1. Introduction
According to several workers, along-strike variations in crustal shortening in the Andes are largely controlled by the dynamics of the subduction system and by the rheological and thermal structure of the South American plate. The shortening pattern recognized for the whole orogenic chain is characterized by a maximum at the central sector of the Central Andes in the order of 320 km (Isacks, Reference Isacks1988; Allmendinger et al. Reference Allmendinger, Jordan, Kay and Isacks1997; Kley, Monaldi & Saltify, Reference Kley, Monaldi and Saltify1999; Ramos, Reference Ramos and Caminos1999) and a decrease to the north and south. The origin of this pattern is still under debate, with researchers invoking different factors such as variations in the width of thermal weakening of the lithosphere by the asthenospheric wedge (Isacks, Reference Isacks1988), the age of the subducted plate (Ramos et al. Reference Ramos, Zapata, Cristallini, Introcaso and McClay2004; Yañez & Cembrano, Reference Yáñez and Cembrano2004), the development of flat subduction segments (Jordan et al. Reference Jordan, Isacks, Allmendinger, Brewer, Ramos and Ando1983; Isacks, Reference Isacks1988), the north to south subduction of oceanic ridges (Yañez et al. Reference Yáñez, Ranero, von Huene and Díaz2001), the presence of inherited heterogeneities and lithospheric strength variations in the upper plate (Tassara & Yañez, Reference Tassara and Yáñez2003; Babeyko & Sobolev, Reference Babeyko and Sobolev2005; Oncken et al. Reference Oncken, Hindle, Kley, Elger, Victor, Schemann, Oncken, Chong, Franz, Giese, Gotze, Ramos, Strecker and Wigger2006), enhanced climate-related erosion (Lamb & Davis, Reference Lamb and Davis2003), the presence of thick sedimentary basins promoting the formation of thin-skinned thrust belts (Allmendinger & Gubbels, Reference Allmendinger and Gubbels1996; Kley, Monaldi & Saltify, Reference Kley, Monaldi and Saltify1999) and the orogenic-scale dynamics of the lithosphere and subduction system (Russo & Silver, Reference Russo and Silver1996; Schellart et al. Reference Schellart, Freeman, Stegman, Moresi and May2007; Faccenna et al. Reference Faccenna, Becker, Conrad and Husson2013).
Superposed onto this continental-scale pattern, second-order variations of shortening are also observed (e.g. Giambiagi et al. Reference Giambiagi, Mescua, Bechis, Tassara and Hoke2012), as well as differences in the across-strike localization of shortening.
In this framework, the development of thin-skinned fold and thrust belts with high amounts of horizontal shortening is well documented throughout the Andes (Kley, Monaldi & Saltify, Reference Kley, Monaldi and Saltify1999). The control on the development of these thin-skinned belts is still a matter of debate, as well as how shortening in the basement is accommodated with respect to shortening in the sedimentary/volcaniclastic cover.
In this work we focus on the foreland fold and thrust belts of the segment between 30° and 36°S, where, following ideas presented in Kley, Monaldi & Saltify (Reference Kley, Monaldi and Saltify1999) and Ramos et al. (Reference Ramos, Zapata, Cristallini, Introcaso and McClay2004), we propose that the composition of the basement and the geometry of the extensional basins, features inherited from the Precambrian to early Mesozoic geologic history of the western Gondwana/South American margin, controlled Andean upper-crustal deformation and determined the development of thick- and thin-skinned belts and shortening localization.
2. Palaeozoic and Mesozoic evolution and the basement of the Southern Central Andes
The early Palaeozoic geologic evolution of the study area is characterized by the accretion of allochthonous terranes to the western margin of Gondwana (Fig. 1; Ramos et al. Reference Ramos, Jordan, Allmendinger, Mpodozis, Kay, Cortés and Palma1986). The Cuyania terrane is an exotic block of Laurentian origin (Thomas & Astini, Reference Thomas and Astini1996) that docked against western Gondwana in Ordovician time (Ramos, Dallmeyer & Vujovich, Reference Ramos, Dallmeyer, Vujovich, Pankhurst and Rapela1998; Thomas & Astini, Reference Thomas and Astini2003). The Chilenia terrane is a suspected allochthonous terrane of unknown origin, accreted to Gondwana in Devonian time (Ramos et al. Reference Ramos, Jordan, Allmendinger, Mpodozis, Kay, Cortés and Palma1986; Basei et al. Reference Basei, Ramos, Vujovich and Poma1998; López & Grégori, Reference López and Gregori2004; Massone & Calderón, Reference Massone and Calderón2008; Willner et al. Reference Willner, Gerdes, Massonne, Schmidt, Sudo, Thomson and Vujovich2011; Heredia et al. Reference Heredia, Farías, García-Sansegundo and Giambiagi2012). Early Palaeozoic sedimentation was predominantly marine, and the sediments are exposed in thrust sheets of the Precordillera range (Fig. 2).

Figure 1. Pre-Andean elements of the study area. The suture between terranes/microplates accreted to Gondwana in Palaeozoic time, the extent of Permo-Triassic Choiyoi magmatism and the basins developed during Palaeozoic and Mesozoic times are shown. Note that Palaeozoic basin development also took place in other sectors of the study area, only the Precordillera basin, not covered or intruded by Choiyoi magmatism, is shown.
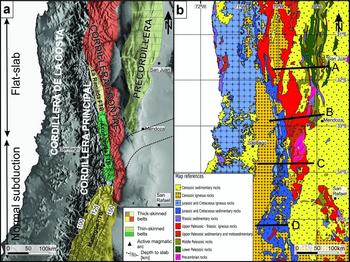
Figure 2. (a) Morphotectonic units of the Andes between 30° and 36°S, showing the fold and thrust belts of the Cordillera Principal and the structural style (thin- v. thick-skinned). The location of the present magmatic arc and slab depth contours from Cahill & Isacks (Reference Cahill and Isacks1992) are also shown, indicating the extent of flat-slab subduction in the study area. (b) Simplified geologic map of the Andes between 30° and 36°S, based on SEGEMAR (1997) and SERNAGEOMIN (2003). The location of the cross-sections of Figures 4 and 6 is also shown.
Retroarc basins developed throughout the region in Carboniferous – Early Permian times (Limarino & Spalletti, Reference Limarino and Spalletti2006). The sediments deposited in these basins were subsequently deformed along with older rocks during the Gondwanan orogeny (Keidel, Reference Keidel1916; Du Toit, Reference Du Toit1937; Cawood, Reference Cawood2005), locally known as the San Rafael phase (Azcuy & Caminos, Reference Azcuy, Caminos and Archangelsky1987; Ramos, Reference Ramos, Clark, Burchfield and Suppe1988).
The Late Permian – Early Triassic time period was characterized by a widespread extensional event associated with the initial break-up of Gondwana (Charrier, Reference Charrier1979; Llambías, Kleiman & Salvarredi, Reference Llambías, Kleiman, Salvarredi and Ramos1993). The acidic magmatic rocks of the Choiyoi Group (Llambías, Kleiman & Salvarredi, Reference Llambías, Kleiman, Salvarredi and Ramos1993; Sato et al. Reference Sato, Llambías, Basei and Castro2015) were formed during this event in west-central Argentina, covering a significant part of the study area (Figs 1, 2b).
In Late Triassic and Early Jurassic times, continued extension generated the isolated hemigrabens of the Cuyo basin and the initial depocentres of the Neuquén basin (Legarreta & Gulisano, Reference Legarreta, Gulisano, Chebli and Spalletti1989; Kokogián, Fernández Seveso & Mosquera, Reference Kokogián, Fernández Seveso, Mosquera and Ramos1993; Legarreta & Uliana, Reference Legarreta, Uliana and Caminos1999). By Middle Jurassic time, the depocentres of the Neuquén basin coalesced, and the basin achieved its maximum size (shown in Fig. 1 for the study area). Deposition continued during Late Jurassic and Early Cretaceous times with alternating marine and continental conditions. Andean uplift at these latitudes started in Late Cretaceous time (c. 90 Ma; Tunik et al. Reference Tunik, Folguera, Naipauer, Pimentel and Ramos2010; Di Giulio et al. Reference Di Giulio, Ronchi, Sanfilippo, Tiepolo, Pimentel and Ramos2012; Mescua, Giambiagi & Ramos, Reference Mescua, Giambiagi and Ramos2013; Balgord & Carrapa, Reference Balgord and Carrapa2016). The present topography of the Andes in the study area is the result of a Miocene to recent stage of deformation and uplift (Ramos, Reference Ramos and Caminos1999; Giambiagi et al. Reference Giambiagi, Mescua, Bechis, Tassara and Hoke2012; Buelow et al. Reference Buelow, Suriano, Mahoney, Kimbrough, Giambiagi and Mescua2015; Suriano et al. Reference Suriano, Mardonez, Mahoney, Giambiagi and Mescua2015).
As will be described in the following, the pre-late Triassic events resulted in the development of the basement of the Southern Central Andes, including a Precambrian metamorphic mafic–ultramafic basement of the Cuyania terrane, a metamorphic basement associated with the Chilenia terrane, of which information is scarce, and a volcanic–plutonic suite of predominantly acidic rocks of the Choiyoi Group. While the Choiyoi Group rocks are widely exposed throughout the study area, knowledge of the Cuyania and Chilenia basements comes from limited outcrops, complemented in the case of Cuyania with studies of xenoliths included in Miocene volcanic rocks and geophysical data. In the area affected by Choiyoi magmatic activity, Palaeozoic sedimentary rocks were covered and intruded by Choiyoi rocks. Therefore, Palaeozoic rocks were disrupted and appear today as discontinuous outcrops surrounded by Choiyoi rocks. In these areas, we consider them incorporated into the basement, following previous works (e.g. Kozlowski, Manceda & Ramos, Reference Kozlowski, Manceda, Ramos and Ramos1993; Manceda & Figueroa, Reference Manceda, Figueroa, Tankard, Suarez and Welsink1995; Kley, Monaldi & Saltify, Reference Kley, Monaldi and Saltify1999 and many others). In contrast, in sectors outside of the Permo-Triassic magmatic activity like the northern Precordillera (Fig. 1), the Palaeozoic sedimentary packages were not disrupted and are considered as part of the sedimentary cover (Cristallini & Ramos, Reference Cristallini and Ramos2000).
Since the Late Cretaceous deformational episode, the basement became involved in deformation in many sectors of the Andes, while in other sectors thin-skinned fold and thrust belts developed. The thick-skinned sectors include the Cordillera Frontal, the southern end of the Precordillera, and the Malargue and La Ramada fold and thrust belts of the Cordillera Principal (Figs 2, 3). Palaeozoic and Mesozoic sedimentary sequences were involved in Andean deformation as a result of the propagation of basement structures, locally forming thin-skinned fold and thrust belts like the northern Precordillera and the Aconcagua fold and thrust belt in the Cordillera Principal (Figs 2, 3). In the Precordillera, Andean structures partially reuse the pre-Andean (Gondwanan) decollement level according to some authors (e.g. Alonso et al. Reference Alonso, Rodríguez-Fernández, García-Sansegundo, Heredia, Farías and Gallástegui2005).
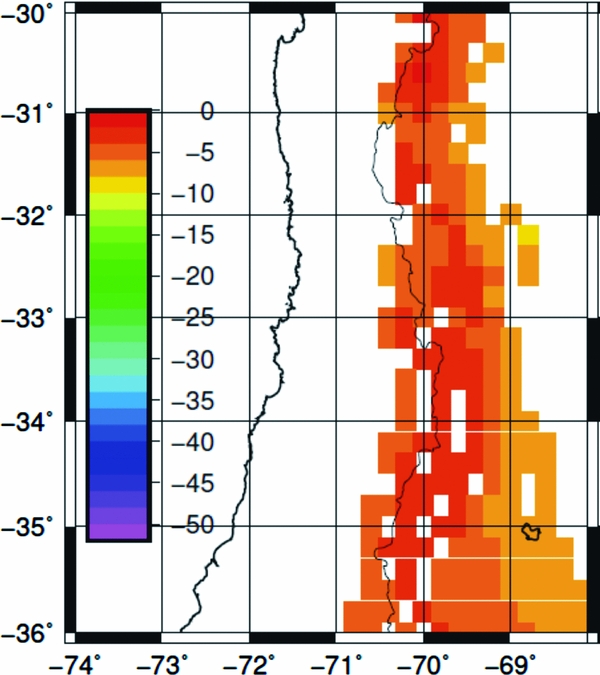
Figure 3. Depth in kilometres to the top of the weak ductile layer (interpreted as a detachment level) in the study area, obtained from the Tassara & Echaurren (Reference Tassara and Echaurren2012) crustal model. The colours represent the shallowest depth at which the condition ‘ductile yield strength < 100 MPa’ is satisfied. White areas correspond to areas in which that condition does not occur for the upper crust. See text for more details.
2.a. Basement of the Cuyania terrane
Traditionally, the Cuyania terrane has been interpreted as a composite terrane amalgamated during the Grenville orogeny, and accreted to Gondwana in Ordovician time. In this interpretation, two basement domains were identified in the terrane: the basement of the Precordillera Cambro-Ordovician carbonate platform and the Pie de Palo metamorphic rocks, separated by an ophiolitic belt (Fig. 1; Ramos, Reference Ramos2004; Vujovich, van Staal & Davis, Reference Vujovich, van Staal and Davis2004). This view has been challenged by Mulcahy et al. (Reference Mulcahy, Roeske, McClelland, Nomade and Renne2007), who support a Gondwanan origin of the Pie de Palo block. Irrespective of this issue, in this work we will take the Cuyania basement, including the Pie de Palo, as a unit, given its compositional uniformity as will be seen below.
In the Sierra de Pie de Palo (31°S, Fig. 1), whole-rock trace-element, field and petrographical studies indicated that the basement was composed by Grenvillian-age, predominantly mafic metamorphic rocks, the protoliths of which were formed in an oceanic subduction setting (Vujovich & Kay, Reference Vujovich, Kay, Pankhurst and Rapela1998; Ramos, Reference Ramos2010). Studies of xenoliths from Miocene volcanic rocks in the Precordillera arrived at similar conclusions, with geochemical data suggesting that the Precordillera basement protolith formed in an oceanic arc/back-arc environment (Kay, Orrel & Abruzzi, Reference Kay, Orrel and Abruzzi1996; Ramos, Reference Ramos2010).
Further evidence of the mafic composition of the Cuyania basement comes from geophysical studies. Alvarado, Beck & Zandt (Reference Alvarado, Beck and Zandt2007) carried out regional waveform modelling from crustal earthquakes, determining high Vp and Vp/Vs ratios for Cuyania basement rocks, which they interpreted as a signature of mafic and ultramafic rocks. Castro de Machuca et al. (Reference Castro de Machuca, Perarnau, Alvarado, López and Saez2012) and Pérez Luján et al. (Reference Pérez Luján, Ammirati, Alvarado and Vujovich2015) complemented seismological data with petrological analysis to determine a mafic–ultramafic composition extending to middle–lower crustal levels beneath the Pie de Palo and the central and western Precordillera. In contrast, Furlani (unpub. Ph.D. Thesis, Univ. Nacional de San Juan, 2014) interpreted a mafic upper crust underlain by a felsic middle to lower crust (below 15–20 km) based on tomography of local earthquakes.
2.b. Basement of the Chilenia terrane and igneous basement of the magmatic arc
The basement of Chilenia has been proposed to crop out in the Guarguaraz Complex in the Cordillera Frontal of Mendoza (Ramos et al. Reference Ramos, Jordan, Allmendinger, Mpodozis, Kay, Cortés and Palma1986; Massonne & Calderón, Reference Massone and Calderón2008; Ramos, Reference Ramos2010), where an assemblage of schists, gneisses, metasediments, metavolcanic rocks and ultramafic bodies is found (López & Gregori, Reference López and Gregori2004). U–Pb Grenvillian ages were determined on the gneisses by Ramos & Basei (Reference Ramos, Basei, Bradshaw and Weaver1997). More recently, these rocks have been reinterpreted as an accretionary prism in the limit between Cuyania and Chilenia or as sediments subducted along the suture zone between Cuyania and Chilenia forming a high-pressure collisional metamorphic complex (López et al. Reference López, Escayola, Azarevich, Pimentel and Tassinari2009; Willner et al. Reference Willner, Gerdes, Massonne, Schmidt, Sudo, Thomson and Vujovich2011). Álvarez et al. (Reference Álvarez, Mpodozis, Arriagada, Astini, Morata, Salazar, Valencia and Veervort2011) have studied the U–Pb ages of detrital zircons from late Palaeozoic accretionary complexes in central Chile, determining a very large subpopulation of Neoproterozoic – Early Cambrian zircons interpreted as coming from a source unrelated to Gondwana, which could represent an igneous source in the basement of Chilenia.
Overall, the composition of the Chilenia basement is usually assumed to be more felsic than that of Cuyania, although this is based on very limited information. In any case, the Palaeozoic, Mesozoic and Cenozoic magmatic arcs have intruded and fluxed the Chilenia basement, modifying it and incorporating plutonic additions, resulting in an average andesitic composition for the upper crust (Thorpe, Francis & Harmon, Reference Thorpe, Francis and Harmon1980). Therefore, in this work, we consider that the Chilenia/magmatic arc basement domain behaves as a block of andesitic composition, which is also supported by slower seismic velocities at lower crustal depths (Marot et al. Reference Marot, Monfret, Gerbault, Nolet, Ranalli and Pardo2014) and lower bulk crustal density (Tassara et al. Reference Tassara, Götze, Schmidt and Hackney2006; Tassara & Echaurren, Reference Tassara and Echaurren2012) compared with those exhibited by the Cuyania basement.
2.c. The rocks of the Choiyoi magmatic province
The Permo-Triassic Choiyoi acidic magmatic province (Fig. 1; Groeber, Reference Groeber1947) developed during a widespread extensional event in western Gondwana (Llambías, Kleiman & Salvarredi, Reference Llambías, Kleiman, Salvarredi and Ramos1993). It is recognized in outcrops and wellbores from northern Chile to south-central Argentina (Sato et al. Reference Sato, Llambías, Basei and Castro2015). In the study area, its rocks are well exposed in the Cordillera Frontal, where they predominate, as well as in the Cordillera Principal of southern Mendoza, and the southern end of the Precordillera (Fig. 2). Lithologically, the Choiyoi Group is dominated by acidic volcanic rocks and plutons (Kay et al. Reference Kay, Ramos, Mpodozis and Sruoga1989; Llambías, Kleiman & Salvarredi, Reference Llambías, Kleiman, Salvarredi and Ramos1993). It constitutes the basement of the Mesozoic Cuyo and Neuquén sedimentary basins, as well as the basement for Andean deformation (Kozlowski, Manceda & Ramos, Reference Kozlowski, Manceda, Ramos and Ramos1993).
In the Cordillera Frontal, the seismic tomography carried out by Furlani (unpub. Ph.D. thesis, Univ. Nacional de San Juan, 2014) determined that the felsic basement of the Choiyoi Group characterized the crust to depths of ~ 20 km.
2.d. The sedimentary cover and detachment levels
In the Precordillera range (Fig. 2), a Palaeozoic sedimentary sequence of more than 5 km of thickness is found, including Cambro-Ordovician platform carbonates, Ordovician to Devonian clastic marine deposits and Carboniferous–Permian continental and marine deposits, with a detachment level in the basal limestones (Ramos & Vujovich, Reference Ramos and Vujovich2000). Except for the northern Precordillera, in the rest of the study area, Palaeozoic units were deformed by the Late Permian San Rafael phase, and intruded by Choiyoi rocks, so that there is not a continuous sedimentary succession.
The Early–Middle Triassic Cuyo basin was developed in the central part of the study area (Fig. 1), with sediment deposition in continental environments controlled by normal faults reaching a maximum thickness of 3500 m (Kokogián, Fernández Seveso & Mosquera, Reference Kokogián, Fernández Seveso, Mosquera and Ramos1993). A potential detachment level is the black shales of the Cacheuta Formation, located in the upper part of the Cuyo basin sedimentary package; however, these rocks are only locally deformed and were not used as a major detachment during Andean deformation.
The Late Triassic – Cretaceous Neuquén basin is characterized by two sectors: a northern, narrow (~ 90 km wide) basin, denominated the Aconcagua basin, and a southern sector in which the basin extends to the east denominated the Neuquén Embayment. The study area includes the Aconcagua basin and the transitional part to the Neuquén Embayment (Fig. 1). Initial basin development took place in isolated grabens during latest Triassic – Early Jurassic times (Legarreta & Uliana, Reference Legarreta, Uliana and Caminos1999). In the study area, this first stage is recorded in synrift deposits in the La Ramada, Malargüe and the southwestern part of the Aconcagua fold thrust belts. The northern part of the Aconcagua fold thrust belt was a basement high until Middle Jurassic time (‘Alto del Tigre’, Álvarez, Reference Álvarez and Ramos1996). Neuquén basin sediments achieve maximum thicknesses of over 5000 m, with at least two regional levels suitable as detachments during Andean deformation: the gypsum of the Oxfordian Auquilco Formation and the black shales of the Neocomian Vaca Muerta Formation (Kozlowski, Manceda & Ramos, Reference Kozlowski, Manceda, Ramos and Ramos1993; Legarreta & Uliana, Reference Legarreta, Uliana and Caminos1999). Locally, other units with limited geographical distribution also worked as detachments.
3. The Andean orogeny and shortening distribution between 30° and 36°S
The rise of the Andes began in the study area in Late Cretaceous time (Mescua, Giambiagi & Ramos, Reference Mescua, Giambiagi and Ramos2013; Balgord & Carrapa, Reference Balgord and Carrapa2016), with deformation limited to the western part of the Cordillera Principal (Fig. 2a). The present topography of the Andean Cordillera is mainly the result of the Miocene to recent deformation (Ramos et al. Reference Ramos, Aguirre-Urreta, Álvarez, Cegarra, Cristallini, Kay, Lo Forte, Pereyra and Pérez1996; Ramos, Reference Ramos and Caminos1999; Giambiagi et al. Reference Giambiagi, Mescua, Bechis, Tassara and Hoke2012; Buelow et al. Reference Buelow, Suriano, Mahoney, Kimbrough, Giambiagi and Mescua2015; Suriano et al. Reference Suriano, Mardonez, Mahoney, Giambiagi and Mescua2015). The development of flat-slab subduction since ~ 12 Ma in the northern part of the study area (30–33°S; Fig. 2) is interpreted to have a major role in the evolution of Andean deformation (Jordan et al. Reference Jordan, Isacks, Allmendinger, Brewer, Ramos and Ando1983; Ramos, Cristallini & Pérez, Reference Ramos, Cristallini and Pérez2002). The cessation of magmatic activity in the arc and the uplift of the Sierras Pampeanas 700 km east of the trench are particular features of the flat-slab segment.
The morphotectonic units comprising the Andes in the study area are shown in Figure 2, indicating the structural style (basement v. cover deformation) developed in each. Many works have proposed that upper-crustal deformation in the study area developed over a regional detachment within the basement, but the location of this level varies depending on the different authors at between 20 and 10 km depth (Allmendinger et al. Reference Allmendinger, Figueroa, Synder, Beer, Mpodozis and Isacks1990; Kozlowski, Manceda & Ramos, Reference Kozlowski, Manceda, Ramos and Ramos1993; Manceda & Figueroa, Reference Manceda, Figueroa, Tankard, Suarez and Welsink1995; Kley, Monaldi & Saltify, Reference Kley, Monaldi and Saltify1999; Cristallini & Ramos, Reference Cristallini and Ramos2000; Farías et al. Reference Farías, Comte, Charrier, Martinod, David, Tassara, Tapia and Fock2010).
As for some of our previous works (Giambiagi et al. Reference Giambiagi, Mescua, Bechis, Tassara and Hoke2012, Reference Giambiagi, Spagnotto, Moreiras, Gómez, Stahlschmidt and Mescua2015 b), we use the geophysically constrained three-dimensional model of lithospheric and crustal structure along the Andean margin presented by Tassara & Echaurren (Reference Tassara and Echaurren2012) in order to obtain a thermomechanical representation that can be used for estimating the geometry of potential crustal detachments. As explained elsewhere (see details in Giambiagi et al. Reference Giambiagi, Mescua, Bechis, Tassara and Hoke2012, Reference Giambiagi, Tassara, Mescua, Tunik, Alvarez, Godoy, Hoke, Pinto, Spagnotto, Porras, Tapia, Jara, Bechis, García, Suriano, Pagano, Sepúlveda, Giambiagi, Moreiras, Pinto, Tunik, Hoke and Farías2015 b), the 3D geometry of the Lithosphere–Asthenosphere Boundary (LAB) of Tassara & Echaurren (Reference Tassara and Echaurren2012) is considered an isotherm of 1350°C. Thermal conduction with radiogenic heat production in the upper crust is assumed in order to compute the 1D geotherms that can be extrapolated in a 3D temperature field; each geotherm is then used along with constitutive rheologic laws for brittle and thermally dependent ductile deformation in order to construct 1D yield strength envelopes (Burov & Diament, Reference Burov and Diament1995). After extrapolation in 3D, this thermomechanical model predicts the possible rheological (brittle–elastic–ductile) behaviour of crust and mantle under loading by differential tectonic stresses and can be used to identify weak ductile mechanical layers inside the crust, where upper-crustal faults are likely rooted. The model is built with a 2 km vertical resolution. For high geothermal gradients at the Cordilleran axis, the intracrustal discontinuity separating upper and lower crust in the original model of Tassara & Echaurren (Reference Tassara and Echaurren2012) serves as the base of a weak ductile layer located in the upper crust, with a predicted ductile strength lower than the average differential stress caused by far-field tectonic forces of 100 MPa (Coblentz & Richardson, Reference Coblentz and Richardson1996). Therefore, this crustal weak zone would flow under this tectonic load, allowing its possible use as a mechanical detachment level for upper-crustal faults. Figure 3 shows a map of the depth (with respect to sea level) to the top of such a weak layer, located at between ~ 3 and 10 km in the study area. The bottom of the weak layer (see Fig. 4) is located at a depth of between ~ 5 and 18 km. The cross-sections presented in Figure 4, which were built without taking into account the thermomechanical models, show an upper-crustal detachment located at depths similar or slightly deeper than the weak ductile layer. Taking into account the vertical resolution of 2 km in the thermomechanical model and the fact that this model is based on present conditions, we consider that overall, both detachment estimations (structural and geophysical) are consistent. We can thus assume that the depth to detachment within the basement during Andean deformation is rooted at less than 18–20 km throughout the study area. We can therefore characterize the basement for Andean deformation based on the geophysical studies detailed in Sections 2a–c.

Figure 4. Cross-sections of the Andean orogen between 30° and 36°S, indicating shortening distribution across-strike. See text for details. (a) Cross-section at 32°S (based on Cristallini & Ramos, Reference Cristallini and Ramos2000). Inset shows the thin-skinned Río San Juan cross-section of the Precordillera (according to the same authors). (b) Cross-section at 33°S (Based on Cegarra & Ramos, Reference Cegarra, Ramos and Ramos1996; Giambiagi et al. Reference Giambiagi, Mescua, Bechis, Martínez and Folguera2011; Jara et al. Reference Jara, Likerman, Winocur, Ghiglione, Cristallini, Pinto, Charrier, Sepúlveda, Giambiagi, Moreiras, Pinto, Tunik, Hoke and Farías2015). Inset shows the northern thin-skinned Aconcagua fold and thrust belt based on Cegarra & Ramos (Reference Cegarra, Ramos and Ramos1996). (c) Cross-section at 33°40’S (based on Giambiagi et al. Reference Giambiagi, Tassara, Mescua, Tunik, Alvarez, Godoy, Hoke, Pinto, Spagnotto, Porras, Tapia, Jara, Bechis, García, Suriano, Pagano, Sepúlveda, Giambiagi, Moreiras, Pinto, Tunik, Hoke and Farías2015b ). Inset shows the southern Aconcagua fold and thrust belt with a mixed thin- and thick-skinned deformation. (d) Cross-section at 35°S in the thick-skinned Malargüe fold and thrust belt (based on Mescua et al. Reference Mescua, Giambiagi, Tassara, Giménez and Ramos2014). Inset shows a detail of the Las Leñas area, where Andean thrusts uplift the Choiyoi basement.
We will analyse the upper-crustal shortening distribution across the strike of the orogen for selected cross-sections of the foreland fold and thrust belts (east of the Miocene to present magmatic arc). Figure 4 displays the structural style as well as the shortening distribution across each transect. The northern transects (A and B in Fig. 2) correspond to the flat-slab segment, transect D is in the southern ‘normal’ subduction segment, and transect C is located in the transition. The along-strike decrease in shortening to the south in the study area is likely the result of flat-slab development in the north.
In the northern transect (32°S), Cristallini & Ramos (Reference Cristallini and Ramos2000) estimated 15 km of shortening in the La Ramada fold and thrust belt in the eastern Cordillera Principal, over Chilenia and Choiyoi basements, and 4 to 6 km in the Cordillera Frontal developed on Choiyoi basement (Fig. 4a). Shortening along this transect is mainly concentrated in the Precordillera, where the Palaeozoic sedimentary units form a thin-skinned fold and thrust belt over Cuyania basement. The basement here is not involved in the outcropping structures. Unlike the rest of the study area, in the northern Precordillera the Palaeozoic structures were not covered by Choiyoi magmatism. Balanced cross-sections by different workers have estimated shortening between 88 and 136 km (Von Gosen, Reference Von Gosen1992; Cristallini & Ramos, Reference Cristallini and Ramos2000), although the Precordillera reactivates a late Palaeozoic thrust belt developed during the San Rafael phase, and there is some debate on the proportion of Cenozoic v. Palaeozoic shortening. Alonso et al. (Reference Alonso, Rodríguez-Fernández, García-Sansegundo, Heredia, Farías and Gallástegui2005) and Álvarez Marrón et al. (Reference Álvarez Marrón, Rodríguez-Fernández, Heredia, Busquets, Colombo and Brown2006) have proposed that most shortening in the Precordillera is pre-Andean. According to these authors, Andean shortening would be less than half that previously proposed (45 km of Andean shortening for the San Juan River cross-section at 31°30’S; Alonso et al. Reference Alonso, Rodríguez-Fernández, García-Sansegundo, Heredia, Farías and Gallástegui2005). In spite of this discussion, there is a consensus on the structural style, corresponding to a thin-skinned fold and thrust belt for both pre-Andean and Andean deformation, and on the higher shortening in the Precordillera than in the Cordillera Principal and Frontal.
The Aconcagua transect (33°S) presents a quite different shortening distribution across strike (Fig. 4b). The Aconcagua fold and thrust belt of the Cordillera Principal, with a thin-skinned deformation of Neuquén basin sediments, absorbed most of the shortening, calculated by Cegarra & Ramos (Reference Cegarra, Ramos and Ramos1996) as ~ 63 km. A few more kilometres (< 10 km) of shortening in the Western Cordillera Principal can be deduced from the cross-sections of Jara et al. (Reference Jara, Likerman, Winocur, Ghiglione, Cristallini, Pinto, Charrier, Sepúlveda, Giambiagi, Moreiras, Pinto, Tunik, Hoke and Farías2015). Chilenia/magmatic arc and Choiyoi basement underlies this fold thrust belt. The transition between both basement domains is not well documented, since the western extent of Choiyoi magmatism in the subsurface is not clear. Shortening for the Cordillera Frontal is estimated at ~ 15–20 km, and the southern Precordillera only shows ~ 10 km of shortening (Giambiagi et al. Reference Giambiagi, Mescua, Bechis, Martínez and Folguera2011). Both morphotectonic units are developed on Choiyoi basement in this area, without a well-developed, continuous sedimentary cover. Furthermore, the southern Precordillera was affected by normal faults during the Triassic development of the Cuyo basin, disrupting the possible detachment levels of the Palaeozoic sediments.
The distribution of shortening along the Maipo–Tunuyán transect (33°40’S) is similar to the Aconcagua transect, but displays smaller amounts of shortening in each morphotectonic unit (Fig. 4c; Giambiagi et al. Reference Giambiagi, Tassara, Mescua, Tunik, Alvarez, Godoy, Hoke, Pinto, Spagnotto, Porras, Tapia, Jara, Bechis, García, Suriano, Pagano, Sepúlveda, Giambiagi, Moreiras, Pinto, Tunik, Hoke and Farías2015b ). The Cordillera Principal, developed over Chilenia and Choiyoi basement with a Neuquén basin sedimentary cover, shows ~ 50 km of shortening, and the Cordillera Frontal, developed over Choiyoi basement without a sedimentary cover, shows ~ 15 km of shortening; the Precordillera is replaced at this latitude by limited inversion of the Cuyo basin developed over Choiyoi basement, accumulating less than 5 km of shortening (Giambiagi et al. Reference Giambiagi, Spagnotto, Moreiras, Gómez, Stahlschmidt and Mescua2015a ).
South of 34°S, the Cordillera Frontal sinks below Cenozoic sediments, and the Andes comprise only the Cordillera Principal, as shown by the Rio Salado transect (35°S). This cross-section (Fig. 4d), taken as an example of the structural style and shortening of the thick-skinned Malargüe fold and thrust belt, where Choiyoi basement is overlain by a Neuquén basin sedimentary cover, presents a combination of tectonic inversion of Mesozoic normal faults and thrusts developed during Cenozoic time (Kozlowski, Manceda & Ramos, Reference Kozlowski, Manceda, Ramos and Ramos1993; Manceda & Figueroa, Reference Manceda, Figueroa, Tankard, Suarez and Welsink1995; Mescua & Giambiagi, Reference Mescua and Giambiagi2012; Mescua et al. Reference Mescua, Giambiagi, Tassara, Giménez and Ramos2014). Along the Malargüe fold and thrust belt, shortening decreases to the south from 27 to 10 km (Giambiagi et al. Reference Giambiagi, Mescua, Bechis, Tassara and Hoke2012).
A summary of shortening variations in the study area and basement features is presented in Table 1.
Table 1. Basement, cover, structural style and shortening variations in the morphotectonic units of the study area

4. Estimation of the strength of the different basement domains and sedimentary rocks
In upper-crustal conditions, deformation is controlled by brittle fracture or frictional sliding (Jaeger, Reference Jaeger1969; Sibson, Reference Sibson1974, Reference Sibson1977).
Rock strength calculations for brittle failure can be made using the Mohr–Coulomb failure criterion, but that requires extensive laboratory testing of samples to determine the cohesive strength and coefficient of internal friction (Jaeger & Cook, Reference Jaeger and Cook1979; Zoback, Reference Zoback2010), data which is not available for the different rock types of the study area. General compilations indicate that properties of sandstones and granitic rocks overlap, while mafic metamorphic rocks are stronger (Carmichael, Reference Carmichael1982). A simplified version of the failure criterion, the linearized Mohr–Coulomb envelope (Colmenares & Zoback, Reference Colmenares and Zoback2002), can be used to describe the strength of rocks based on two parameters: the coefficient of internal friction (μi ) and the unconfined compressive strength (UCS).
Some empirical formulations have been developed to relate the UCS of a rock to different properties such as P-wave velocity (Vp), density (ρ), Young's modulus (E), etc. This approach to calculating rock strength implies that the coefficient of internal friction is of secondary importance because even weak rocks have high internal friction (Zoback, Reference Zoback2010), and therefore UCS is a good representation of rock strength. Average values of some of these properties are available for rocks in the study area from a number of geophysical studies (as detailed below and in Table 2). We will use the equation proposed by Freyburg (Reference Freyburg1972):

Table 2. Density and P-wave velocity of basement and sedimentary rocks in the study area. Results for unconfined compressive strength (UCS) using equation (1)

as a first-order approximation of rock strength to brittle failure in the study area.
However, in most cases, the strength of rocks in the upper crust responds to frictional sliding along a pre-existing surface, since these rocks usually have inherited structures. In our case study, basement and sedimentary rocks have experienced long histories of tectonic activity before Andean deformation that generated fractures of different directions (e.g. Von Gosen, Reference Von Gosen1992; Giambiagi et al. Reference Giambiagi, Mescua, Bechis, Martínez and Folguera2011; Mescua & Giambiagi, Reference Mescua and Giambiagi2012). A linear frictional failure criterion applies, which can be defined in terms of the differential stress, and allows us to estimate rock strength from density as (Sibson, Reference Sibson1974; Ranalli & Murphy, Reference Ranalli and Murphy1987):

where σ1 and σ3 are maximum and minimum compressive stresses, respectively, g the acceleration due to gravity (9.8 m s−2), z is depth (km), λ the pore fluid factor (ratio of pore fluid pressure to overburden pressure) and β a parameter depending on the type of faulting regime, with values of 3, 1.2 and 0.75 for thrust, strike-slip and normal faulting regimes, respectively, if a friction coefficient of 0.6 is assumed (following Byerlee, Reference Byerlee1978). Therefore, the frictional failure criterion defines a pressure-dependent mechanism, unaffected by temperature. Assuming hydrostatic pore fluid pressure, the main control on upper-crustal rock strength for a particular faulting regime is rock density. Estimates obtained using equation (2) are conservative, since we use the same friction value of 0.6 (the lower value indicated by Byerlee, Reference Byerlee1978) for all rocks.
We calculated the strength of the different basement and sedimentary rocks to brittle failure and frictional sliding using equations (1) and (2) and Vp and ρ estimates for Cuyania basement rocks (Pérez Luján et al. Reference Pérez Luján, Ammirati, Alvarado and Vujovich2015), Precordillera Palaeozoic sedimentary rocks (Pérez Luján et al. Reference Pérez Luján, Alvarado, Guell, Sáez and Vujovich2013; Perucca & Ruiz, Reference Perucca and Ruiz2014), Choiyoi basement and Neuquén basin sedimentary rocks (Rojas Vera et al. Reference Rojas Vera, Folguera, Zamora Valcarce, Giménez, Ruiz, Martínez, Bottesi and Ramos2010), and Cuyo basin sedimentary rocks (Miranda & Robles, Reference Miranda and Robles2002), as well as the theoretical calculations of density for different lithologies and their variation with depth of Tassara (Reference Tassara2006). The data are shown in Table 2, and results are summarized in Figure 5. In all those works, Vp estimates are given for certain depths, and we only calculated strength from equation (1) for the depths reported in those publications. In contrast, equation (2) allowed us to estimate a continuous curve for frictional rock strength variations with depth, assuming that density variations for each unit are well represented by the values shown in Table 2. It must be noted that the sedimentary successions contain shale and gypsum horizons that do not respond to these strength calculations, being very weak rocks that act as ductile detachment levels (see Section 2.d).

Figure 5. Strength against frictional sliding of basement and sedimentary rocks with depth. When two curves are shown for a rock unit, they correspond to the maximum and minimum density values indicated in geophysical works as shown in Table 2. (a) Strength contrast between the strong Cuyania basement and the Precordillera Palaeozoic basement rocks, which favoured the development of the thin-skinned northern Precordillera. (b) Strength contrast between the Chilenia/magmatic arc basement, which favoured the development of the thin-skinned Aconcagua fold and thrust belt. (c) Lack of strength contrast between the Choiyoi basement and the Neuquén basin rocks, which favoured basement involvement in the deformation.
5. Results
Our calculations show that the strength of the basement is highly heterogeneous in the study area, consistent with the average composition of the rocks of the different domains.
Our results for UCS to brittle fracture (Table 2) indicate that, consistent with their mafic–ultramafic composition, the strongest rocks in the area correspond to the basement of Cuyania. For depths of 10–18 km, estimates of UCS vary between 196 and 214 MPa. Unfortunately, Vp data is only available for depths of a few metres (< 50 m) for Precordillera sedimentary rocks; these data indicate weak rocks with UCS up to 108 MPa. While strength of the Palaeozoic sediments must increase with depth, it is unlikely that high values like those of the basement are reached. The results for Choiyoi basement rocks indicate UCS between 178.5 and 185.5 MPa, values similar to Neuquén basin sedimentary rocks (143.5–178.5 MPa). Cuyo basin rocks are the weakest in the study area. Equation (1) could not be used for Chilenia/magmatic arc rocks, since no Vp data were available for the northern part of the study area, and in the southern part, the development of the present magmatic arc results in low Vp owing to the presence of melts.
The results of strength to frictional sliding were calculated using equation (2). Figure 5 shows the variations in rock strength with depth for the different morphotectonic units in each cross-section. The results are similar to those obtained for brittle fracture. Cuyania basement contains the strongest rocks, 25–30% stronger than the sediments of the Palaeozoic basins of the Precordillera. In contrast, the strength of Choiyoi basement is relatively weak, with a similar strength to the overlying Neuquén basin sediments and the Palaeozoic basins of the Precordillera. Chilenia basement shows an intermediate strength, being 13% stronger than Neuquén basin sediments. Like in the previous case, the weakest rocks in the study area are Cuyo basin sediments.
6. Discussion
6.a. Control of basement composition on Andean deformation
Our results suggest that second-order along-strike and first-order across-strike distribution of Andean shortening in the study area can be a result of the basement lithologies and their strength contrast with the overlying sedimentary rocks (Fig. 6). The first-order N–S variation of shortening is arguably controlled by the behaviour of the subduction system (Russo & Silver, Reference Russo and Silver1996; Schellart et al. Reference Schellart, Freeman, Stegman, Moresi and May2007; Faccenna et al. Reference Faccenna, Becker, Conrad and Husson2013), and in particular, the strong gradient in shortening between the Aconcagua, Maipo–Tunuyán and Malargüe transects is likely the result of the development of the Pampean flat-slab since 12 Ma in the northern sector (Jordan et al. Reference Jordan, Isacks, Allmendinger, Brewer, Ramos and Ando1983; Ramos, Cristallini & Pérez, Reference Ramos, Cristallini and Pérez2002). The development of flat-slab segments likely results in increased interplate coupling (Gutscher, Reference Gutscher2002), favouring higher shortening.
In contrast, we postulate that the across-strike localization of shortening is related to basement v. cover dominated deformation. Areas with thick-skinned tectonics show relatively smaller amounts of shortening, and thin-skinned fold and thrust belts absorb most of the shortening in each cross-section. We propose that this relationship is the reflection of the strength of the different basement domains, and that high shortening amounts correspond to areas with the highest contrast between basement and cover strength.
High shortening belts in the study area correspond to (i) the northern Precordillera and (ii) the Aconcagua fold and thrust belt (Figs 2, 5, 6). In the first, Palaeozoic sediments overlie the mafic basement of the Cuyania terrane, with the highest contrast in strength of the whole area. Our calculations for frictional sliding indicate a contrast of 25–30%, increasing from ~ 20–25 MPa at shallow depths to ~ 100–120 MPa at 5 km of depth in the basement–cover contact. In the second case, the Neuquén basin sediments overlie Chilenia/magmatic arc basement that is 13% stronger according to our calculations.
In contrast, regions of thick-skinned deformation such as the La Ramada and Malargüe fold and thrust belts of the Cordillera Principal, the Cordillera Frontal and the southern Precordillera present relatively minor shortening amounts (Figs 2, 5, 6). This is likely the result of the predominance of tectonic inversion as the mechanism of basement deformation (Manceda & Figueroa, Reference Manceda, Figueroa, Tankard, Suarez and Welsink1995; Kley, Monaldi & Saltify, Reference Kley, Monaldi and Saltify1999; Mescua & Giambiagi, Reference Mescua and Giambiagi2012), in which high-angle reverse faults produce uplift rather than horizontal shortening. In all these sectors, basement corresponds to the Choiyoi Group, and the strength contrast between the basement and the sedimentary cover of the Neuquén basin, where this was deposited, was small or inexistent. This likely hindered the development of a thrust belt with thin-skinned deformation and decreased the shortening of individual structures in these sectors. In this way, the La Ramada fold thrust belt at 32°S only accumulated ~ 15 km of shortening, and at that latitude shortening was concentrated further east in the Precordillera, where a thin-skinned belt could develop. In the Malargüe fold thrust belt, in southern Mendoza (34–36°S), a wider mountain range with thick-skinned deformation was developed to absorb the shortening determined by the subduction system behaviour at these latitudes. Cover deformation was limited between uplifted basement blocks. In the eastern piedmont of the Mendoza province, the Cuyo basin contains the weakest rocks according to our data, over Choiyoi and Cuyania basement. We propose that the sediments of the Cuyo basin did not develop a thin-skinned fold thrust belt because shortening at these latitudes was already accumulated in the Cordillera Principal. In the Cordillera Frontal, where no basin was developed, Choiyoi basement was uplifted reaching altitudes of 4000–6000 m, with little horizontal shortening.
6.b. Control of basin development on deformation
The geometry and characteristics of the infill of the Mesozoic basins, especially the Neuquén basin, may also have played a role in the shortening distribution during Andean deformation. In the Aconcagua area, the Neuquén basin was formed by a single, relatively narrow, N-trending depocentre, and its infill contained a major detachment level in the weak horizon of Oxfordian gypsum (Auquilco Formation: Kozlowski, Manceda & Ramos, Reference Kozlowski, Manceda, Ramos and Ramos1993). These factors probably favoured the development of the thin-skinned Aconcagua fold and thrust belt, with 50–60 km of shortening (Cegarra & Ramos, Reference Cegarra, Ramos and Ramos1996; Giambiagi et al. Reference Giambiagi, Tassara, Mescua, Tunik, Alvarez, Godoy, Hoke, Pinto, Spagnotto, Porras, Tapia, Jara, Bechis, García, Suriano, Pagano, Sepúlveda, Giambiagi, Moreiras, Pinto, Tunik, Hoke and Farías2015b ), in addition to the contrast between the Chilenia/magmatic arc basement and the basin fill in its western margin. The fold thrust belt overlies Choiyoi basement of the Cordillera Frontal in its eastern margin, with basement thrusts developed in the eastern front of this morphotectonic unit (Figs 2, 4).
In contrast, the Maipo–Tunuyán transect in the southern Aconcagua fold and thrust belt presents a hybrid thick- and thin-skinned deformation, with shortening decreasing to less than 50 km (Giambiagi et al. Reference Giambiagi, Tassara, Mescua, Tunik, Alvarez, Godoy, Hoke, Pinto, Spagnotto, Porras, Tapia, Jara, Bechis, García, Suriano, Pagano, Sepúlveda, Giambiagi, Moreiras, Pinto, Tunik, Hoke and Farías2015b ). The western sector, where an Early Jurassic extensional depocentre was developed, is characterized by the inversion of the Mesozoic normal faults (Fig. 4).
Towards the south, the Malargüe fold and thrust belt is located in the transition to the Neuquén Embayment, where the Neuquén basin was wider and developed over Choiyoi basement, with little strength contrast to the basin fill. This resulted in basement involvement in the deformation, with thin-skinned structures developed locally in response to the propagation of basement faults (Kozlowski, Manceda & Ramos, Reference Kozlowski, Manceda, Ramos and Ramos1993). Furthermore, in some sectors the Neuquén basin consisted of two extensional depocentres divided by a basement high (Gerth, Reference Gerth1931; Legarreta & Kozlowski, Reference Legarreta and Kozlowski1984; Mescua et al. Reference Mescua, Giambiagi, Tassara, Giménez and Ramos2014), with thickness and facies variations of the weak units that may have acted as detachment levels hindering the development of a thin-skinned thrust belt. While this basin geometry and the inversion of weak Mesozoic normal faults likely contributed to thick-skinned tectonics in the Malargüe fold and thrust belt, the basement is also involved in the deformation and uplifted as major basement blocks by Andean thrusts in some areas (e.g. Bardas Blancas: Dimieri, Reference Dimieri1997; Las Leñas: Mescua et al. Reference Mescua, Giambiagi, Tassara, Giménez and Ramos2014), indicating that the strength of Choiyoi basement, with little contrast to Neuquén basin sediments, was the main control. This is even clearer south of the study area, in the Andes of Neuquén province, where the Neuquén basin presents thicknesses of 4–6 km extending well into the foreland. In spite of this important basin thickness, Choiyoi basement is involved in the deformation, through both the inversion of Mesozoic normal faults and the formation of Andean thrusts (e.g. the Agrio fold and thrust belt, 38–39°S; Rojas Vera et al. Reference Rojas Vera, Folguera, Zamora Valcarce, Giménez, Ruiz, Martínez, Bottesi and Ramos2010).
7. Concluding remarks
Several researchers have argued that the existence of a thick, continuous sedimentary basin and the presence of extensional basin depocentres were the main factors controlling the development of thin-skinned and thick-skinned belts, respectively (e.g. Allmendinger & Gubbels, Reference Allmendinger and Gubbels1996; Kley, Monaldi & Saltify, Reference Kley, Monaldi and Saltify1999).
The analysis of rock strength variations in the Southern Central Andes indicates that thin-skinned thrust belts with high amounts of shortening developed where the basement was strong. Therefore, a basement with a high strength contrast to the overlying sedimentary basin seems to be a requisite for the formation of thin-skinned belts, hindering basement involvement in the deformation. This is the case for Cuyania basement and Precordillera Palaeozoic rocks, and for Chilenia/magmatic arc basement and Neuquén basin rocks.
In areas where thick sedimentary basins overlie weak basement (with strength similar to basin rocks), thick-skinned deformation took place. While basement involvement can be favoured by weak pre-existing normal faults, in some areas it is the result of Andean thrusts, indicating that the basement is not significantly stronger that its cover. Our strength calculations for Choiyoi basement and Neuquén basin sediments support this idea.
In this way, while the total shortening for each transect is a result of the dynamics of the subduction system, the across-strike shortening distribution in the foreland thrust belts of the Andes between 30 and 36°S is controlled by the strength contrast between the different basement domains and the sedimentary cover. A secondary contribution by the geometry of the depocentres of the Neuquén basin is also supported.
Acknowledgments
This research was supported by CONICET (grant PIP 638) and the Agencia de Promoción Científica y Tecnológica (grant PICT-2011-1079). We thank comments by Dr Jonas Kley and an anonymous reviewer which allowed us to improve this paper.