associated with changing porewater pressures and strain rates. The latter are represented by turbate structures, in situ brecciation and imbricated deformation in a pre- to syn-diagenetic stage.
Pre-glacial, glaciomarine and post-glacial sedimentation took place in a time span of c. 562–540 Ma. Age constraints completely rule out a correlation of this Upper Ediacaran Glacial Period recorded in the upper Granville Formation with the Gaskiers glaciation, which occurred at c. 581–579 Ma (Bowring et al. Reference Bowring, Landing, Myrow and Ramezani2002; Thompson et al. Reference Thompson, Ramezani and Crowley2014; Pu et al. Reference Pu, Bowring, Ramezani, Myrow, Raub, Landing, Mills, Hodgin and Macdonald2016). Instead, a correlation with a glacial period recorded in the rest of Cadomia, in NW Africa (Morocco) (Vernhet et al. Reference Vernhet, Youbi, Chellai, Villeneuve and El Archi2012), in Arabia (Vickers-Rich et al. Reference Vickers-Rich, Kozdroj, Kattan, Leonov, Ivantsov, Johnson, Linnemann, Hofmann, Al Garni, Al Qubsani, Shamari, Al Barakati, Al Kaff, Ziolkowska-Kozdroj, Rich, Trusler and Rich2013) and in Iran (Etemad-Saeed et al. Reference Etemad-Saeed, Hosseini-Barzi, Adabi, Miller, Sadeghi, Houshmandzadeh and Stockli2016) is much more likely and provides more information concerning the timing of this late Ediacaran glacial period. Upper Ediacaran tillites within the Cadomian orogenic belt have been described from the Saxo-Thuringian Zone of the Bohemian Massif and from the Central Iberian Zone of the Iberian Massif (Linnemann et al. Reference Linnemann, Pidal, Hofmann, Drost, Quesada, Gerdes, Marko, Gärtner, Zieger, Ulrich, Krause, Vickers-Rich and Horak2018).
Owing to the lack of datable ash beds, only the oldest age is well constrained for glacigenic sedimentary deposits in Cadomia, provided by maximum depositional ages of c. 562 Ma (this study and Linnemann et al. Reference Linnemann, Pidal, Hofmann, Drost, Quesada, Gerdes, Marko, Gärtner, Zieger, Ulrich, Krause, Vickers-Rich and Horak2018). Younger time markers can be determined only based on the intrusions of c. 540 Ma aged late Cadomian plutons (Linnemann et al. Reference Linnemann, Gerdes, Hofmann and Marko2014, Reference Linnemann, Pidal, Hofmann, Drost, Quesada, Gerdes, Marko, Gärtner, Zieger, Ulrich, Krause, Vickers-Rich and Horak2018). The youngest limit of the Upper Ediacaran Glacial Period should be placed much earlier, and a likely correlation with the late Ediacaran glaciations in West Africa and in Arabia constrains the youngest age limit (Fig. 11). The Bou Azzer glaciation (West Africa; Verhnet et al. Reference Vernhet, Youbi, Chellai, Villeneuve and El Archi2012) is recorded associated with rhyolite flows of the Ouarzazate Group, which is constrained by U–Pb zircon ages to a duration of from 569 ± 4 Ma to 567 ± 4 Ma (Karaoui et al. Reference Karaoui, Breitkreuz, Mahmoudi, Youbi, Hofmann, Gärtner and Linnemann2015; Errami et al. Reference Errami, Linnemann, Hofmann, Gärtner, Zieger, Gärtner, Mende, Kabouri, Gasquet, Ennih, Murphy, Strachan and Quesada2021). The absolute age of the Dhaiqa Formation (Arabia) is reflected by a U–Pb zircon tuff age of 560 ± 4 Ma (Vickers-Rich et al. Reference Vickers-Rich, Kozdroj, Kattan, Leonov, Ivantsov, Johnson, Linnemann, Hofmann, Al Garni, Al Qubsani, Shamari, Al Barakati, Al Kaff, Ziolkowska-Kozdroj, Rich, Trusler and Rich2013). A glacial deposit intercalated in the Kahar Formation (Central Elborz Mountains, Iran) yields a maximum depositional age of 560 ± 5 Ma derived from U–Pb detrital zircons (Etemad-Saeed et al. Reference Etemad-Saeed, Hosseini-Barzi, Adabi, Miller, Sadeghi, Houshmandzadeh and Stockli2016).
We relate the glacial deposits of the Armorican Massif described in this paper with the other occurrences of Cadomia in the Bohemian and Iberian massifs and with the deposits of glaciomarine diamictites in the Anti-Atlas (Bou Azzer) as well as those in Saudi Arabia (Dhaiqa Formation) and the Kahar Formation (Iran). We propose the term ‘Upper Ediacaran Glacial Period’ for such post-Gaskiers c. 570 Ma to 560 Ma aged glaciomarine deposits (Fig. 11). It should be noted that there are some other rare occurrences of late Ediacaran-aged glacial deposits on Earth. Most are not very well constrained because of their age and/or that their glacigenic nature is currently under discussion (see compilations of Arnaud et al. Reference Arnaud, Halverson and Shields-Zhou2011; Youbi et al. Reference Youbi, Ernst, Söderlund, Boumehdi, Ait Lahna, Tassinari, El Moume, Bensalah, Adatte, Bond and Keller2020). The two most likely ones are located on the North China craton (Le Heron et al. Reference Le Heron, Vandyk, Wu and Li2018) and in Tasmania (Calver et al. Reference Calver, Black, Everard and Seymour2004). Another glacial deposit (Moelv diamictite) with a suggested Ediacaran age also crops out in Baltica (Bingen et al. Reference Bingen, Griffin, Torsvik and Saeed2005), but at present available age data are not sufficient to constrain an age younger than the Gaskiers glaciation. Furthermore, a new U–Pb baddeleyite age for the Ottfjället Dyke Swarm points to a minimum age of 596.3 ± 1.5 Ma for these glacigenic deposits (Kumpulainen et al. Reference Kumpulainen, Hamilton, Söderlund and Nystuen2021).
LA-ICP-MS U–Pb zircon dating is one of the best and most rapid dating methods for detrital zircons. In view of the relatively large errors, the apparent duration of c. 568–561 Ma for the proposed Upper Ediacaran Glacial Period is quite lengthy, noted in Figure 11. Ages obtained using high-precision U–Pb tuff zircon dating for the Marinoan and Gaskiers glacial events constrain such events to a very narrow time corridor (Bowring et al. Reference Bowring, Landing, Myrow and Ramezani2002; Hoffmann et al. Reference Hoffmann, Condon, Bowring and Crowley2004; Thompson et al. Reference Thompson, Ramezani and Crowley2014; Pu et al. Reference Pu, Bowring, Ramezani, Myrow, Raub, Landing, Mills, Hodgin and Macdonald2016; Prave et al. Reference Prave, Condon, Hoffmann, Tapster and Fallick2016).
Relating the Upper Ediacaran Glacial Period (c. 568–561 Ma) to the δ13C curve shows little overlap with the negative excursion of the Shuram–Wonoka anomaly (Fig. 11; Halverson et al. Reference Halverson, Hoffman, Schrag, Maloof and Rice2005; Rooney et al. Reference Rooney, Cantine, Bergmann, Gómez-Pérez, Al Baloushi, Boag, Busch, Sperling and Strauss2020). Halverson et al. (Reference Halverson, Hoffman, Schrag, Maloof and Rice2005) proposed a duration for the Shuram–Wonoka anomaly of between c. 590 Ma and c. 560 Ma. A shorter time interval (581 ± 6 Ma to 567 ± 6 Ma) was later inferred by an age model put forward by Retallack et al. (Reference Retallack, Marconato, Osterhout, Watts and Bindeman2014). New age data by Rooney et al. (Reference Rooney, Cantine, Bergmann, Gómez-Pérez, Al Baloushi, Boag, Busch, Sperling and Strauss2020) suggested a more constrained time bracket of between 574.0 ± 4.7 Ma and 567.3 ± 3.0 Ma. That analysis was based on Re–Os geochronology of organic-rich shales recovered from samples collected in NW Canada and Oman. According to such a result, the timing of the Shuram–Wonoka anomaly has been refined in Figure 11 (orange curve).
All in all, the Gaskiers glacial event does not seem to be a feasible driver of the Shuram–Wonoka event, because it is dated to between c. 581 Ma and 579 Ma (Pu et al. Reference Pu, Bowring, Ramezani, Myrow, Raub, Landing, Mills, Hodgin and Macdonald2016). Late Ediacaran glaciations also most probably did not contribute to such a negative anomaly. All available age data point to an onset of the Shuram–Wonoka anomaly after the Gaskiers glaciation. Within errors, the final regression of the anomaly overlaps slight with the Upper Ediacaran Glacial Period. Both glacial episodes bracket the anomaly, which means it took place in a warmer climate, and glacial influence is thus ruled out. Ediacaran glaciations do not seem to have impacted the ocean water chemistry.
Our age spectra of U–Pb ages of detrital zircons constrain all glacigenic sediment packages of the upper Granville Formation to the margin of the West African Craton (this study). The same is the case for all other glacial deposits within Cadomia (Linnemann et al. Reference Linnemann, Pidal, Hofmann, Drost, Quesada, Gerdes, Marko, Gärtner, Zieger, Ulrich, Krause, Vickers-Rich and Horak2018). This constrains the palaeoposition of the origin of the glacigenic tillites to within the Cadomian orogenic belt in southern latitudes at c. 60° S (Li et al. Reference Li, Bogdanova, Collins, Davidson, De Waele, Ernst, Fitzsimons, Fuck, Gladkochub, Jacobs, Karlstrom, Lu, Natapov, Pease, Pisarevsky, Thrane and Vernikovsky2008). Correlatives of the glaciations in Cadomia and West Africa are also present in Arabia and Iran. Such areas had in Ediacaran times palaeopositions at c. 30° S (Arabia) and close to the equator (Iran) (Li et al. Reference Li, Bogdanova, Collins, Davidson, De Waele, Ernst, Fitzsimons, Fuck, Gladkochub, Jacobs, Karlstrom, Lu, Natapov, Pease, Pisarevsky, Thrane and Vernikovsky2008). The spread of late Ediacaran glaciations is patchy and apparently independent of latitude. Most often, these deposits occur on the northern periphery of the Gondwana supercontinent (Fig. 11) and thus are coupled most probably to orogenic processes in peri-Gondwana and related to uplift of higher glaciated mountains in the hinterland of the depositional areas. A modern example of this kind is represented by the more than 2000 km long Patagonian ice sheet, which has been loading the shelf with glaciomarine deposits for the last 35 ka (Davies et al. Reference Davies, Darvill, Lovell, Bendle, Dowdeswell, Fabel, García, Geiger, Glasser, Gheorghiu, Harrison, Hein, Kaplan, Martin, Mendelova, Palmer, Pelto, Rodés, Sagredo, Smedley, Smellie and Thorndycraft2020).
Supplementary material
For supplementary material accompanying this paper visit https://doi.org/10.1017/S0016756821001011
Acknowledgements
The Senckenberg Gesellschaft für Naturforschung, the Leibniz-Gemeinschaft and the Sächsisches Staatsministerium für Wissenschaft, Kunst und Tourismus are thanked for permanent financial support of the GeoPlasmaLab (Senckenberg Naturhistorische Sammlungen Dresden). Daniel Le Heron (Universität Wien) and Marjorie Cantine (MIT, Boston) are thanked very much for their fruitful reviews which improved the paper considerably. Further, the authors thank very much Sören Jensen (Universidad de Extremadura, Badajoz) for helpful advice and his editorial work.