1. Introduction
Well-preserved, fine-grained deltaic systems reveal links between oceanographic conditions, input, dispersal and biologic mixing of a wide range of grain sizes that together control the distribution of sedimentary facies on the continental shelf (e.g. Aigner & Reineck, Reference Aigner and Reineck1982; Potter et al. Reference Potter, Maynard and Depetris2005; Clifton, Reference Clifton, Posamentier and Walker2007; Milligan et al. Reference Milligan, Ogston, Puig, Scully, Traykowski, Wheatcroft, Nittrouer, Austin, Field, Kravitz, Syvitski and Wiberg2007; Plint et al. Reference Plint, Macquaker and Varban2012; Hart et al. Reference Hart, Macquaker and Taylor2013; Plint, Reference Plint2014; Poyatos-Moré et al. Reference Poyatos-Moré, Jones, Brunt, Hodgson, Wild and Flint2016; Schieber, Reference Schieber2016; Laycock et al. Reference Laycock, Pedersen, Montgomery and Spencer2017; Li & Schieber, Reference Li and Schieber2018). Relative to our understanding of sand-rich sedimentary facies, the products of muddy sediment transport and deposition are less well understood, even in modern settings (Wright et al. Reference Wright, Friedrichs, Kim and Scully2001; Cattaneo et al. Reference Cattaneo, Correggiari, Langone and Trincardi2003, Reference Cattaneo, Trincardi, Asioli and Correggiari2007; Jaramillo et al. Reference Jaramillo, Sheremet, Allison, Reed and Holland2009; Hanebuth et al. Reference Hanebuth, Lantzsch and Nizou2015; Denommee et al. Reference Denommee, Bentley, Harazim and Macquaker2016; Eidam et al. Reference Eidam, Nittrouer, Ogston, DeMaster, Liu, Nguyen and Nguyen2017; Shchepetkina et al. Reference Shchepetkina, Gingras, Zonneveld and Pemberton2017; Shanmugam, Reference Shanmugam2018). The reason for this knowledge gap is that primary mud depositional fabrics, such as lamina- and bedding-, are (a) difficult to observe with the naked eye (e.g. Schieber, Reference Schieber1999; Potter et al. Reference Potter, Maynard and Depetris2005) and (b) frequently overprinted by post-depositional processes including bioturbation and compaction (e.g. Egenhoff & Fishman, Reference Egenhoff and Fishman2013), as well as by other diagenetic processes including dissolution, alteration and replacement (Hower et al. Reference Hower, Eslinger, Hower and Perry1976; Bjørlykke, Reference Bjørlykke1998; Bentley et al. Reference Bentley, Sheremet and Jaeger2006; Bhattacharya & MacEachern, Reference Bhattacharya and MacEachern2009; Buatois etal., Reference Buatois, Saccavino, Zavala, Slatt and Zavala2011; Harazim et al. Reference Harazim, McIlroy, Edwards, Wogelius, Manning, Poduska, Layne, Sokaras, Alonso-Mori and Bergmann2015; Harazim & McIlroy, Reference Harazim and McIlroy2015).
This contribution reaches beyond the approaches developed for describing sandstone and systematically investigates the compositional, diagenetic and textural alterations of early Palaeozoic mudstone as a function of hydrodynamic sorting and reworking along an onshore–offshore dispersal path. The Cambrian–Ordovician succession on Bell Island (Fig. 1) preserves >1 km-thick siliciclastic sandstone and mudstone that were deposited by wave-, current- and gravity-driven processes in a shallow marine setting (Ranger et al. Reference Ranger, Pickerill and Fillion1984; Brenchley et al. Reference Brenchley, Pickerill and Stromberg1993; Harazim & McIlroy, Reference Harazim and McIlroy2015). Since studies focused on the sedimentary evolution of early Palaeozoic mud clinothems are extremely rare, the Power Steps Formation serves as a new key archive for understanding early Palaeozoic shelf-building processes where most of the detrital components have been delivered via mechanical weathering from a non-vegetated hinterland. As part of the Power Steps Formation, the fully exposed muddy Ochre Cove clinothem (OCC, Figs 1, 2) offers a unique opportunity to investigate (1) the nature and varying mechanisms of sediment dispersal across an early Ordovician clinothem and (2) how post-depositional changes, in particular burial diagenesis, govern the distribution of chlorite and illite cement across the OCC.

Fig. 1. (a) Topographic map of Eastern Newfoundland, indicating the location of Bell Island inside Conception Bay. (b) Simplified geological map of Bell Island, Newfoundland, with the location of the study area at Ochre Cove (black star).

Fig. 2. Generalized stratigraphic log of the OCC exposure at Ochre Cove, Bell Island, Newfoundland, indicating the stratigraphic position of facies, sampling locations for QXRD analyses and interpreted depositional environments.
2. Study area and geological overview
The study area is located in the Avalon Zone, the easternmost tectonostratigraphic zone of the Newfoundland Appalachians (Williams, Reference Williams1979; Van Staal et al. Reference Van Staal, Barr and Murphy2012), and consists of three major assemblages of predominantly late Precambrian to Lower Cambrian volcanic, volcaniclastic and sedimentary rocks. In the eastern part of the Conception Bay area, the Lower Ordovician (Arenigian ∼478–472 Ma) interval is preserved and exposed at Ochre Cove on Bell Island. This interval records the transition between the Bell Island and Wabana Groups (Figs 1, 2). During early Ordovician times, the Avalon Zone is suggested to be located on the western margin of Gondwana (Cocks & Torsvik, Reference Cocks and Torsvik2002; Van Staal et al. Reference Van Staal, Barr and Murphy2012). Rapid subsidence and contemporaneous sea-level rise (Austermann, Reference Austermann2016) provided the accommodation necessary to deposit the deep- to shallow-marine Bell Island and Wabana Group strata, which are presumed to unconformably overlie Precambrian Gondwanan continental crustal rocks (Miller, Reference Miller1983; Ranger et al. Reference Rotondo and Bentley1984).
The muddy OCC is located on N-NW Bell Island (‘Locality I’ of Ranger et al. Reference Rotondo and Bentley1984) and is preserved along 60 m tall cliffs, which fully expose dipping clinoform surfaces that were identified in this study as foreset, topsets and possible bottomsets (Fig. 3). At the base of the exposure, the uppermost Bell Island Group exposes oolitic, chamositic ironstone, onto which the Wabana Group strata downlap (Ranger et al. Reference Rotondo and Bentley1984) (Figs 2, 3). As a whole, the lowermost Power Steps Formation is composed of interbedded, clay-rich, silt-bearing mudstones and subordinate, decimetre-thick sandstones (Fig. 3). The lowermost part of the Power Steps Formation (Youngster’s Gulch Member) is composed of clay- and silt-rich mudstone that is interbedded with centimetre- to decimetre-thick medium- to coarse-grained sulfide-rich sandstone (Ranger et al. Reference Rotondo and Bentley1984) (Fig. 3). The formation has previously been interpreted as being deposited in a tidally dominated deltaic environment (Ranger et al. Reference Rotondo and Bentley1984), with detrital components sourced from the Precambrian Harbour Main, Conception and Cabot groups, as well as from the Holyrood plutonic series (e.g. Bruckner, Reference Bruckner1969).

Fig. 3. (a) Field view of the clinothem exposure (Youngster’s Gulch Member and Power Steps Formation) at Ochre Cove, Bell Island, Newfoundland. (b) Annotated view of the clinothem exposure indicating the architectural elements and major facies associations.
3. Materials and methods
3.a. Characterization of sedimentary fabric and texture
In order to examine the sedimentary processes responsible for the deposition of the OCC, the succession was logged at centimetre scale from continuous section (Fig. 2). Large (up to 50 cm in diameter), oriented hand samples (n = 35) of unweathered mudstone and sandstone were collected from the cliff face, stabilized with epoxy resin and slabbed in the laboratory. Petrographic thin-sections (thickness ∼25 μm) were prepared from select slabbed intervals and scanned at high resolution using a flatbed film scanner. Descriptions of texture, fabric, bedding contacts and bioturbation indices (sensu Taylor & Goldring, Reference Taylor and Goldring1993) from outcrop, hand specimen and petrographic thin-sections were integrated in order to generate facies descriptions that also reveal the most likely origin of framework components (grain versus cement). Mudstones were described using the methodologies of Campbell (Reference Campbell1967) and Lazar et al. (Reference Lazar, Bohacs, Macquaker, Schieber and Demko2015), in order to constrain both depositional environment and sediment transport mechanisms. Nine genetically related sandstone and mudstone facies have been grouped based on their palaeoenvironmental context into two facies associations (FAs).
3.b. Compositional analysis
Quantitative X-ray diffraction (QXRD) has been performed on 13 powder samples, which cover all sedimentologic facies. The pronounced stratigraphic trends for the most common minerals illite, chlorite and quartz have been captured. For QXRD analyses, ∼2 g of crushed material was processed in ethanol and micronized in a McCrone mill for ∼3 min. Samples were then dried at 60 °C and disaggregated using a mortar and pestle. Randomly oriented sample material was loaded into aluminium sample holders following the methods of Poppe et al. (Reference Poppe, Paskevich, Hathaway and Blackwood2001). For clay mineral analysis, the size fraction equivalent to <2 μm spherical diameter of quartz was separated by dispersing a representative sample of whole rock powder in a 0.1 % sodium phosphate solution and extracting particles from the upper 5 cm of suspension after settling for 3 hours 10 minutes as determined by Stokes’ law. Oriented clay smears were prepared following the methods of Moore & Reynolds (Reference Moore and Reynolds1997).
X-ray diffraction (XRD) data were collected using a Panalytical Empyrean X-ray diffractometer with Cu Kα radiation (40 kV and 20 mA). For clay mineral analysis, XRD runs were performed for each sample after each of the following four steps: (1) air-drying; (2) ethylene glycol salvation at 25 °C for a minimum of 8 hours; (3) heating at 300 °C for 1 hour; and (4) heating at 550 °C for 1 hour. Clay minerals were identified by comparing peak positions and intensity of basal (001) reflections on the four XRD diagrams (Moore & Reynolds, Reference Moore and Reynolds1997; Poppe et al. Reference Poppe, Paskevich, Hathaway and Blackwood2001). Semi-quantitative determination of bulk and clay mineral composition was facilitated by using MacDiff software (Petschick, Reference Petschick2001), with the uncertainty in XRD analysis estimated at ±5 %. For clay, mineral abundances are based on peak areas and normalized to Smectite + Illite + Kaolinite + Chlorite = 100 %. Grain boundaries and intergranular pore space filling have been visually examined.
3.c. Scanning electron microscopy (SEM)
Composition and sedimentary fabrics of representative samples from each facies were undertaken from polished thin sections using a JEOL JSM-7600F Thermal Field Emission Scanning Electron Microscope (SEM) equipped with an Oxford Instruments Aztec Energy Dispersive X-ray System and a silicon drift detector. Images were obtained primarily in backscattered mode utilizing a JEOL solid-state backscatter detector. Imaging conditions were 15 kV and 8 mm working distance. Polished thin-sections were prepared for SEM imaging by lightly cleaning the thin-section surface with methanol using low-lint cloths, air-dried and then coated with c. 30 nm of carbon using a Cressington 208 carbon evaporator. Particular attention was paid to the amount and type of grain dissolution, cementation as well as replacement, as an indicator for in situ weathering and generation of clay minerals, chlorite, and quartz cement (Figs 4–8; see discussion).

Fig. 4. Facies S1 and M1. (a) Outcrop photograph showing decimetre-thick S1 sandstone beds interbedding with decimetre- to metre-thick M1 mudstone. (b) Polished hand sample of fine- to medium-grained sandstone consisting of asymmetric (possibly combined-flow) ripples (black arrows) overlain by possibly combined-flow ripples with long wave periods (grey arrow). S1 sandstones internally exhibit erosional contacts (white dashed line). The interbedded M1 mudstone contains sandstone-filled gutters (white arrows) and sparse, millimetre-sized sand-filled Planolites burrows (P). (c) Close-up micrograph of M1 mudstone (perpendicular to bedding, flat bed scan). M1 mudstones are faintly laminated with elongated silty streaks and burrow fills of medium-grained chloritized grains and lithoclasts. (d) Low-resolution micrograph of M1 with dominant replacement mineralogy (plane-polarized light, perpendicular to bedding). Note how Fe-enriched chlorite with well-developed replacement fabric (white, dashed polygons, Fe–Chl) and in authigenically replaced lithic fragments (LF) dominate a large portion of the M1 mudstone. ‘Floating’ quartz grains (Qz) and remnants of Felspar (Fsp) are also present.
4. Facies and facies associations
4.a. Facies descriptions
Nine sedimentary facies, four sandstone facies and five mudstone facies were recognized in this study. Sedimentary facies were identified by integrating hand specimen observations, thin-section petrography, and QXRD analyses. Sandstone and mudstone facies are described separately. The stratigraphic distribution of bioturbation style and intensity as well as the main mineral trends in the mudstones follow the description of individual facies.
4.b. Facies S1: amalgamated sandstone
4.b.1 Description
Facies S1 comprises less than 10 % of the studied section. This facies is characterized by fine- to medium-grained, continuous sandstone units, with internally discontinuous, often amalgamated erosive-based, centimetre- to decimetre-thick, thin-bedded, beds and bedsets (Fig. 4a). These internal beds and bedsets contain steep (>10°) bidirectional ripples with broad scours that are overlain by long-wavelength wave ripples with upward-flattening lamina sets (Fig. 4b). S1 sandstones are sparsely bioturbated (BI 1–2, 5–30 % sensu Taylor & Goldring, 1993). The most common trace fossils observed include compacted Planolites (Fig. 4c). The S1 framework is composed of predominantly detrital, well-cemented quartz embedded within a sand-sized pseudomatrix of diagenetically altered lithic fragments. Minor components include detrital biotite and muscovite, with remnants of plagioclase and heavy accessory minerals (Fig. 4d).
4.b.2. Interpretation
Relatively continuous S1 sandstone beds (>10 m continuity) (Fig. 4a) with a vertical division of erosive-based wave ripples with increasing wavelength are interpreted as having been deposited by possibly combined flows with relatively long wavelengths (Clifton, Reference Clifton, Posamentier and Walker2007). Well-preserved bidirectional wave ripples with long wavelengths are indicative of sediment reworking and lateral transport under the influence of wave action; wave-ripple erosion might also indicate a (weak) unidirectional component operating during deposition (Duke et al. Reference Duke, Arnott and Cheel1991; Myrow & Southard, Reference Myrow and Southard1996) (Fig. 6b, further below).
The absence of significant volumes of detritally derived, clay-rich material within S1 sandstone may imply deposition under initially very low clay concentration (little to no interstratified mud) or an initially fast aggradation of sand-sized material. The presence of wave-reworked tops and significant breaks in sedimentation without change in grain size argues for occasional erosion and possibly redeposition (Fig. 4b). A thin top layer with mainly fine-grained sandstone-filled Planolites within this coarser-grained S1 sandstone and some other biodeformational structures indicates potential bypass of the finer grain-size fraction downstream (Fig. 4b).
4.c. Facies S2: lenticular sandstone
4.c.1. Description
Lenticular sandstone makes up less than 5 % of the studied section. This facies appears as centimetre-thick, erosive-based, laterally discontinuous, very fine-grained sandstones that are interbedded with wave-reworked mudstone of facies M2 (Fig. 5a–d). S2 sandstone contains current or possible combined-flow ripples (Fig. 5b). S2 sandstones appear generally non-bioturbated. Framework grains include predominantly detrital quartz, diagenetic chlorite cement, lithic clasts and detrital biotite together with some muscovite.

Fig. 5. Facies S2 and M2. (a) Outcrop view of mud-dominated facies M2, showing curved bed contacts and possible mud-on-mud erosional contacts (white dashed lines). (b) Polished hand sample of M2 mudstone, showing starved, asymmetric wave (possibly combined-flow) ripples (white arrows) of facies S2. Continuous, centimetre-wide erosional mud-on-mud contacts (white dashed line) are common in this facies. (c) Close-up micrograph showing centimetre-thick event beds (sediment gravity flows, SGFs) with a cross-laminated base (unit a), overlain by a laminated silty middle portion (unit b) and a bioturbated silt-rich mudstone (unit c). Clay-rich tops contain occasionally Gyrolithes (G), Planolites (P) and Trichophycus (T). (d) High-power SEM micrograph (backscatter mode) shows silt-sized, authigenically replaced mafic lithoclasts (LF, black arrow), engulfed within a ‘pseudomatrix’ of Fe-rich chlorite cement (white arrow) that also include ‘floating’ quartz (Qz) grains.
4.c.2. Interpretation
The highly variable distribution of this facies indicates that supply of sand has not been continuous throughout the deposition of this facies. Internally curved, non-even lamination (Fig. 5b) indicates that ripples have not been deposited entirely via current-dominated processes, but transport also might have included an oscillatory component. Lamina geometries resembling wave-reworking could point to the fact that these sandstone patches were differentially compacted starved ripples, similar to the ones described by Schieber et al. (Reference Schieber, Southard and Schimmelmann2010). While during an initial erosion event (i.e. storm) a previous sand bed was reworked and mostly removed, the ripple trails indicate the lateral transport during the waning phase of the storm, which also entails preferential preservation within gutters and other local topographic depressions distributed over the seabed. If this scenario is correct, then the thin, disconnected sandstone lenses and laminae represent ripple tails (Schieber et al. Reference Schieber, Southard and Schimmelmann2010).
4.d. Facies S3: storm-reworked sandstone
4.d.1. Description
Facies S3 makes up c. 20 % of the overall exposure at Ochre Cove. The S3 sandstones comprise thin-bedded (5–10 cm thick), fine-grained sandstone with laterally (at metre-scale) continuous units that exhibit wavy and even contacts (Fig. 6a, b). Internally, S3 sandstones exhibit laterally continuous sets of centimetre-sized dunes with partially eroded tops (Fig. 6a) Single dunes include well-developed sets of dark-coloured laminae (Fig. 6a) and, occasionally, millimetre-thick backflow ripples (Fig. 6b). Framework components comprise quartz within a pseudomatrix of chloritized lithic fragments. Minor components include biotite, muscovite and plagioclase. Some framboidal pyrite is present as well. S3 sandstones are non-bioturbated to sparsely bioturbated (BI 0–1, 0–5 %) and contain rare, vertical, decimetre-long, possible escape traces (Esc, Fig. 6a).

Fig. 6. Facies S3 and M3. (a) Close-up photograph of a large, polished hand sample resolving S3 sandstones and interbedded M3 mudstone. Medium-grained sandstone-filled gutters (white arrow) are overlain by pinch-and-swell lamination (small hummocky cross-stratified (HCS) beds?). Clay-rich bed tops contain a palimpsest ichnofaunal assemblage composed of Planolites (P) and Rosselia (Ro). Laterally continuous centimetre-thick, partially eroded, potential three-dimensional tidally influenced dune-scale bedforms (TD, grey arrows) comprise facies S3. Those tidal dunes have eroded tops (black dashed line) and include prominent tidal bundling (white, opposing arrows). (b) Cross-section through facies M3 mudstone showing the tripartite subdivision of partially eroded, stacked, potential HCS beds: cross-laminated, very fine-grained basal sandstone (unit I) with intensely bioturbated middle portion (unit II) is overlain by a non-bioturbated clay-rich mudstone (unit III). If preserved, the upper bed portion appears to be modified via cryptobioturbation (CB, white arrows) the basal portion of sandstone S3 (tidal dunes), with well-developed current ripple lamination (black, continuous line) with tidal reactivation surfaces (black, thick continuous lines; BR). (c) Backscattered SEM micrograph of the fine-grained fraction in facies M3 showing silt-sized mica (Biotite, ‘Bio’) and quartz grains (Qz), embedded within a silt- and clay-sized illite-rich matrix (black arrows).
4.d.2. Interpretation
The unidirectional ripple sets with backflow ripples possibly represent reactivation surfaces formed during current reversal. Given that S3 sandstones are preferentially located in near-shore facies (delta top in Figs 2, 3), a combination between fluvial and tidal influence is plausible. Frequently eroded tidal dune beds are sharply overlain by M3 mudstones (Fig. 6a). The tidal influence inferred for S3 sandstones is supported by the fact that the entire unit is non-bioturbated. Permanently changing salinities with episodically brackish conditions could have put additional stress on any infauna and therefore prevented colonization. Another possibility is that bioturbated tops were eroded during storm events.
4.e. Facies S4: planar-laminated sandstone
4.e.1. Description
This facies covers c. 5 % of the entire succession (Fig. 2). S4 sandstones appear as decimetre-thick, erosive-based, laterally highly continuous beds with non-even contacts (Fig. 7a). Individual units contain planar-bedded, normally graded fine- to medium-grained sandstone with muddy tops that belong to mudstone facies M4 (Fig. 7b, c). The tops of individual, erosive-based S4 event beds contain elongated, well-cemented septarian horizons and septarian concretions composed of brownish/reddish (possibly) ferroan carbonate (Fig. 7b). In hand specimen, S4 facies appear non-bioturbated. The framework is composed of angular quartz, lithic clasts, biotite and some minor plagioclase and muscovite.

Fig. 7. Facies S4 and M4. (a) Outcrop panel showing the OCC topset and foreset. White arrows indicate positions of large septarian concretions, which seem to occur preferentially along bed contacts. A normal fault (white, continuous line) dissects the OCC. (b) Close-up of facies S4; graded bedding of medium- to coarse-grained sandstone S4. Note that large septarian concretions and lenses are concentrated at bed tops (white arrow). (c) Flat bed scan of M4 mudstone, which is faintly laminated (white, dashed lines), with ‘floating’ silt grains throughout the clay-rich matrix that also contain rare biodeformation structures (Bio). (d) Backscattered SEM micrograph of the fine-grained fraction in facies M4 showing silt-sized mica (Biotite, ‘Bio’), feldspar (Fsp) and quartz grains (Qz) with overgrowths (white arrows), embedded in a pore-occluding illite-rich matrix (black arrows) and white mica (Musc).
4.e.2. Interpretation
The presence of erosive-based, laterally highly continuous, graded event beds with little evidence for post-depositional reworking indicates deposition from a depletive flow, such as a turbidity current (e.g. Talling et al. Reference Talling, Masson, Sumner and Malgesini2012). The vertical bed thickness of several decimetres and its stacked nature in conjunction with laterally continuous beds that can be traced across the entire OCC exposure (Fig. 7a) indicate deposition from depletive currents across large parts of the clinothem.
4.f. Facies M1: thick, continuous mudstone
4.f.1. Description
This facies was only observed in the lower part of the OCC and makes up less than 10 % of the overall succession (Fig. 2). M1 mudstone facies are developed as decimetre-thick, continuous mudstone beds with wavy bed contacts that also encase subordinate, centimetre-wide, sandstone-filled gutters (Fig. 4a, b). The bioturbation intensity is sparse to moderate (BI 1–2; 5–30 %) and consists of predominantly compacted, sandstone-filled, centimetre-sized Planolites burrows (Fig. 4c). Bed tops are moderately bioturbated by millimetre-sized Planolites and biodeformational structures (Fig. 4c). The framework of facies M1 contains very fine-grained to coarse silt-sized angular and subrounded quartz floating in a matrix of silt-sized, partially chloritized lithic fragments and mafic minerals, together with chlorite and illite that make up the matrix of this mudstone (Fig. 4d). Minor M1 components encompass biotite, muscovite and very little plagioclase (Fig. 4d).
4.f.2. Interpretation
Decimetre-thick, continuous mudstone beds intially encompassed a wide grain-size distribution that initially contained a large volume of silt, very fine-grained sized, potentially highly weathering susceptible minerals and (volcanoclastic) rock fragments (Fig. 4d). This mineral assemblage indicates deposition under rapidly decelerating flows, possibly from submarine flows with an initially high volcaniclastic content (pyroclastic currents?). Coarse-grained mud with high volumes of weathering-susceptible grains could also have been delivered from suspension settling of dense volcaniclastic clouds, after volcanic eruptions in the hinterland or after settling from plumes that eroded ash from a non-vegetated hinterland (Harazim & McIlroy, Reference Harazim and McIlroy2015). At this point it cannot be determined if the absence of grading within M1 mudstones reflects primary sedimentation, or if this represents a weathering artefact.
4.g. Facies M2: wave-reworked mudstone
4.g.1. Description
Facies M2 makes up the central part of the exposed OCC (less than 10 % of the entire exposure) (Fig. 5). Decimetre-thick M2 mudstone beds are highly discontinuous with even and wavy contacts and exhibit, internally, either one or two grain-size breaks (Fig. 5a, b). At bed scale, M2 mudstone contains subparallel, discontinuous, normally-graded medium silt- and clay-rich laminae that often preserve a prominent triplet motif (Fig. 5c, motif A–C). Mud-on-mud erosion is common in this facies. M2 mudstones are non- to sparsely bioturbated (BI 0–2; 0–30 %) comprising sandstone-filled Planolites and rare Gyrolites, preferentially confined to bed tops (Fig. 5c). Internally, M2 mudstone is composed of subangular to rounded, fine to medium silt-sized quartz, lithic clasts, biotite, some plagioclase and muscovite. The M2 matrix is predominantly composed of chlorite which shows intense replacement fabric within and around lithoclasts (Fig. 5d).
4.g.2. Interpretation
In M2 mudstones, well-preserved, highly continuous, erosion surfaces cross-cut partially preserved centimetre-thick clay- and siltstone-rich mudstone beds. This type of partial mudstone bed preservation is commonly the product of slow accumulation and periodic storm-wave reworking (Harazim & McIlroy, Reference Harazim and McIlroy2015). The preservation of centimetre-sized, partly erosive starved ripples with asymmetric lamination (facies S4) indicates that mud delivery to the seafloor is pulsed, alternating with periods of lateral sand transport but without significant aggradation (Schieber & Yawar, Reference Schieber and Yawar2009). The intercalated, thin (millimetre-thick) beds with highly discontinuous lamina sets indicate periods of mud deposition that were most likely dominated by oscillating or even combined flows, possibly above the fair-weather wave base (Denommee et al. Reference Denommee, Bentley, Harazim and Macquaker2016) (Fig. 5c).
4.h. Facies M3: normally-graded mudstone
4.h.1. Description
Facies M3 makes up c. 25 % of the logged section at Ochre Cove (Fig. 8). M3 mudstones are developed as centimetre-thick, wavy-discontinuous very fine-grained siltstone and silty mudstone separated by one or, sometimes, two grain-size breaks (Figs 6a,b). The bases of single beds are subdivided into three parts. Fine-grained basal units (unit I) contain planar and cross-laminated siltstone with some pinch-and-swell lamination that occasionally also includes some very fine-grained sandstone (Fig. 6b). This latter unit is overlain by wavy laminated silty mudstone (unit II) and non- to sparsely bioturbated clay-rich mudstone (unit III) (Fig. 6b). The M3 bioturbation index is 0–1 (0–5 %). Bed tops contain rare compacted, sandstone-filled Planolites, very rare Rosselia and some biodeformation (Fig. 6a). Discrete lamina sets and bed boundaries appear disrupted and ‘diffuse’ (Fig. 6b). The framework of the mudstone-dominated unit III (Fig. 6c) facies is composed of subangular to rounded, fine- to medium-grained quartz, silt-sized lithic clasts, biotite, some plagioclase and muscovite. The matrix is composed predominantly of illite (Fig. 6c).
4.h.2. Interpretation
Preferential preservation of single centimetre-sized gutters filled with coarser-grained sandstone, in conjunction with well-developed grain-size breaks within mudstone (Fig. 6b), possibly indicates erosion of sand and bypass following gravity flow deposition. Preferential preservation of sandstone in open Planolites burrows highlights that initial coarser-grained bed tops (which infilled open Planolites burrows) might have been eroded (Fig. 6a). Diffuse laminae potentially indicate the presence of cryptobioturbation, attesting to the possibility of oxygenated pore waters following deposition (Gingras et al. Reference Gingras, MacEachern and Dashtgard2011). Two prominent grain-size breaks between the basal very fine-grained sandstone (unit I), faintly laminated coarse siltstone (unit II) and the overlying sparsely to non-bioturbated mudstone (unit III) potentially support flow transformation during bed deposition, respectively during the waning phase of the sediment flow (Duke et al. Reference Duke, Arnott and Cheel1991).
4.i. Facies M4: homogeneous mudstone
4.i.1. Description
The M4 mudstones comprise c. 10 % of the entire succession and are developed as centimetre- to decimetre-thick, highly continuous beds over several tens of metres, bound by erosive, wavy-continuous contacts (Fig. 7a). These mudstones are composed of silt-bearing, clay-rich mudstone that terminates with decimetre-thick, metre-wide carbonate lenses and decimetre-wide septarian concretions (Fig. 7a, b). Internally, M4 mudstones appear homogeneous and contain little evidence of bioturbation (BI 0–1; 0–5 %). Only very rarely isolated patches of biodeformational and escape structures are visible in bed tops (Fig. 7c). The framework components include subangular to round, fine to medium silt-sized quartz, lithic clasts, biotite, some plagioclase and muscovite. The mudstone M4 matrix is composed predominantly of illite (Fig. 7c, d). The septarian concretions are composed of potentially ferroan carbonate.
4.i.2. Interpretation
The continuous nature of the M4 mudstone, in combination with a non-graded texture and the fact that it lies conformably above S4 sandstone, indicates that M4 mudstones could have been deposited by rapidly decelerating flows. These deposits are commonly observed on the clinothem foreset, and might indicate the final stage of deposition from potentially dilute density currents (Talling et al. Reference Talling, Masson, Sumner and Malgesini2012; Zavala & Arcuri, Reference Zavala and Arcuri2016). Longer periods of slower sediment accumulation produce excellent conditions for the growth of carbonate cement and the formation of ferroan carbonate lenses and septarian concretions (Föllmi, Reference Föllmi2016) (Fig. 7b). Their stratigraphic relationship suggests that M4 mudstones are genetically related to underlying S4 sandstones. If those S4 sandstones originated from offshore-directed sediment gravity flows then the decimetre-thick, non-graded, upper M4 portion could originate from lofting and coeval suspension settling (Zavala & Pan, Reference Zavala and Pan2018).
4.j. Facies M5: thin-bedded mudstone
4.j.1. Description
Thin-bedded mudstone comprises c. 20 % of the OCC (Fig. 2). M5 mudstone contains centimetre-thick, even and wavy-continuous beds with small, centimetre-sized sandstone-filled gutters (Fig. 8a, b). The M5 mudstone beds are normally graded with both even and cross-laminated coarse siltstone in the base and clay-rich mudstone. Beds are laterally continuous at the decimetre scale (Fig. 8a). Within millimetre-thick beds, siltier laminae form upward deformed flame structures (Fig. 8b). This facies is non-bioturbated to sparsely bioturbated (BI 0–1; 0–5 %) and contains millimetre-sized, sand-filled Planolites, Trichophycus and ample biodeformational structures preferentially concentrated close to bed tops. The framework components contain subangular to rounded, fine to medium silt-sized quartz, lithic clasts, biotite, and some plagioclase and muscovite (Fig. 8c). The mudstone matrix is composed predominantly of illite and some dark, wavy stringers of kerogen (Fig. 8c, d).

Fig. 8. Facies M5 (thin-bedded mudstone). (a) Polished hand sample showing centimetre-thick, wavy-continuous, normally-graded beds, many of which contain erosive tops (white dashed lines) and are sparsely bioturbated by shallow-tier Trichophycus (T). Centimetre-wide sandstone-filled gutters are occasionally preserved (white arrows). (b) Stacked, partially eroded beds contain coarse siltstone in the base, overlain by fine silt- and clay-rich mudstone. Soft-sediment deformation below these event beds (black arrows) is common. Sandstone-filled gutters are common at bed tops (white arrows). (c) Low-resolution micrograph showing the textural characteristics of a single, normally graded M5 bed. Wavy stringers of kerogen and possibly pyrite (white arrows) are dispersed throughout the mudstone (plane-polarized light). (d) Backscattered SEM micrograph of the fine-grained fraction in facies M5 showing silt-sized platy mica (biotite, ‘Bio’), some silt-sized quartz (Qz), embedded within a pore-occluding illite-rich matrix (white arrows).
4.j.2. Interpretation
Thin-bedded, laterally continuous mudstone beds with normal grading and occasional flame structures point towards relatively rapid deposition over an unconsolidated, preferentially mud-rich seabed (Fig. 8b). The clay-rich, non-bioturbated tops could originate from rapid deposition and burial. Thinner and finer-grained M2 beds were deposited below the fair-weather wave base, where only strong storms or density flows were able to rework the seafloor. Gutters filled with fine-grained sandstone with wavy-discontinuous lamination indicate occasional bed erosion via storms and bypass of coarser-grained sediment (Fig. 8a). The presence of flame structures indicates lateral drag in mud that was not yet fully dewatered when the following event bed has been deposited (e.g. Mills, Reference Mills1983) (Fig. 8b). The dark stringers composed of both, pyrite and wavy opaque material might constitute allochthonous (or reworked?) kerogen (Harazim & McIlroy, Reference Harazim and McIlroy2015) (Fig. 8c, d).
4.k. Facies associations
4.k.1. FA1: wave- and current-reworked topsets
Sandstone facies S1 and S2, and mudstone facies M1–M3 share basal flat lamination and were therefore grouped into one facies association. In this, wave-ripple cross-lamination, trough cross-bedding and undulating lower bounding surfaces are indicative of bedform migration and potentially widespread seafloor erosion and bed removal (Figs 4–6). Elongate sandstone-filled scour structures (i.e. gutter casts) with ripple cross-lamination that are dipping transverse to scour axes are prominent for facies S3. Likewise, the disconnected, asymmetric, starved sandstone ripples (Fig. 5) equivalent to the same S3 facies could represent a part of the topset that is not directly influenced by frequent riverine sand supply (Catuneanu & Zecchin, Reference Catuneanu and Zecchin2013). Well-developed, unidirectional wave-reworked sandstone (S1), medium-grained, wave- and current-rippled sandstone (S2) interbedded with M1 and M2 all exhibit a high lateral continuity, similar (decimetre) thickness and were observed in facies that most likely belong to the clinoform topset (Fig. 2, Fig. 11 further below). Mud-rich facies M1, M2 and M3, located below 13 m stratigraphic height (Fig. 2), were grouped into this FA1 facies association due to their significantly higher amount of Fe-rich chlorite, compared to FA2 (Fig. 9).

Fig. 9. The bulk and clay mineralogical composition of the studied interval at Ochre Cove. Note the overall increase in the ratio of clay minerals to quartz and the shift in the dominant clay mineral from chlorite to illite, which were recorded across ∼10 m stratigraphic height.
4.k.2. Facies association 2 (FA2): gravity-flow dominated foresets and bottomsets
Planar-laminated sandstone (S4), homogeneous mudstone (M4) and thin-bedded mudstone (M5) exhibit high lateral continuity at the bedset scale and were almost exclusively observed on the clinothem bottom- and foreset, at above 20 m stratigraphic height (Fig. 2). Above 20 m, those latter mudstones also experience a substantial increase in illite/chlorite ratio. FA2 sandstone and mudstone facies exhibit very high aspect ratios, with graded bedding and well-developed grain-size breaks that terminate, in the case of facies M4, with stratiform septarian concretions and lenses (Figs 8–10).
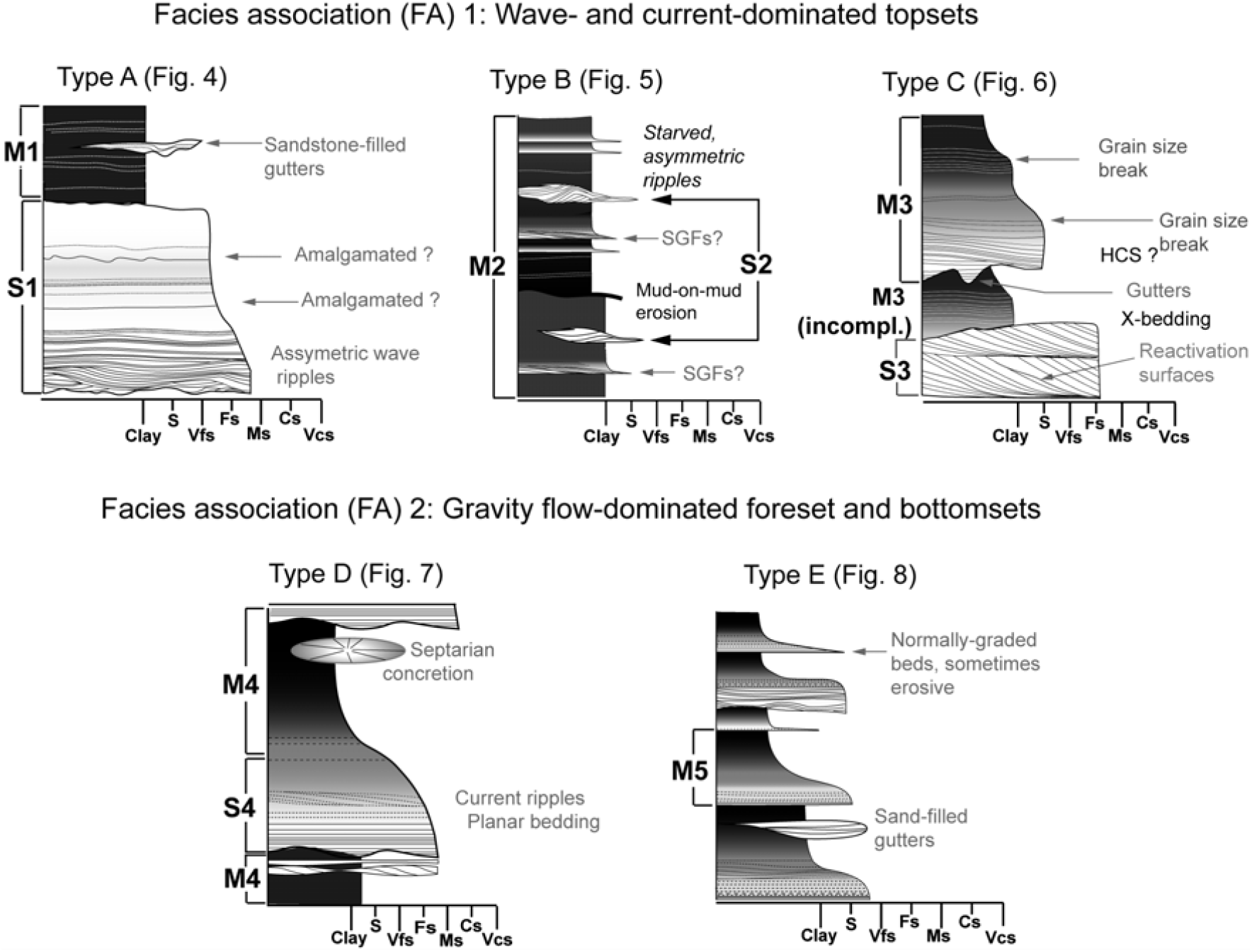
Fig. 10. The five characteristic, recurring bedset types A–E across the OCC depositional profile. Each bedset type contains at least one out of four sandstone (S1–4) and five mudstone (M1–5) facies. Bedset types and facies are summarized into two facies associations (FAs): FA1 (wave- and current-dominated topset) and FA2 (gravity-flow dominated foreset and bottomset).
5. Stratigraphic distribution of component minerals in the mud-dominated facies
About seven dominant minerals were identified by employing SEM petrography and whole-rock and clay-specific QXRD, of which only four were abundant enough (>5 wt %) to be relevant for discussion in clinothem building and progradation (Fig. 9).
Stratigraphic comparison of QXRD analyses (Fig. 9) shows significant mineralogical variability throughout the studied succession. The volume of clay minerals as a percentage of the total rock volume increases vertically through the succession. Non-clay silicate minerals (i.e. quartz and plagioclase), as a percentage of total rock volume, however, show variations decreasing from >50 % by volume at the base of the section to ∼30–40 % at the top. A notable deviation from this trend is observed at ∼9 m (Fig. 9), where non-clay silicates contribute to only ∼14 % of the rock volume. Overall the ratio of clay minerals to non-clay silicates in the succession increases vertically. The composition of the clay minerals also varies within the succession. Chlorite, as a percentage of the clay mineral volume, sharply decreases throughout the succession from >90 % of the clay mineral assemblage at the base of the studied succession to a low of 43 % at 12.5 m stratigraphic height (Fig. 9), above which the percentage of chlorite remains relatively constant at ∼55 % of the clay mineral volume. Illite as a percentage of the clay mineral volume increases throughout the succession from <10 % at the base of the studied section to a maximum of 57 % at 12.5 m stratigraphic height, above which the percentage of illite remains relatively constant at ∼45 %. Pyrite is observed as an accessory component, comprising <5 % of the total rock volume except for the lower 10 m of the exposure, where its higher volume coincides with the presence of pyritic sandstones described by Ranger et al. (Reference Rotondo and Bentley1984).
6. Discussion
6.a. Process-based interpretation of event bed deposits
Bedset types are useful descriptors of mud depositional processes, because they preserve the mudstone depositional product as it evolves throughout successive stages of bed formation (erosion → settling → deposition → reworking), which then can result in the preservation of more than one stacked facies (Fig. 10). The fabric and texture of individual sandstone and mudstone beds depend on the initial sediment concentration and especially volume fraction of cohesive clay (Sumner et al. Reference Sumner, Talling and Amy2009). A change in flow viscosity and yield strength across orders of magnitude can be induced by adding even small amounts of clay to the suspension (e.g. Coussot, Reference Coussot2017), which, overall, controls if bedforms develop (Schieber & Southard, Reference Schieber and Southard2009). Five recurring mudstone bedset types (A–E) record sand and mud transport and depositional processes across the OCC depositional profile. Those bedset types are defined based upon mudstone grading, fabric and characteristics of subordinate sandstone beds and lenses, and are internally composed of at least two but often more than two lithofacies. This process-based interpretation of OCC mudstones is based upon an assemblage of well-described ancient sand- and mudstone deposits (Duke et al. Reference Duke, Arnott and Cheel1991; Myrow & Southard, Reference Myrow and Southard1996; Clifton, Reference Clifton, Posamentier and Walker2007; Haughton et al. Reference Haughton, Davis, McCaffrey and Barker2009; Macquaker et al. Reference Macquaker, Bentley and Bohacs2010; Talling et al. Reference Talling, Masson, Sumner and Malgesini2012; Plint, Reference Plint2014; Poyatos-Moré et al. Reference Poyatos-Moré, Jones, Brunt, Hodgson, Wild and Flint2016; Birgenheier et al. Reference Birgenheier, Horton, McCauley, Johnson and Kennedy2017), experimental studies (Baas et al. Reference Baas, Best and Peakall2011, Reference Baas, Davies and Malarkey2013, Reference Baas, Best and Peakall2016) and examples from the modern seafloor (Bentley & Nittrouer, Reference Bentley and Nittrouer2003; Rotondo & Bentley, Reference Rotondo and Bentley2003; Denommee et al. Reference Denommee, Bentley, Harazim and Macquaker2016).
6.b. Deposition and reworking of mud in the OCC
Variability in lithofacies across the OCC is driven by (a) prominent change in bedding aspect ratio, (b) winnowing of grain size in more distal portions of the clinothem and (c) a gradual change from wave- to gravity-controlled deposition control (Fig. 11). This depositional setting produces bedsets composed of often multiple, stacked event beds with a wide range of grain sizes, which do not always show traditional (offshore-fining) facies distributions with increasing shoreline proximity. Normally-graded beds with erosive tops and palimpsest ichnofabric are formed on modern storm-dominated shelves, where large volumes of coarse-grained sediment are seasonally discharged via flashfloods and reworked across high-energy shelves (e.g. Eel Shelf; Milliman & Syvitski Reference Milliman and Syvitski1992; Ogston et al. Reference Ogston, Cacchione, Sternberg and Kineke2000).

Fig. 11. Schematic block diagram showing the facies diversity, along with the distribution of bedset types across the OCC depositional profile.
FA1 deposits are inferred to originate from episodic, storm-driven event sedimentation. Storm conditions likely existed only for short, discrete periods within the clinothem topset depositional environments. Their influence on sediment transport and deposition, however, is disproportionately large because they are erosional and deposit thick beds (Clifton, Reference Clifton, Posamentier and Walker2007). When storms coincide with high fluvial discharge then large quantities of fine-grained sediment are discharged onto the shelf via deltas (Hill et al. Reference Hill, Voulgaris and Trowbridge2001; Mulder et al. Reference Mulder, Syvitski, Migeon, Faugeres and Savoye2003). Storm-related discharge delivers a large amount of sand during heightened seasonal discharge (Zavala & Arcuri, Reference Zavala and Arcuri2016). This discharged, water-laden mixture of sand, water and mud forms bottom-hugging sediment laden suspensions that alternate between deposition and erosion, and can therby deposit complex composite beds (Zavala & Arcuri, Reference Zavala and Arcuri2016; Zavala & Pan, Reference Zavala and Pan2018).
Another possibility for the deposition of FA1 units is a potential slope failure. The resulting avalanching generates fully turbulent gravity flows (i.e. turbidity currents) with potentially long flow run-out distances (e.g. Haughton et al. Reference Haughton, Davis, McCaffrey and Barker2009). Both sandstones and mudstones of FA1 were deposited by both unidirectional currents (Fig. 5) and waves (Fig. 7). In the sandstone fraction, centimetre- to decimetre-thick Type A bedsets preserve decimetre-length wavelength wave ripples, intra-bed erosional surfaces and very sharp grain-size changes (Fig. 10A). M1 mudstones contain internally little fabric evidence for bedload transport (i.e. neither widespread lamination, nor starved sandstone ripples or wavy-discontinuous lamination). Instead, M1 mudstones seem to record alternating pulsed sediment delivery events of sand and mud as well as frequent storm-dominated erosion and sediment bypass. The presence of sandstone-filled scours (Fig. 4b) represents a coarse-grained relict facies that could potentially indicate sediment bypass.
Starved fine- to medium-grained sandstone lenses (Fig. 5, Type B bedsets) with internally asymmetric wave ripples of S2 possibly originated during unidirectional transport and subsequent wave reworking (Duke et al. Reference Duke, Arnott and Cheel1991). Millimetre-thick beds with a prominent triplet motif are common throughout this facies (Fig. 5c). M2 beds broadly resemble bed stratigraphy deposited by a number of density-driven mud-rich gravity flow types similar to current-wave-enhanced sediment gravity flows (CWESGFs) (Macquaker et al. Reference Macquaker, Bentley and Bohacs2010; Denommee et al. Reference Denommee, Bentley, Harazim and Macquaker2016), hyperpycnal flows (e.g. Mulder et al. Reference Mulder, Syvitski, Migeon, Faugeres and Savoye2003; Lamb & Mohrig, Reference Lamb and Mohrig2009) and turbidity currents (e.g. Haughton et al. Reference Haughton, Davis, McCaffrey and Barker2009). Readily visible strataform, silty linings connecting starved sandstone ripples (Fig. 5b) were previously described as the product of migrating ripples that indicate forcing via unidirectional currents that prevailed at least during the waning phase of the flow (Schieber, Reference Schieber2016).
Repeated observance of intra-bed sedimentary fabrics within layers that contain this Type B bedset type that exhibit a triplet motif (unit a – turbulent traction transport and erosion; unit b – planar parallel laminae deposited during the laminar phase of flow; unit c – suspension settling as the flow wanes) (Fig. 5b) probably indicates a form of waning density flow that potentially experienced wave-influenced transport. However, true CWESGFs (sensu Friedrichs & Wright, Reference Friedrichs and Wright2004) require sufficient supply of detritally sourced fluidized muds to facilitate across-shelf mud transport (Wright et al. Reference Wright, Friedrichs, Kim and Scully2001; Bentley & Nittrouer, Reference Bentley and Nittrouer2003). This condition is unlikely to have been met in the Power Steps Formation, since the original illite-dominated detrital clay–mineral fraction sourced from the Palaeozoic hinterland is relatively small (Harazim & McIlroy, Reference Harazim and McIlroy2015). Nevertheless, they might constitute some form of (wave-modified?) sediment gravity flow (SGF) (Fig. 5c).
Sandstones of Type C bedsets contain laterally continuous, cross-bedded sandstone with internal duplet structures and ample reactivation surfaces (Fig. 6a). Based on their unidirectional ripples and abundant reactivation surfaces, these sandstones are being interpreted as the product of tidal dune deposition that were deposited and preserved between storm events. Here as well, well-developed grain-size breaks in overlying M3 facies indicate bypass of intermediate grain sizes, possibly as a result of increasing relative clay content during the waning phase of the storm (e.g. Coussot, Reference Coussot2017).
Sandstone-dominated tidal bedforms in facies S3 (Fig. 6a) and normally graded M3 mudstone are interpreted as the more distal equivalent of S1 sandstones and M1 mudstones. While storm-driven mud dispersal is inferred to have been episodic on the OCC, the presence of centimetre-thick, three-dimensional tidal bedforms indicates that the prevailing fair-weather sedimentation has been dominated by unidirectional tidally controlled sand transport. The absence of a fully developed hummocky cross-stratification in the base layer of M3 facies (Duke et al. Reference Duke, Arnott and Cheel1991) might result from the fact that original grain-size distribution also contained higher amounts of cohesive clay.
Either way, the occurrence of Type B bedsets indicates that at one point muds were being advected alongshore, outside the reach of immediate sand supply where the shore-oblique geostrophic currents component increases in strength (Duke et al. Reference Duke, Arnott and Cheel1991; Plint, Reference Plint2014). The presence of short, asymmetric very fine-grained sandstone ripples and frequent large-scale (mud-on-mud) erosion events (Fig. 5b) perhaps indicates that more distal Type C bedsets experienced a higher preservation potential and more complete preservation of event stratigraphy compared to Type A or B bedsets, even though bed reworking frequency and intensity is proposed to have been broadly similar (see Fig. 11).
It is inferred that the storm-weather wave base (which is usually one order of magnitude deeper than the regional fair-weather wave base; Snedden et al. Reference Snedden, Nummedal and Amos1988) has changed across the OCC. The preservation potential of shelf currents seems to increase from the proximal to the distal portion of the clinothem topset where the majority of mud and sand is being moved offshore via unidirectional, river-flood hyperpycnal discharge. This offshore transport has, additionally, been modified by a combination of combined flows (consisting of wave-motion and long-shore directed geostrophic flows; Perillo et al. Reference Perillo, Best and Garcia2014) and, to some degree, by a density-driven offshore flow (Duke et al. Reference Duke, Arnott and Cheel1991; Myrow & Southard, Reference Myrow and Southard1996).
At modern-day sea-level highstand (Skilbeck et al. Reference Skilbeck, Heap and Woodroffe2017) some shelf areas often remain too gentle to initiate autosuspensive, gravity-dominated transport (i.e. as a turbidity current). The initiation of hyperpycnal turbidity currents requires a gradient of at least 0.7°, a flooding event, and high suspended sediment concentration (e.g. Wright et al. Reference Wright, Friedrichs, Kim and Scully2001; Mulder et al. Reference Mulder, Syvitski, Migeon, Faugeres and Savoye2003), conditions which are easily met in the studied section. With a measured slope of ∼5° on the clinothem foreset (Fig. 6a), autosuspensive transport might easily have been initiated after pulsed flashfloods or earthquakes. The deposition of large volumes of initially non-weathered, sand-size grains and clasts, with a typical volcanic grain density of above 2.7 g cm–3, could easily have produced clinoform foreset slopes in excess of 0.7°.
The OCC foreset and bottomset (FA2) are interpreted to represent exclusively pulsed sediment delivery and much longer breaks in sedimentation compared to FA1. This is based on the presence of laterally continuous, normally-graded event bed deposits with extensively cemented and concretionary bypass surfaces (Fig. 7a, b). Even-bedded and ripple cross-laminated S4 sandstone of Type D bedsets are encountered close to the clinothem rollover, most likely originated as dilute, fully turbulent density flows with relatively low sediment fall-out rate (Fig. 7b) that either formed in an area of flow expansion (close to an increase in clinothem slope; Kane & Hodgson, Reference Kane and Hodgson2012; Poyatos-Moré et al. Reference Poyatos-Moré, Jones, Brunt, Hodgson, Wild and Flint2016) or as a result of river-fed hyperpycnal currents (Sumner et al. Reference Sumner, Talling and Amy2009). The prevalence of normal grading and absence of the preserved inversely graded lower bed portion within Type D bedsets indicate that those flows were potentially not always sustained (Bhattacharya & MacEachern, Reference Bhattacharya and MacEachern2009). The overall absence of interlaminated sand–mud current ripples throughout FA2 suggests that flow deceleration times were probably short.
Palimpsest bioturbation in facies M4 indicates either erosion of the bioturbated bed tops, or indicates rapid sediment accumulation on the clinothem with insufficient time available for bed colonization by benthic organisms. The OCC bottom-set Type E bedsets, which are interpreted to represent the thinner, distal deposits of turbidite flow run-outs, include unidirectional current-ripples, composed of fine-silt to fine-sand grains. These can be observed to downlap onto basal scour surfaces, whereby internal lamina sets display continuous, planar, fining-upward grading (Fig. 8a, b). Those beds have been preferentially recorded above the OCC downlap surface ‘DS’ (Figs 2, 3) that also includes the most distal clinothem bottomset deposits.
6.c. The origin of mud in the Power Steps Formation
Combined SEM and transmission light microscopy as well as QXRD analyses reveal a highly variable illite and chlorite volume across the OCC. The silt- and clay-sized mineral assemblage of the M1 and M2 facies is primarily composed of iron-rich minerals, which are in large part of diagenetic origin. The presence of widespread replacement fabric and large volume of chloritized grains and lithoclasts (Figs 4, 5), combined with the unusually high hand sample weight, due to high matrix Fe content, indicates that the clay- and silt-sized sediment fraction has originated, at least partially through the riverine influx of higher volumes of non-stable, Fe-rich mafic and mechanically weathered components that were most likely sourced from an early Palaeozoic, non-vegetated hinterland (Tosca et al. Reference Tosca, Johnston, Mushegian, Rothman, Summons and Knoll2010). Upon deposition, sand- and silt-sized mafic grains and lithoclast are chloritized within the sediment upon burial and temperature increase (e.g. Hower et al. Reference Hower, Eslinger, Hower and Perry1976; Harazim & McIlroy, Reference Harazim and McIlroy2015). This in situ transformation of less stable minerals appears to be a common process in the Bell Island Group, as has already been demonstrated in muddy shoreface deposits of the stratigraphically lower-positioned Beach Formation (Harazim & McIlroy, Reference Harazim and McIlroy2015). An initially silt-sized pseudomatrix, composed of weathering-susceptible olivine and pyroxenes, now altered to Fe-rich chlorite (chamositic) cement engulfs subangular silt-sized quartz, biotite, and muscovite and plagioclase (Figs 4d, 5d).
Burial-diagenetic dissolution of volcanic ash can lead to the precipitation of a wide range of authigenic minerals, controlled by their starting composition as well as weathering susceptibility (Kiipli et al. Reference Kiipli, Kiipli, Kallaste, Hints, Somelar and Kirsimäe2007). The large amount of illite recorded in the upper part of the OCC might have originated from the subsequent smectite-to-illite transition and subsequent quartz cement precipitation. Higher silica activities in initially open pore space of the silt-rich fraction could explain the high modern-day abundance of quartz overgrowths (Fig. 7d) and extensive pore-occluding illite (Figs 6–8) (e.g. van de Kamp, Reference Van de Kamp2008).
6.d. Clinothem architecture
Deltaic slopes in excess of ∼0.7° are not very common for modern-day transgressive shorelines (Boyd, Reference Boyd2010; Denommee et al. Reference Denommee, Bentley, Harazim and Macquaker2016). With a much steeper foreset the OCC does therefore provide a unique window into how mud has been deposited and converted into mudstone across an early Palaeozoic delta. Previous sedimentologic investigations of the underlying Tremadocian Beach (Harazim & McIlroy, Reference Harazim and McIlroy2015) and Redmans formations (Miller & McIlroy, Reference Miller, McIlroy and McIlroy2014) revealed that autocyclic changes in the supply of silt- and sand-sized detrital components dominate the stratigraphic and compositional variability of each respective depositional setting. On the OCC, outcrop-wide exposure of event beds allows correlation between all described bedset types and facies across the depositional profile, leading to the proposal of a sedimentological depositional dip-parallel model (Fig. 11). This model highlights that with increasing distance from the riverine dispersal system the relative thicknesses of storm-dominated mudstones (Type A and B bedsets) tend to become thinner, while bed continuity increases in the offshore direction (Type C bedsets). The Type D to E bedsets of more distal FA2 deposits highlight that much of the sediment transport to foreset and bottomset positions has been facilitated via sediment gravity flows. Well-cemented event bed tops attest that longer periods of non-deposition and bypass accompanied offshore-directed sand and mud transport across the OCC, via sustained delivery of river-fed sediment and potential slope failure at the comparatively steep delta front (Fig. 3) (Clare et al. Reference Clare, Clarke, Talling, Cartigny and Pratomo2016). The continuous facies succession of a prograding clinothem from distal offshore muds to thick, amalgamated topset sandstone is inferred for the clinothem portion above 20 m stratigraphic height. The underlying shoreface–shelf succession below 20 m stratigraphic height, however, does not show a classic onshore–offshore facies succession (sensu Aigner & Reineck, Reference Aigner and Reineck1982; Clifton, Reference Clifton, Posamentier and Walker2007; Plint, Reference Plint, Dalrymple and James2010). Instead, it is interpreted to represent a small number of amalgamated and incomplete parasequences, most likely linked to episodic, partial bed removal and sediment bypass on the OCC delta top. In order to explain the observed contrast in mudstone composition across the OCC not only the delivery mechanism is considered, but also the density contrasts among the original mineralogical assemblage, which is inferred to have included highly variable amounts of volcanoclastic material. The inferred hydrodynamic ‘sieving’ of sand-sized mafic higher-density grains (i.e. olivine, pyroxene, amphibole) and mafic lithoclasts (versus quartz) (Fig. 4d) closer to the shoreline might explain the prominent shift in the chlorite/illite ratio at ∼10 m stratigraphic height (Fig. 9). The increase in overall clay content (Fig. 9) as well as the increase in the ratio of mudstone to sandstone beds is interpreted to coincide with increasing shoreline proximity and water depth.
It is proposed that the wave-induced turbulence remained insufficient on the OCC to maintain this high iron, high density mineral assemblage of riverine-sourced, sand-sized mafic mineral and lithoclasts in suspension as the water deepened and/or wave-orbital velocities waned. However, combined, wave–current flows that involve two forcing mechanisms, and are therefore more effective at sediment transport than waves or currents alone (e.g. Grant & Madsen, Reference Grant and Madsen1986), could have facilitated offshore-directed sediment transport.
The observed onshore–offshore change in grain size and clay origin (Figs 9, 11) most likely reflects a change in input volume and frequency of high volumes of unstable mafic detritus as well as feldspar (as a result of changing volcanic activity), or a more direct change in transport path, possibly via autocyclic river avulsion and shifting distributory channels (e.g. Catuneanu & Zecchin, Reference Catuneanu and Zecchin2013). The overall coarse grain-size distribution of the proto-mud fraction within OCC deposits and the general absence of widespread cohesive clay in the Bell Island Group (Harazim & McIlroy, Reference Harazim and McIlroy2015) might explain the steep nature of the OCC, as well as its relatively small size compared to other, larger clinothem systems that extend over several tens to hundreds of kilometres (Johannessen & Steel, Reference Johannessen and Steel2005; Pattison et al. Reference Pattison, Ainsworth and Hoffman2007; Varban & Plint, Reference Varban and Plint2008; Patruno et al. Reference Patruno, Hampson and Jackson2015; Poyatos-Moré et al. Reference Poyatos-Moré, Jones, Brunt, Hodgson, Wild and Flint2016).
7. Conclusions
The OCC on Bell Island, Newfoundland, serves as a key archive for understanding the sedimentology and diagenesis of early Palaeozoic shelves, where most of its detrital components have been delivered via mechanical weathering from a non-vegetated hinterland. Based on our integrated sedimentological and compositional analyses, the facies architecture of the OCC can be genetically linked to: (1) deltaic sediment input; (2) offshore- and alongshore-directed sediment dispersal driven by storm waves and unidirectional currents; and (3) relative chemical grain stability during burial and diagenesis.
Detailed facies analyses reveal that sediment transport on the different architectural elements of the muddy shelf clinothem (i.e. the shoreface topset, foresets and inner shelf bottomsets) was driven by different mechanisms that reflect local hydrodynamic conditions. In proximal regions of the shelf clinothem, wave processes transported relatively coarse-grained sediment while in more seaward environments, currents play an increasingly important role in sediment transport, whereby the combined energies of waves and currents are capable of transporting relatively coarse-grained sediments when wave-orbital velocities alone are insufficient. Waves are inferred to influence sand and mud dispersal even across foreset and bottomset regions and therefore suggest much of the OCC has been deposited above storm-wave base.
A portion of the present-day clay mineral fraction represents the alteration product of highly unstable mafic (lithic) grains that were delivered to the shelf as silt-sized particles where they formed a fraction of the framework components. These lithic fragments were diagenetically altered to chlorite before significant compaction occurred. It is important to consider this fraction of the chlorite in the rocks separately from detrital chlorite when using clay mineralogy as a proxy for provenance.
The early Palaeozoic rocks on and around Bell Island capture a critical role in our developing understanding of how fine-grained, early Palaeozoic clastic dispersal systems functioned; especially since they provide a gateway to examine the conditions of in situ clay mineral production, prior to the evolution of widespread soil-based clay mineral factories from the Devonian onwards. To test the robustness and validity of in situ weathering, as an important ‘clay factory’ through pre-Devonian time, it will require more rigorous sedimentological analysis of more exposures on Bell Island and in the Conception Bay area as well as expansion of this research effort into similar successions which are even older.
Acknowledgements
This work was supported by the Billy and Anne Harrison endowment for sedimentary geology (S.J.B.) and by student research grants from the American Association of Petroleum Geologists (AAPG), the Geological Society of America (GSA) and the Society for Sedimentary Geology (SEPM) awarded to K.C.D. Rick Young (Louisiana State University) is thanked for his assistance with hand specimen preparation, and Wanda LeBlanc (Louisiana State University) is thanked for performing all QXRD analyses. Dr Sven O. Egenhoff is thanked for reviewing an early version of the manuscript.