Introduction
Glaciomarine sediments (GMS), melted out and reworked from floating ice, comprise crudely sorted terrigenous sediments possessing an abundant silt-size fraction with scattered cobbles and associated alteration products, but poor in CaCO3 and other organic matter. Current hypotheses of glaciomarine sedimentation (e.g. Carey and Ahmed (Reference Carey, Ahmed and Raasch Carey and Ahmed 1961) are based upon sketchy a priori assumptions and often upon outdated concepts. This study presents ideas for the interpretation of ice-rafted debris (IRD), based upon glaciological and oceanographic models. The marine zone around Antarctica is taken as a type area for discussion.
Four factors may be identified which control IRD (summarized in Figure 1): (1) nature of sediments in ice arriving in the marine environment; (2) nature of the transition from grounded to floating ice; (3) processes which operate at the ice/water interface of ice tongues and ice shelves, governing the amount of melt, if any, and, hence, the release of debris; (4) within the open ocean, IRD sedimentation is controlled by drift trajectories, melt, and break-up history of those icebergs which contain debris.

Fig.1. Composite depiction of processes and patterns of glaciomarine sedimentation.
Nature of sediments arriving at grounding line
Glacial sediments destined for deposition in the ocean are incorporated into grounded ice masses at their surfaces, but more importantly by entrainment at the bed (Fig. 1). Basal debris-rich layers extend upwards from a few centimetres to several tens of metres. Typical values for margins of polar ice sheets appear to place the thickness of the debris layer at −1% ice depth.
A basal debris layer may comprise several discrete bands of sediment-rich ice and the average concentration is extremely variable, from a fine dispersion (0.01% by volume) to upwards of 60 to 70%. For an average polar ice sheet, the value is probably −1%. Observations of ice cliffs and cores indicate that there is usually a thick basal horizon of similar sediment content above which concentration shows a marked exponential decrease. Reference YevteyevYevteyev S A (1959) has presented a detailed study showing this variation from ice cliffs near Mirny (Fig. 2). In order to model melt-induced debris release in later sections, we have represented the vertical variation in debris concentration by a Heaviside function of the form:

Fig.2. Debris characteristics for basal ice. Left: debris-concentration results of Reference YevteyevYevteyev S A (1959) for ice cliffs near Mirny. Equation 1 is shown [C0 = 0.12; kl = 0.1; k2 = 8 m]. Spot value for basal debris in Byrd core is also shown. Right: an inferred depth-particle-size curve for a typical basal section of Antarctic ice.

where C is concentration at any height (y) above bed, C 0 is initial concentration of basal layer, K 1 is constant, and K 2 is constant proportional to thickness of basal layer of constant debris concentration. U0 (0) and U0 (y 1) are Heaviside unit step functions. Equation 1 is depicted in Figure 2.) The nature of the debris concentration function (DCF) at the grounding line is an extremely important factor in determining subsequent sedimentation rate beneath a floating ice mass. If, for instance, the melt rate and ice velocity were constant, sedimentation rate would directly mimic the DCF. Too few measurements of basal debris are available to suggest how typical Equation 1 may be.
Grounding-line transition
Excluding lodgement and melt-out of clastic materials beneath the grounded ice sheet, debris and ice are transferred out of the ice sheet system in three modes.
(a) Ice cliffs
According to Bardin and Suyetova Reference Bardin, Suyetova, Bardin and NagataBardin V I, Suyetova I A (1967), ice cliffs account for −16% annual ice discharge into the seas surrounding Antarctica. Mass loss is small (0.017 km3 km−1 a−1) and occurs principally by ice fall from unstable advancing ice cliffs. Wave action at the foot of ice cliffs and submergence of bottom sections of the ice front, and their slow rate of advance (5 to 50m a−1) when compared to outlet glaciers and ice shelves, promote bulk sedimentation close to the ice edge prior to calving.
(b) Outlet alaciers and ice streams
Inland ice draining towards the coast may become channelled into ice streams or outlet glaciers, the latter constrained by rock walls. Bardin and Suyetova Reference Bardin, Suyetova, Bardin and NagataBardin and Suyetova (1967) indicate that discharge per unit width is high (0.091 km3 km−1 a−1) and total annual discharge accounts for 22% of Antarctic mass loss into the sea.
Observations of icebergs calving from the front of outlet glaciers, including those that pass through a mountain belt, show that they retain basal debris (up to 15m thick) plus a variable thickness of near-surface and recently acquired moraine (Fig. 1)(Reference Bellair, Tourenq and VernhetBellair and others 1964, Reference Anderson, Domack and KurtzAnderson and others 1980). The release of sediments by melting beneath outlet glacier tongues is similar to that under ice shelves. As there is an almost continuous supply of small bergs with a high debris content (mean size < 1 km) from outlet glaciers (compared to high-magnitude low-frequency calving of ice shelves), we suggest that the major portion of sediments discharged into the Southern Ocean comes from these outlet glaciers. Most icebergs calving from ice shelves are devoid of sediments.
(c) Ice shelves
Grounded ice may decouple upon entering deep water and float out over the sea where it experiences creep thinning from acceleration due to reduction of lateral and basal drag (Fig. 1). Reference Bardin, Suyetova, Bardin and NagataBardin and Suyetova (1967) indicate that ice shelf termini constitute the greatest discharge element for Antarctica at 62%.
Important for sedimentation considerations are mass balance and particle paths. Ice shelves receive considerable surface snow accumulation. As rates increase towards the ice front, particle paths are inclined downwards at progressively steeper angles. If there is net basal melt, particle paths will intersect the bottom and any debris in transit in basal layers is likely to be rapidly melted out prior to calving at the ice-front (Fig. 1), making ice shelves important for sedimentation at the inner margins of the continental shelf but of minimal significance to open-ocean glaciomarine sedimentation.
Modelling basal melt and sedimentation from ice shelves and outlet glaciers
Melting theory
When ice decouples from bedrock at the grounding line two possible regimes may develop at the ice/water interface. These are basal melting or freezing, and are governed by the upward heat fluxes from the sea (Q s) and through the ice (Q i) (Reference DoakeDoake 1976):

where M is melt rate (negative for freezing), L is latent heat of fusion of ice, ρ iis density of ice.
Q s is a function of temperature above freezing, flow characteristics, and thermohaline convection, if it occurs. Q i is the product of temperature gradient in the ice and thermal conductivity. As few data are available on the oceanographic regime beneath floating ice masses, indirect methods are required to estimate basal melting and freezing. Reference Crary, Robinson, Bennett and BoydCrary and others (1962) have given the following relationship based upon the assumption of steady state:

where ν is horizontal velocity, dH/dx is ice-thickness gradient along flow-line (x direction), A is surface accumulation rate, and ε z is vertical strain rate = –(ε x + ε y ). Figure 3) shows computed melt rates from Equation 3 along flow lines for the Brunt and Ross ice shelves. In , general, on larger ice shelves, melt rates decrease up the flow line from the ice front where the effect of active water circulation is greatest for heat exchange. Further inland, as heat exchange is weakened and a layer of cold isothermal low-salinity water develops, a zone of net basal freezing may be encountered, and enhanced where the ice base slopes strongly upwards(Reference RobinRobin 1979). Towards the grounding line, however, basal melting may again occur resulting from convective turbulent flow associated with tidal pumping and general circulation. Some investigators, however, suggest basal freezing may continue to the grounding line (Reference ThomasThomas 1976, Reference Zotikov, Zagorodnov and RaikovskyZotikov and others 1980).
The amount of basal melt or on-freezing in any small horizontal element (dx) along a flow line is:

where M is melt/freeze rate, and ν is horizontal ice velocity. The quantity of sediment released at any point from the ice base will depend upon the vertical debris concentration function (Equation 1) and up-stream sedimentation melt history as given by integration of Equation 4. As M and ν vary along the flow line, Equations 1 and 4 have been solved numerically for two Antarctic ice shelves (Brunt and Ross). As the DCF is unknown, Equation 1 has been applied with variable thickness and volume concentration. The results are shown in Figure 3.

Fig.3. Melt and sedimentation curves for Brunt(left) and Ross (right) ice shelves using Equations 1, 3, and 4. Chebyshev polynomials have been fitted to all ice thickness, velocity, accumulation, and strain-rate raw data. Sources: ice-thickness data from SPRI radio echosounding, Brunt data from Reference ThomasThomas (1973), Ross data from Thomas (personal communication).
Sedimentation
It can be seen in Figure 3 that, due to the relatively thin basal debris zone, sedimentation is highly sensitive to melt rate. Strong melting close to the grounding line, for instance, may remove all debris within the first few tens of kilometres of ice shelf. The effect of basal freezing prior to complete release of sediments is to shift the deposition curve further along the flow line. These findings are supported by two observations. First is the total absence of debris in core retrieved from the Ross Ice Shelf at J9 and the Amery Ice Shelf at Gl. At J9 6 m of frozen-on sea ice were observed at the bottom of a 416 m core. Various estimates suggest an accretion rate of between 0.01 and 0.035 m a−1(Reference Jacobs, Gordon and ArdaiJacobs and others 1979, Reference Zotikov, Zagorodnov and RaikovskyZotikov and others 1980). Reference MorganMorgan (1972)interprets the Amery core as comprising 270 m of glacier ice overlying 158 m of frozen-on sea ice with an inferred growth rate of 0.3 to 0.5 m a−1, As the ice streams feeding both the J9 and Glsites are vigorous, possess beds at the pressure-melting point, and have velocities indicative of basal sliding, it I unlikely that bottom layers will be devoid of debris at the grounding line. It must be assumed, therefore, that melt releasing sediments must take place further up-stream from the drill sites and close to the grounding line. Additional evidence for this explanation comes from the Maudheim Ice Shelf where Reference RobinRobin (1958) has reported that the seismic record from station 167, just beyond the grounding line, suggests the presence of a basal moraine layer (~10 m thickness), while seismograms further out on the ice shelf show no such effect.
Secondly, geophysical studies in the grounding zone of both Ross and Filchner Ronne ice shelves suggest the presence of rapidly accumulating sediments. Radio echo-sounding of the Filchner Ice Shelf indicates that its inner boundary rests upon soft unconsolidated water-saturated sediments over an area several tens of kilometres wide resulting from rapid melt-out of debris during initial stages of decoupling (Reference Drewry, Meldrum and JankowskiDrewry and others 1980). Close to the grounding line of the Ross Ice Shelf, studies demonstrate only a thin and variable water column between ice shelf and unconsolidated strata with several regions of incipient grounding (Robertson and others, in press).
Under steady-state conditions, therefore, we suggest that the bulk of basal debris is melted out close to the grounding line, producing a zone of sediment progradation (Fig.4).There is a gradual facies change from coarse orthotills (Reference Harland, Herod and KrinsleyHarland and others 1966)beneath melting ice streams to aqueous orthotills as ice becomes buoyant. Bottom traction currents generated by cold-water flow or from large-scale circulation may induce local scour and erosion. Preferential movement of finer fractions by this process results in gravel lags and production of residual paratills (Fig.4). Further from the grounding line the rate of sedimentation will drop rapidly with only finer material being deposited, due to the exponential nature of the DCF and particle-size distribution (Fig.2). With bottom-current transportation towards the open sea, paratills thus grade into a thin wedge of non-calcareous silts and clays with occasional dropstones.
Ice shelves and outlet glaciers are the most sensitive elements of large ice masses: they respond rapidly to sea-level and climatic changes. Frequent migration, therefore, over the continental shelf of the grounding line zone will produce a thick, complex, and strongly diachronous sequence of glaciomarine sediments (Fig.4).
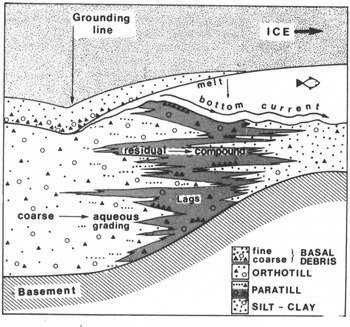
Fig.4. Sedimentary facies generated by floating ice shelf or outlet glacier exHibiting nonsteady state behaviour (irregular oscillation of grounding line) but no major erosive events are shown. See text for discussion.
Iceberg sedimentation
Icebergs are produced from ice shelves, outlet glaciers, and ice cliffs, with size diminishing in that order. They are extremely important for open-ocean IRD sedimentation (i.e. up to 1000km from the Antarctic coast). The processes of debris release, however, are poorly understood but depend upon mechanisms of calving, subsequent fragmentation, location, and quantity of debris, drift, and melt histories (Fig.l).
(1) Debris content of icebergs
The debris content of bergs will depend on their calving source. Most ice shelves will not produce bergs containing significant amounts of basal debris (sediment content can be computed using Equations 1 and 4). Any debris present may consist of englacial sediment ribbons from ice flowing into the ice shelf through exposed rock terrain. This may be the cause of banded icebergs observed off the Antarctic Peninsula. In the case of small ice shelves or those whose basal regime exceptionally favours extensive zones of bottom freezing, it is possible for limited amounts of basal debris to be present. Outlet glaciers, however, appear to be the dominant source of debris-rich icebergs due to their thick basal moraine layers, short length of floating section, and rapid calving.
(2) Iceberg trajectories and velocities
Typically, the drift of lcebergs will depend upon predominant winds and currents, oceanic fronts, pack ice conditions, and seabed topography. Until the advent of satellite surveillance it was difficult to track iceberg movements continuously with ease. and reliability. Even today few thorough studies have been undertaken to provide drift characteristics (e.g. Reference VinjeVinje 1980).
Reference Tchernia and JeanninTchernia and Jeannin (1980) tracked several icebergs around the coast of east Antarctica by means of transponders which were attached to the bergs and which relayed to Eole and Nimbus F satellites. Average speeds recorded over periods of several hundred days ranged between 0.11 and 0.20ms−1. Reference TcherniaTchernia (1974) reports that such drift is between 60 and 75% of the ocean current velocity. The icebergs in question ranged in size from 0.4 to 3.8 km (length) with freeboards of 30 to 50 m. Parts of the trajectories of several bergs showed a pronounced but irregular east-west movement close to the Antarctic coast line (100 to 150 km) in the zone dominated by the East Wind Drift. Four icebergs showed distinctive recurvature of their trajectories as they moved progressively further from the coast. Such motion indicates transition from the influence of westward flow close to the continental slope to the West Wind Drift, the primary circulation pattern of the Southern Ocean.
This twofold distinction of drift tracks is important for distribution of sediments. Many icebergs tend to drift around Antarctica from east to west close inshore where waters are colder and constraining effects of thicker pack ice are greater and debris release consequently slower. Nevertheless, periods of several years spent in this zone (Reference VinjeVinje 1980) probably result in most basal debris being released on the continental shelf, generating an extremely coarse inner girdle of IRD. Bergs pushed towards the edge of the pack come under the influence of west-east currents and local gyres. Icebergs may then be swept both east and north into warmer waters where their melt rates are enhanced.
(2) Debris release mechanisms
(a) Melt above the water Line
Debris cropping out on the surface or sides of an iceberg is released by incoming solar radiation, giving rise to periodic debris falls, especially of coarse material, or a continuous rain of finer sediments. Close to the Antarctic coast, such release will be small due to low atmospheric temperatures and positive mass balances (the latter being reported for bergs south of 66°S in the Weddell Sea (Reference OrheimOrheim 1980)). Nevertheless, the significantly reduced albedo of dirty ice may assist preferential melt-out during summer periods.
(b) Melt at and below the water Line
Wave action gives rise to both water-line melting and collapse of undercut cliffs but, unless substantial amounts of debris are exposed at these locations, the sedimentational significance is not great. Melting of submerged side walls will also release debris and Reference HuppertHuppert (1980) has calculated that rates of melt are in the order of m a−1.
Although overturned icebergs may possess debris in almost any place, it is most likely for sediments to reside in the base. Bottom melting of icebergs is similar to that of floating ice attached to land, except that drift velocity, the effects of currents, and other oceanic factors are more pronounced. In general, melt rates are lower than those for side walls (Reference HuppertHuppert 1980). Several studies have suggested rates of bottom melting varying between 0.1 and 100 m a−1. Martin and Kauffman(Reference HuppertHuppert 1980) give extrapolated bottom melt rates in the order of 0.5 to 1 m a−l, similar to theoretical suggestions by both Reference GadeGade (1979) and Reference Weeks and CampbellWeeks and Campbell (1973). Reference Budd, Jacka and MorganBudd and others (1980), however, report average, all-round ice loss (i.e. buttom and sides) two orders of magnitude greater, obtained from consideration of the steady-state flux of icebergs in the Southern Ocean. These values are similar to those obtained in laboratory experiments by Reference Russell-HeadRussell-Head (1980). We have calculated melt rates for given water temperatures using: (i) Weeks and Campbell's method, (ii) Russell-Head's experimental relationship, and (iii) the Reference VinjeBudd and others (1980) envelope. Results are given in Table I. If icebergs are held close into the Antarctic coast for up to five years (Reference VinjeVinje 1980), all basal debris would be released prior to drift into the open ocean. Only fragmentation and overturning would preserve material in the iceberg for rafting at great distances from the coast. It is not certain, however, whether basal debris layers inhibit or enhance bottom melting. Laboratory experiments should be undertaken to evaluate factors important in heat transfer through a sediment-charged basal horizon.
Table I Melt Rates Fdr Iceberg Base (M berg in m a−1)

where L is constant, V b is relative free stream flow velocity (= 0.05 m s−1) and x b is iceberg length (= 1 km). ΔT is ice/water temperature difference.
(c) Dumping
During fragmentation, tilting and overturning of icebergs, any loose sediments may simply be dumped into open water giving rise to periodic sedimentation events. In addition, upturned bergs containing debris may concentrate sediment at their upper surfaces by melting from solar radiation. Periodic saturated flow or slumping of such debris may release material over the sides of a berg (Reference OvenshineOvenshine 1970). Meltwater rivulets also carry away fines in suspension, or larger particles in traction.
Figure 5 illustrates a hypothetical debrisrelease history for an outlet glacier berg. Dumping, mudflow, dirty melt-water contributions, and basal and radiation melt episodes give rise to highly irregular and complex patterns of sedimentation, such as sporadic patches of coarse or mixed deposits lying upon and often interbedded with finer silty sediments. Nevertheless, there is a steady decline in rate of deposition as the berg is progressively cleaned of debris. Several of these events are shown in Figure 5.

Fig.5. Hypothetical debris-release history for icebergs calving from ice stream/outlet glaciers.
Acknowledgement
Radio echo-sounding data used in this study were gathered in a programme funded by the U.K. NERC Grant GR3/2291 and with the generous logistic support of the U.S. National Science Foundation. We thank Dr C.S. Neal for helpful discussions, S. Jordan for assistance with diagrams, and Dr R.H. Thomas for providing unpublished data for the Ross Ice Shelf.