Introduction
Cognitive evolution did not occur as a single, or even a few, ‘revolutionary’ episode/s, nor as a sudden genetic mutation (sensu Coolidge & Wynn Reference Coolidge and Wynn2005; Klein Reference Klein2000). Instead, we can accept that it was a long and complex developmental path of material engagement (Malafouris Reference Malafouris2013) and mutational enhancement (Garofoli Reference Garofoli, Etzelmüller and Tewes2016), incrementally broadening mental flexibility to cope with natural and/or socio-economic circumstances in innovative ways (e.g. Haidle et al. Reference Haidle, Bolus and Collard2015). Aspects of cognition probably also developed in parallel, and evidence for change does not necessarily imply that aspects of preceding levels of cognition ended their evolutionary trajectories (e.g. Gärdenfors & Lombard Reference Gärdenfors and Lombard2018; Lombard & Gärdenfors Reference Lombard and Gärdenfors2017). In terms of Earlier Stone Age/Lower Palaeolithic technologies, several authors have recently demonstrated gradual cognitive developments. For example, the hierarchical complexity in the production of handaxes is well explored (e.g. Mahaney Reference Mahaney2014; Stout et al. Reference Stout, Apel, Commander and Roberts2014). Stout and colleagues (Reference Stout, Hecht, Khreisheh, Bradley and Chaminade2015) investigated the neurophysiological demands of stone-tool making through lithic experimentation, while Herzlinger and colleagues (Reference Herzlinger, Wynn and Goren-Inbar2017) discussed expert cognition involved in cleaver manufacture, and Muller and colleagues (Reference Muller, Clarkson and Shipton2017) reconstructed the hierarchical complexity and attention spans associated with different lithic technologies.
Yet interpretations of what stone artefacts and techno-behaviours associated with their production can reveal about our cognitive evolution remain divided. Some argue for little or no variation in behaviour and cognition when comparing non-human primate tool use such as chimpanzee nut cracking with early lithic technologies (e.g. Tennie et al. Reference Tennie, Premo, Braun and McPherron2017; Wynn et al. Reference Wynn, Hernandez-Aguilar, Marchant and McGrew2011). Others argue that ‘motor and cognitive-perceptual demands necessary for nut-cracking are inferior to those of stone-flaking’ (Bril et al. Reference Bril, Parry and Dietrich2015, 11).
Here we argue that an interpretation of cognitive equivalence between nut cracking and stone-tool knapping is too simplistic, as it was reached from methods focusing on the tool and its life history without considering the broader techno-behaviour. With a hammer as tool and/or anvil in focus, the studies of hominin percussion techniques generally end with the manufacture of a flake and the discard of the hammer, as do the studies of chimpanzee percussion techniques with the opening of nuts and the discard of the hammer. From an activity-based perspective, however, the hominin flake was only an intermediate target, applied as a cutting tool on other objects to obtain the final target, whereas in chimpanzee nut cracking the final target is reached with the opening of the nut.
We add to the body of work that aims to assess variation in levels of cognition associated with present-day capuchin rock pounding and chimpanzee nut cracking, and the oldest known intentionally knapped stone artefacts from the 3.3-million-year-old site of Lomekwi 3 in West Turkana, Kenya (Harmand et al. Reference Harmand, Lewis and Feibel2015; Lewis & Harmand Reference Lewis and Harmand2016). With our multi-model approach, we explore similarities and differences between 11 techno-behaviours and components thereof, used by wild apes and monkeys, and the earliest known flaking techniques used by extinct hominins. We discuss these techno-behaviours in terms of cognigrams (see Haidle Reference Haidle2014), the expert cognition model (Wynn et al. Reference Wynn, Haidle, Lombard, Coolidge, Wynn and Coolidge2017), the seven-grade causal cognition model (Lombard & Gärdenfors Reference Lombard and Gärdenfors2017) and modes of teaching that can be inferred from the archaeological record (Gärdenfors & Högberg Reference Gärdenfors and Högberg2017), to provide an integrated interpretation of cognitive variability.
Our approach
Below we introduce the basic principles used in the analytical and interpretative components of our study of different techno-behaviours. Readers are also referred to the documents cited in this section for comprehensive discussions of the various models. Throughout our analysis, and as a matter of caution, we opt for the simplest possible cognitive explanations, to prevent inflation of levels of cognitive complexity.
Cognigrams: perception-and-action sequences encoded
Cognigrams have been used effectively in cognitive archaeology for almost a decade as tools to ‘think through’ technologies in a two-tiered approach. First, they systematically encode the perception-and-action sequences providing contextualized, detailed procedural understanding of the associated techno-behaviours (Haidle Reference Haidle2012; Haidle et al. Reference Haidle, Garofoli, Scheiffele, Stolarczyk, Etzelmüller, Fuchs and Tewes2017). Secondly, the sequences and interpretation thereof can be used to hypothesize about aspects of cognition reflected by a specific techno-behaviour (e.g. Haidle Reference Haidle, de Beaune, Coolidge and Wynn2009; Reference Haidle2014; Lombard & Haidle Reference Lombard and Haidle2012; Wragg Sykes Reference Wragg Sykes, Coward, Hosfield, Pope and Wenban-Smith2015). Thus far, the use of cognigrams and subsequent interpretations has been instrumental in providing discussion on established cognitive aspects such as working memory (Haidle Reference Haidle2010), problem-solution distance and cognitive modularity (e.g. Haidle Reference Haidle2012; Hodgskiss Reference Hodgskiss2014; Lombard Reference Lombard2012; Reference Lombard, Haidle, Conard and Bolus2016; Lombard & Haidle Reference Lombard and Haidle2012), the evolution of causal cognition (Haidle Reference Haidle2014) and material engagement and embodied cognition (Haidle et al. Reference Haidle, Garofoli, Scheiffele, Stolarczyk, Etzelmüller, Fuchs and Tewes2017). Their application has also been tested experimentally by Muller and colleagues (Reference Muller, Clarkson and Shipton2017) and served to inspire new methodological approaches, such as analysing changes in cognitive task-structuring strategies across different hominin tool-making behaviours (Fairlie & Barham Reference Fairlie and Barham2016).
In broad terms, cognigrams represent parsimonious interpretations of behavioural units with an initial stimulus or need and an end in its satisfaction or abandonment. Instead of following the life history of an artefact as in chaînes opératoires, cognigrams follow the perceptions and actions of a subject in a specific operational context. The resulting perception-and-action sequence is a hypothetical reconstruction of the performance based on a summary of evidence gathered from, for example, technological, functional and spatial analyses of archaeological remains, replication experiments and ethological or ethnographic observations or descriptions. This evidence is supplemented by realistic assumptions about phases of material procurement, the transport of different elements and repeated interruptions of the process by other urgent needs. Cognigrams are therefore not merely a depiction of primary observations, but are enriched by secondary or tertiary sources and the interpretations thereof.
Thus, the reconstruction of techno-behaviours through encoding them in cognigrams allows for nuanced discussions about differences and similarities across species, contexts and sources (e.g. Haidle Reference Haidle2012; Haidle et al. Reference Haidle, Garofoli, Scheiffele, Stolarczyk, Etzelmüller, Fuchs and Tewes2017). Minor variability in the sequence of actions is included in the systematic encoding, and depending on the specific research interest, elements of the sequence can be summarized or unfolded. Also, as is true for all qualitative approaches, a certain level of interpretative subjectivity is expected. Yet, if the approach is conducted thoroughly and systematically, and based on the same set of archaeological, ethological, ethnographic and/or experimental observations, variation in inferred outcomes should not be radical.
Cognigrams consist of two parts, a description of a perception-and-action sequence and its graphic implementation. The texts provide details with regard to contents of the perceptions and actions, while the graphics give a visual overview and highlight relations between the different elements, such as association of actions to attention foci or effects of a focus on another focus. In Figure 1, we provide the graphical symbols used to encode perception-and-action sequences in our cognigrams (for a full discussion of method, see Haidle Reference Haidle2012). Starting with the subject's perception of a basic need, a series of subsequent problems is perceived, opening new attention foci, which are acted upon to satisfy the basic need. The attention foci can be classified as active, when they are actively controlled by the subject and act upon other foci. They can encompass the subject itself, or the tools that act on objects. In contrast, passive foci are locations, objects or aids (natural or created) that are acted upon. In the cognigrams, the different elements of a behaviour are represented in active and passive attention foci, perceptions of need opening the attention foci, actions within or directed to an attention focus, effects of attention foci on other attention foci or on other effects. Operational steps are located within the context of each focus and further grouped into phases that have to be executed as a unit, or when interrupted, started again with the first action of the phase.
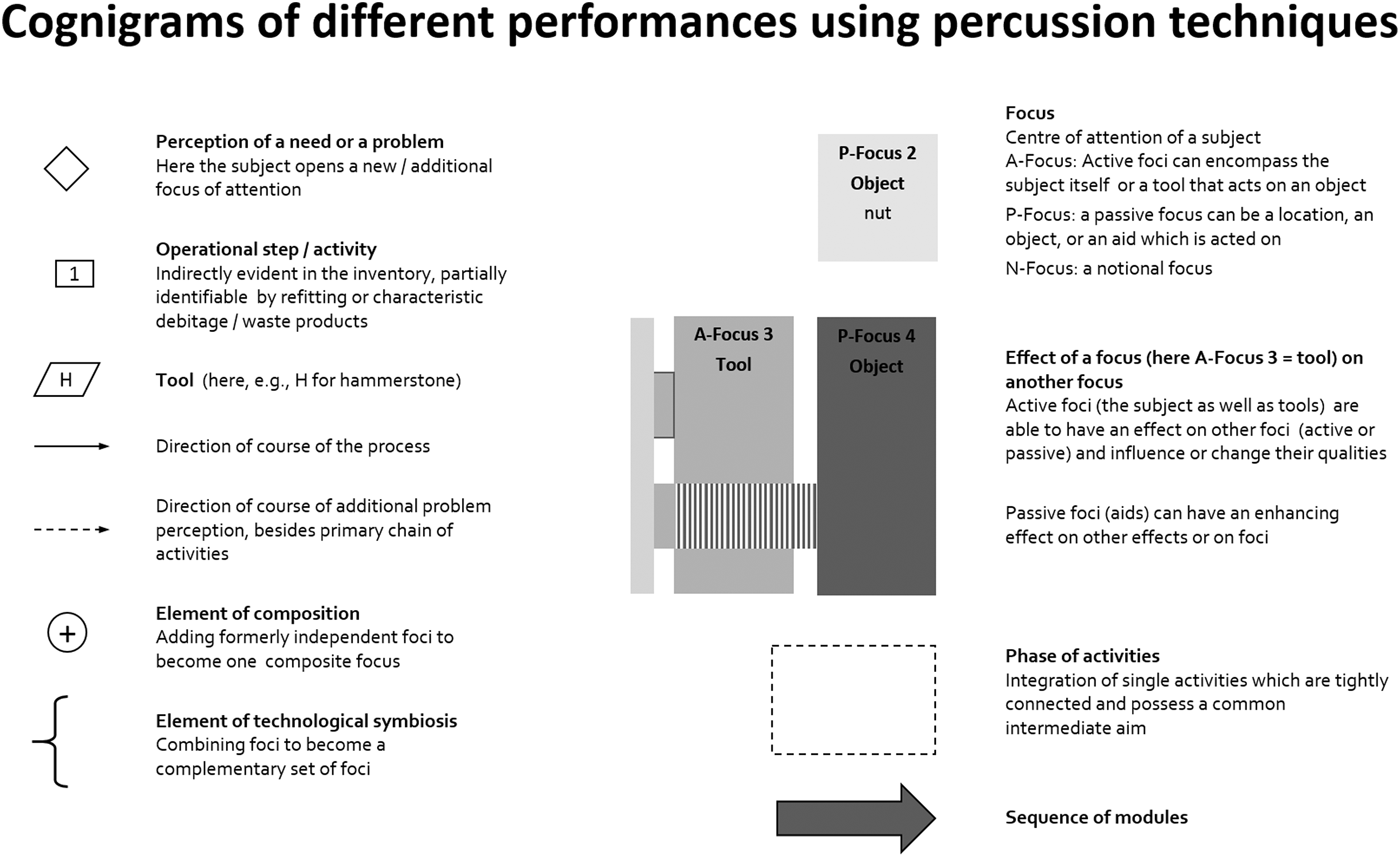
Figure 1. Graphical symbols and definitions used for encoding perception-and-action sequences in cognigrams.
With secondary tool use, i.e. using tools to produce tools to solve a problem, an extension of the problem-solution distance becomes evident. Not only do intermediate targets in direct connection to the satisfaction of the basic need have to be perceived, but also tools have to be prepared in advance, changing the status of an object to become the tool, to solve the problem. High levels of complexity in tool behaviour are possible only by conceptual and technological modularization—by separating large problem settings into small modules, each with its own intermediate aim. To reach the final target of the large problem setting, the individual modules have to be linked to each other in specific hierarchical sequences. From an analytical perspective, the reconstructed modules have to be depicted in separate cognigrams, even though they are only parts of the larger problem setting.
It is important to understand that the cognigrams are schematic representations used to illustrate techno-behavioural sequences by encoding them into a range of interconnected elements and procedures. They do not equal ‘cognitive steps’. Instead, they provide a rich understanding of how the elements and procedures interact with each other and their hierarchical structure within any given techno-behaviour. Because of the systematic approach, elements and procedures captured within the cognigrams can be highlighted or even counted to generate directly comparable impressions regarding variation in levels of techno-behavioural complexity. It is then the variation in such complexity that provides the basis for hypothesizing about aspects of cognition represented by a specific techno-behaviour compared to another (e.g. Lombard & Haidle Reference Lombard and Haidle2012).
Here we use cognigrams to ‘think through’ 11 techno-behaviours, providing visual interpretations and thick descriptions for each. We then proceed with new comparative analyses to hypothesize about concepts such as item-attention range, problem-solution distance (planning depth) and maximum attention span. All three of these concepts have theoretical grounding in cognitive research. For example, in terms of item-attention range, Alvarez and Cavanagh (Reference Alvarez and Cavanagh2004) showed that both the visual information load and number of objects impact on short-term memory capacity (also see Lombard & Haidle Reference Lombard and Haidle2012 regarding symbiotic technologies). Solving problems or planning through time and outside the immediate sensory context (i.e., planning depth) has been shown to operate across a range of cognitive domains, such as mental representation of a temporally distant event and the ability to buffer current sensorial input in favour of a delayed/imagined goal (e.g. Kabadayi & Osvath Reference Kabadayi and Osvath2017), and is used extensively to discuss the cognitive abilities of Stone Age populations (e.g. Ambrose Reference Ambrose2010; Davidson Reference Davidson2010; Goren-Inbar Reference Goren-Inbar2011; Shipton et al. Reference Shipton, Petraglia, Paddayya, Adams and Blades2009; Wadley Reference Wadley2010; Reference Wadley2013). Kirova and colleagues (Reference Kirova, Bays and Lagalwar2015) recently demonstrated a direct link between attention span and the cognitive concepts of episodic memory, working memory, and executive functions. These concepts are well-grounded in cognitive theory (e.g. Tulving Reference Tulving1985), and have been used extensively to inform approaches to cognitive archaeology (e.g. Coolidge & Wynn Reference Coolidge and Wynn2005; Reference Coolidge and Wynn2008; Overmann et al. Reference Overmann, Wynn, Coolidge, Alloway and Alloway2013; Wadley Reference Wadley2013; Wynn et al. Reference Wynn, Haidle, Lombard, Coolidge, Wynn and Coolidge2017).
Expert cognition
One of the aspects that distinguishes the evolutionary trajectory of humans from other primates is our ever-increasing technological sophistication, built (at least partly) on skilled technical cognition. Much of such thinking can be explained through expert cognition. Simply put, expert cognition relies on the effective chunking and chaining of information (memory and sensory) series (see Wynn et al. Reference Wynn, Haidle, Lombard, Coolidge, Wynn and Coolidge2017 for most recent discussion of the model). Importantly, expert cognition does not require the high levels of working memory, flexible executive functioning or a fully modern episodic memory system necessary for some complex technological performances. We have recently summarized the broadly accepted behavioural characteristics of expert cognition (Wynn et al. Reference Wynn, Haidle, Lombard, Coolidge, Wynn and Coolidge2017, 23):
• Novices require years to attain mastery.
• The expert performs at a high level of accuracy and reliability, making few ‘unforced’ errors.
• The expert makes rapid, in-depth, assessments of problems.
• Experts can be interrupted and return to a task or problem with little or no loss of information.
• Experts learn new material in their field of expertise very rapidly.
• Expert performance is limited to narrow domains of activity.
• Expert responses appear largely automatic, requiring little in the way of active attention.
Our initial expert cognition analysis, although coarse-grained, revealed two interesting prospects. First, that the basic elements of expert technical cognition had deep evolutionary roots, already available to early hominins, on which the Homo lineage elaborated to become the ultimate masters in technical performance. Secondly, that procedural memory chains are probably longer and more numerous for expert Oldowan knapping compared with expert non-human primate nut cracking, suggesting a modest increase in long-term memory associated with simple core knapping technologies.
Grades of causal cognition
Causal cognition allows humans to predict outcomes based on observations, to affect and control events in the world around us and to predict causes from effects, even when such causes cannot be perceived (Lombard & Gärdenfors Reference Lombard and Gärdenfors2017). A strong link seems to exist between tool use and causal cognition (e.g. McCormack et al. Reference McCormack, Hoerl and Butterfill2011). It has been suggested that non-human, tool-using primates understand basic associations between a tool and its effects, but are unable fully to comprehend the physical principles on which a technology relies (e.g. Penn & Povinelli Reference Penn and Povinelli2007). In contrast, current humans display a deep understanding of physical and psychological causal relations (e.g. Gopnik et al. Reference Gopnik, Glymour, Sobel, Schulz, Kushnir and Danks2004). Lombard and Gärdenfors (Reference Lombard and Gärdenfors2017) developed a seven-grade perspective on the evolution of causal cognition in humans (Table 1). This approach is based primarily on the degree to which the cause is perceivable, imaginable, purely abstract, and interchangeable between knowledge domains.
Table 1. The grades of causal cognition following Lombard and Gärdenfors (Reference Lombard and Gärdenfors2017, 3–6, and references therein). The graded model is not necessarily unilinear, so that some of the grades might have evolved parallel to each other.
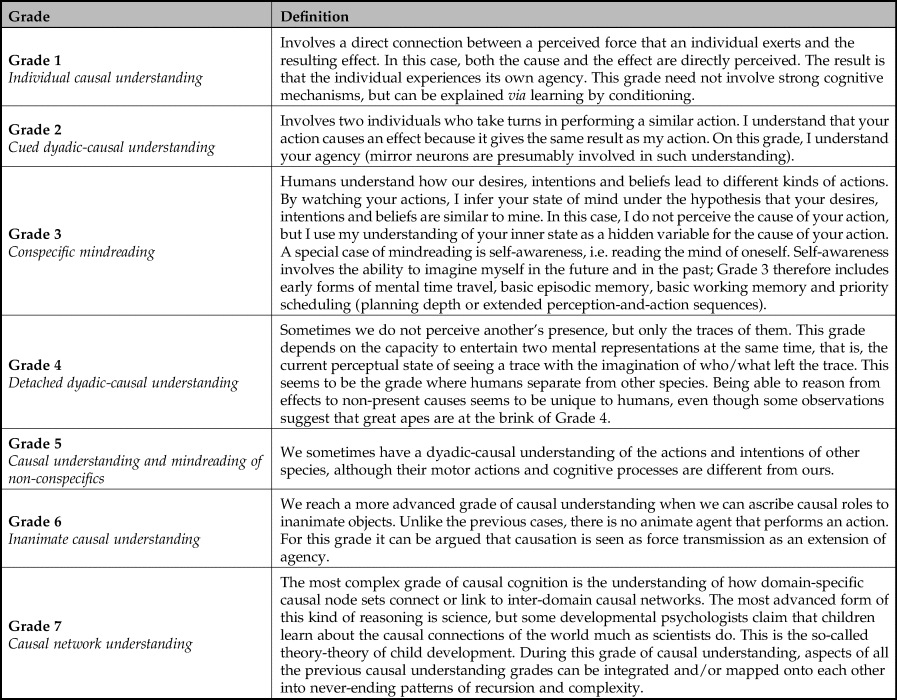
Variation in modes of teaching
Much of human cognition is stimulated through learning and teaching. Learning increases human knowledge and thereby stimulates what Haidle and colleagues (Reference Haidle, Bolus and Collard2015) termed ‘an expansion of human cultural capacity’, contributing to the uniquely constructed human socio-cognitive niche (Whiten & Erdal Reference Whiten and Erdal2012). It has been claimed that ‘something happened during the evolution of Homo sapiens that also made us Homo docens—the teaching animal’ (Gärdenfors & Högberg Reference Gärdenfors and Högberg2017, 188). It therefore follows that exploring types of, and variation in, learning and teaching behaviours throughout our evolutionary history is a useful endeavour.
Social learning is a significant adaptation throughout hominin prehistory. In social learning, no intention is required on the part of teachers. Adults or peers on which learners model their behaviour do not adjust their actions with the intention of enhancing learning (Gärdenfors & Högberg Reference Gärdenfors and Högberg2017). Typically, such learning happens by learners simply copying their models, through emulation or imitation (see Tomasello Reference Tomasello1999).
Intentional teaching, however, goes beyond social learning. The capacity to understand that somebody else does not know how to do something, or does not know relevant facts, and that interacting with that person will increase their understanding or knowledge, is central to intentional teaching. This requires mindreading (e.g. Gärdenfors Reference Gärdenfors, Liljenström and Århem2007), or theory of mind (e.g. Tomasello Reference Tomasello1999), which in this context means the sharing and representing of others’ mentalities. Even though non-intentional teaching exists in other species, intergenerational cultural transmission of concepts or facts by intentional teaching is unique to modern humans (Gärdenfors & Högberg Reference Gärdenfors and Högberg2017).
Gärdenfors and Högberg (Reference Gärdenfors and Högberg2017) define a variety of intentional teaching modes that require increasing capacities of mindreading and communication on the part of teachers and learners, interpreted from the archaeological record (Table 2). We here apply them for analysis of the 11 different techno-behaviours studied.
Table 2. Summary of teaching modes and their criteria (adapted from Gärdenfors & Högberg Reference Gärdenfors and Högberg2017, 196, table 1). Note that teaching can be one-on-one, but is largely embedded in cultural settings, and should be understood in a context of a many-to-many relationship, horizontally distributed within a generation and/or vertically between generations (d'Errico & Banks Reference d'Errico and Banks2015).
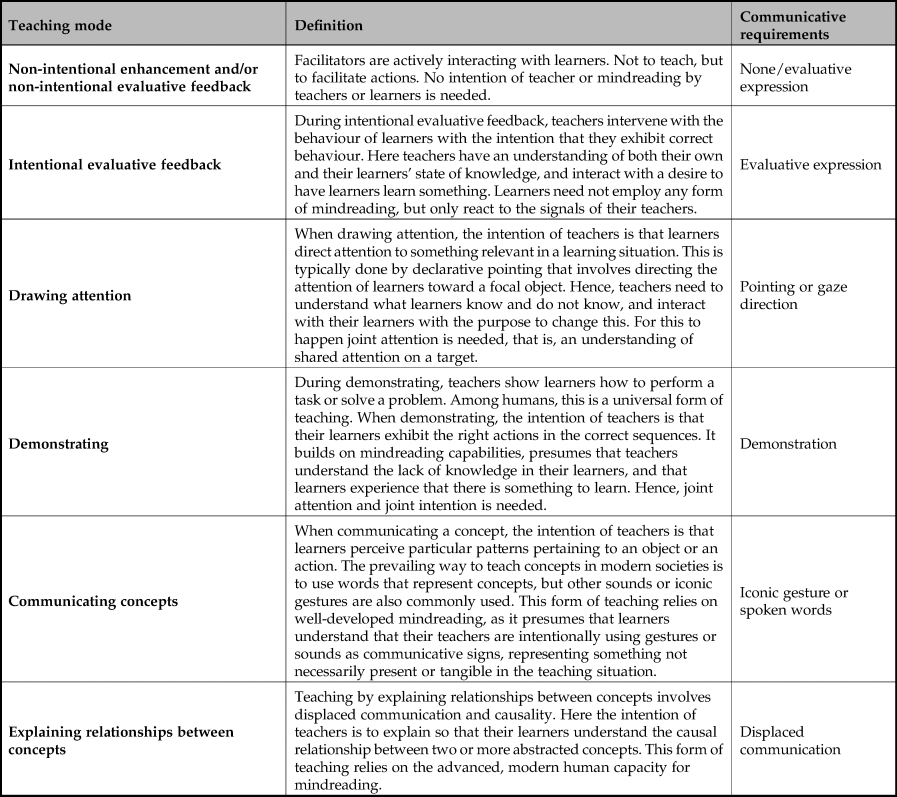
Thinking through capuchin rock pounding
Capuchin monkeys grasp hammerstones with both hands to pound on rocks lodged in alluvial deposits (Proffitt et al. Reference Proffitt, Luncz, Falótico, Ottoni, de la Torre and Haslam2016). The reason for the pounding remains unclear. One interpretation is that of aggressive display (Moura Reference Moura2007). Observation of capuchin monkeys sniffing and licking freshly pounded stones suggests that the pounding is likely to be a way to obtain mineral nutrients from the rocks (Proffitt et al. Reference Proffitt, Luncz, Falótico, Ottoni, de la Torre and Haslam2016). We included both alternatives in one cognigram, as the actions do not differ.
The pounding activity results in surfaces with crush marks on the stones and rocks. Sometimes it results in the accidental production of conchoidally fractured flakes, morphologically transforming the hand-held stones into pieces that resemble rough cores. The flakes and cores, however, are not used for secondary tasks (Proffitt et al. Reference Proffitt, Luncz, Falótico, Ottoni, de la Torre and Haslam2016). The pounding behaviour can be seen as a use of proto-tools wherein the lodged rocks are used as passive (not manipulated) anvils, and thus aids, to affect the stones pounded against them. Alternatively, it can be interpreted as the use of hand-held hammerstones as tools, used to affect the lodged rocks.
Capuchin rock pounding as proto-tool use
To perform the pounding activity, a monkey picks a stone and repeatedly hits a lodged rock (anvil). The anvil is not manipulated, but has an enhancing effect on the stone used for pounding it. No other strategies are needed. This task represents a single perception-and-action module and a single problem with two sub-problems, represented in three foci: the subject (need for display/nutrients), an object (the hand-held stone) and an aid (anvil) (Fig. 2). The subject represents the only active focus, whereas both the object and the aid remain passive. At its most complex, capuchin rock pounding, seen as proto-tool use, includes eight operational steps in five phases. An optional ninth step may include inspecting the resulting damage by sniffing or licking the hand-held stone. Once a stone is found, the only activity performed is recurrent pounding with no change in foci (Fig. 2). Sometimes the object is discarded, and another stone selected for a repeat performance.

Figure 2. Encoded perception-and-action sequences for capuchin rock pounding as proto-tool use, i.e., passive anvil use (stone on stone percussion). (Cognigram based on Proffitt et al. Reference Proffitt, Luncz, Falótico, Ottoni, de la Torre and Haslam2016).
In terms of expert cognition, the performance remains within a limited range of seemingly automatic responses. There is no evidence that capuchin monkeys learn this behaviour over an extended period or through extensive repetition, and no observations have been made to show that pounding accuracy varies between practicsed and non-practised monkeys. There is no evidence of rapid or in-depth problem assessment, and the action sequence is too short to include interruption without memory loss. No learning of new materials is associated with the activity.
Individual causal understanding (Grade 1) is represented by the monkeys’ perception that a stable, hard surface can be used as an aid to exert force on a hand-held stone, that such force will result in sound and/or damage to the stone, and that the damage can be observed by sniffing or licking stones used in this manner. Such understanding requires no complexity in cognitive processing. The monkey simply experiences its own agency, and learning takes place through individual conditioning. The fact that the pounding behaviour is observed amongst several monkeys in a group indicates that, at least partly, they have achieved cued dyadic-causal understanding (Grade 2), probably through mimicking each other's actions. We do not see any evidence of causal understanding beyond Grade 2.
The presence of basic Grade 2 causal understanding implies some social learning. There is no indication that capuchin monkeys teach each other to pound rocks, either non-intentionally or intentionally.
Capuchin rock pounding as tool use
The performance of capuchin rock pounding using a hammerstone is similar to that of a passive anvil as proto-tool use. The same number of modules (N = 1), problems (N = 1), sub-problems (N = 2), operational steps (N = 8 + 1) and phases (N = 5) are involved (Fig. 3). In this scenario, however, there is no passive aid. Instead, a tool (the hammerstone) is used to pound on the object (lodged rock/anvil) repeatedly with no other strategies involved. The task performed by the monkey again consists of three foci: the subject (need for display/nutrients), an object (anvil) and a tool. In this scenario there are two active foci (the subject and the tool) that are engaged simultaneously, with the tool actively affecting the object. Focus on the object remains passive. Repeating the performance represents no increase in the problem-solution distance even when one hammerstone is discarded for another. The only activity performed is recurrent pounding (Fig. 3).

Figure 3. Encoded perception-and-action sequence for capuchin rock pounding as tool use, i.e., the use of hammerstones (stone on stone percussion). (Cognigram based on Proffitt et al. Reference Proffitt, Luncz, Falótico, Ottoni, de la Torre and Haslam2016.)
We interpret the level of expert cognition for capuchin rock pounding as tool use the same as using a passive anvil as proto-tool. Although capuchin monkeys cracking nuts showed a skilful adjustment of their actions depending on the mass of the hammerstones (Liu et al. Reference Liu, Fragaszy and Visalberghi2016), this cannot be said for using a hammerstone to pound stones. There is no evidence that the behaviour is learned over an extended period or through extensive repetition, that pounding accuracy increases with practice, or that rapid or in-depth problem assessment is applied. The action sequence is too short to facilitate interruption without memory loss. No learning of new material is involved and responses remain automatic.
Causal understanding is the same as for the proto-tool scenario. The active use of a hammerstone, however, may represent the initial, limited stages of self-awareness (included in Grade 3 causal understanding), where the monkey senses that a hand-held tool can be used as an extension of its body to accomplish a task more effectively. Indeed, in an experimental setup Borgo and colleagues (Reference Borgo, Spagnoletti, Vieu and Visalberghi2013) showed that nut-cracking capuchin monkeys intentionally selected specific types of hammerstones. There is, however, no evidence that this understanding is accompanied by a perception of the self in the future or the past; neither is there any evidence of any of the other grades of causal understanding. Basic social learning is again implicated, but teaching is not necessary for this techno-behaviour.
Thinking through chimpanzee nut cracking
Wild apes have not been observed to perform intentional knapping (Whiten et al. Reference Whiten, McGuigan, Marshall-Pescini and Hopper2009). Numerous other forms of tool use have, however, been recorded for non-human primates (Shumaker et al. Reference Shumaker, Walkup and Beck2011). Here we use an example of chimpanzees cracking Panda oleosa nuts, using a hammerstone and an anvil (Carvalho et al. Reference Carvalho, Cunha, Sousa and Matsuzawa2008). Observations of wild chimpanzees performing nut cracking show variation between groups and reveal systems of stone-tool transport and rock selection (e.g. Arroyo et al. Reference Arroyo, Hirata, Matsuzawa and de la Torre2016). Still, the basic task is a repeated striking of a nut to crack the nutshell open, so that the seed within can be retrieved and consumed. We do not consider cracking nuts with a hammerstone and anvil to be the use of a ‘tool set’, since the anvil is a proto-tool or aid (see Haidle Reference Haidle2012; but see Carvalho et al. Reference Carvalho, Cunha, Sousa and Matsuzawa2008). The rare use of wedges to stabilize anvils for nut cracking is also not considered an example of a ‘composite’ technology (see Haidle et al. Reference Haidle, Bolus and Collard2015 for definition). The wedges simply serve as aids, sometimes used in situ and sometimes transported to a nut-cracking site, but never actively manipulated as tools (Haidle Reference Haidle2012).
For a chimpanzee to perform nut cracking, it must select suitable stones and transport them to, and place them in, the nut-cracking location (Mercader et al. Reference Mercader, Panger and Boesch2002). In an experimental setup, Carvalho and colleagues demonstrated that wild chimpanzees systematically selected tools, a hammerstone and/or an anvil, with individually preferred properties (Carvalho et al. Reference Carvalho, Cunha, Sousa and Matsuzawa2008). Excavation has revealed spatial layout arrangement of nut-cracking sites where chimpanzees repeatedly worked in areas focused around the same immobile anvils, but sometimes substituting hammerstones (Mercader et al. Reference Mercader, Panger and Boesch2002). It has been suggested that early hominins discovered the mechanisms of stone knapping as a result of flakes that unintentionally fractured from hammerstones or anvils used for pounding tasks (Marchant & McGrew Reference Marchant, McGrew, Rous and Bril2005). How such unintentional flaking was transformed into intentional knapping is not yet known, and wild chimpanzees have not been recorded to use such accidental flakes for any subsequent tasks.
We distinguish between two nut-cracking scenarios: 1) a minimal performance with nut cracking on a passive anvil surface, where nuts and hammerstone are brought to a naturally occurring hard surface for cracking. Here, none of the optional phases sometimes observed during chimpanzee nut cracking are included, representing the most basic form of this techno-behaviour; 2) a maximal scenario, with chimps manipulating the anvil using a wedge to stabilize/position it, representing the most complex techno-behaviour observed for nut-cracking chimpanzees.
Chimpanzee nut cracking—minimal
Performing the basic task of nut cracking, chimpanzees have to find a location with an anvil and collect nuts and a hammerstone. Once everything is assembled, they have to position a nut on the anvil and hammer it until the shell is cracked. They repeat the process for each nut during a non-modular performance. Our cognigram for the simplest nut-cracking scenario (Fig. 4) shows that it can be broken down into one problem (basic need for food) and three sub-problems (need of a food source: nuts, a tool: hammerstone and a technological aid: anvil). Thus, four foci are involved; two are active (i.e. the subject with its need for food, and the tool), and two remain passive (the object and the aid). Eleven operational steps in five phases are needed to complete the task, and if subsequent nuts are cracked during the same session, steps 5–11 are repeated for each nut. The only direct (active) effect is that of the hammer on the nut placed on the anvil.

Figure 4. Encoded perception-and-action sequence without optional phases (minimal) for chimpanzees using hammerstones aided by in situ anvils to crack Panda oleosa nuts. (Cognigram based on Carvalho et al. Reference Carvalho, Cunha, Sousa and Matsuzawa2008.)
Considering expert cognition, success is only achieved through tedious repetition. Novice nut crackers require up to four years to master the activity—if at all (Boesch Reference Boesch1991). Expert nut crackers are more accurate than novices. To perform the task well, a chimpanzee must develop a good understanding of how to position nuts on the anvil so that the hammer blow becomes successful. It must also move the hammer-holding arm in accordance with how the nut is positioned (Visalberghi et al. Reference Visalberghi, Sirianni, Fragaszy and Boesch2015). Such observations indicate the ability to assess a problem rapidly, but no in-depth problem assessment is required. The action sequence is too short for interruption without memory loss. The hammering performance remains limited to a narrow activity domain. For example, chimpanzees have never been observed using their nut-cracking skills to retrieve other resources, so that there is no learning of new materials. Once the task is mastered, the chimpanzees execute it mostly automatically with little active attention.
Individual causal understanding is a requirement. Cued dyadic-causal understanding is evident in the fact that young chimpanzees seem to understand that by mimicking expert crackers, they too might be able to access the food source. Conspecific mindreading is represented at a basic level on the rare occasions when a mother helps her infant to improve its hammer grasp (Boesch Reference Boesch1991). Using the hammerstone also implies limited self-awareness, a trait associated with this level of causal reasoning. Transporting of nuts and/or hammerstones to the anvils indicates basic, short-term planning skills—awareness of the self in the immediate future. There is no evidence of causal understanding beyond the basic stages of Grade 3.
The aptitude for enhancing another's performance and for non-intentional evaluative feedback can be assumed for nut-cracking chimpanzee mothers who assist their infants by correcting their hammer grasps. They are therefore capable of evaluative expression. Whereas joint attention is required, the teaching in this scenario still lacks the joint intention required for drawing attention to or demonstration of an action sequence. There is no communicating of concepts or relational explanations.
Chimpanzee nut cracking—maximal
Here we assume that hammerstones and anvils are transported to the food source, i.e. the nut tree, and that a wedge is collected and used to position/stabilize the anvil. The cracking follows the same sequence as described above (phase IV in Fig. 4 and phase X in Fig. 5). The performance is non-modular with one problem, but can have up to four sub-problems, and the problem-solution distance is extended to 11 phases. With maximal nut cracking, the chimpanzees use one tool (hammerstone), but employ up to two technological aids (anvil and wedge). The wedge enhances the position of the anvil, and the anvil enhances the effect of the hammerstone in cracking the nut. The process requires five foci: two are active (subject and tool) and three remain passive (two aids and object). Up to 20 operational steps are facilitated in the 11 phases.

Figure 5. Encoded perception-and-action sequence with optional phases (maximal) for chimpanzees using hammerstones, aided by collected anvils and wedges, to crack Panda oleosa nuts. (Cognigram based on Carvalho et al. Reference Carvalho, Cunha, Sousa and Matsuzawa2008.)
Expert cognition is very similar to the minimal scenario. It requires several years of practice, success depends on incessant repetition and expert nut crackers are more accurate than novices. In the maximal scenario, however, the expert chimpanzee not only understands how to position nuts on the anvil effectively and to move its arm accordingly, but also understands how to position and stabilize the anvil. The latter can be seen as some depth (although limited) in problem assessment. The action sequence is too short for interruption without memory loss. There is no evidence for learning new material, the performance remains limited to nut cracking, and experts execute their actions automatically, with little or no active attention.
The same grades of causal understanding are required as for the minimal scenario. Even the use of wedges as passive stabilizing aids can be explained through the experience of own agency and via learning by conditioning, i.e. Grade 1 causal understanding, and if imitated, it would imply cued dyadic-causal understanding (Grade 2). Using the hammerstone can be seen as a limited form of self-awareness. Planning skills are slightly extended, represented by the inclusion of the wedge option, but there is no evidence of causal understanding beyond some basic stages of Grade 3 self-awareness.
Because no teaching has yet been recorded in association with chimpanzees using wedges to stabilisze anvils for nut cracking, the teaching skills for this techno-behaviour are assumed to be the same as described for minimal nut cracking.
Thinking through Lomekwi 3 passive hammer flake production
The Lomekwian knappers used hefty cobbles and nodules to produce irregular flakes from asymmetrical cores (Harmand et al. Reference Harmand, Lewis and Feibel2015; Lewis & Harmand Reference Lewis and Harmand2016). These flakes were detached from a single face of the core and not modified or retouched. Knappers seem to have combined ‘core reduction and battering activities and may have used artefacts variously: as anvils, cores to produce flakes, and/or as pounding tools’ (Harmand et al. Reference Harmand, Lewis and Feibel2015, 313). Although it is not known what the flakes were used for, possible cut marks reported on bones from the 3.39-million-year-old site at Dikika, Ethiopia, allude to the ancient use of sharp flakes as cutting tools (McPherron et al. Reference McPherron and Alemseged2010). Here we accept that flakes were used as cutting tools to assist early hominins in scavenged meat procurement and consumption.
Flake use
Hominin flake production and the subsequent use of the flakes might imply technological modularity. To avoid repetition and the inflation of cognitive interpretation, we encode flake use as a discrete module, especially since naturally occurring sharp rock slivers or accidentally produced flakes might have been used as tools. Flake use to procure/process meat represents the problem of hunger, with two sub-problems (need of meat and need of cutting tool), encoded in three foci. During flake use, two foci are active: the subject with its need to satisfy hunger and the activated flake tool. The object (carcass) remains passive. The performance is facilitated in six phases with eight operational steps. The flake directly affects the carcass during use (Fig. 6).

Figure 6. Encoded perception-and-action sequence for the independent use of a natural or intentionally produced flake as a tool.
Novice meat cutters probably did not require much repetition to master the task. Once thumbs and fingers were capable of a tight grip (e.g. Key & Dunmore Reference Key and Dunmore2015), cutting meat with a stone sliver requires little practice. The accuracy of cutting or butchery activities with flakes, however, probably increased with practice. We suggest that problem assessment during the activity is rapid, but not in depth. The cutting actions are too short to be affected by memory loss during interruption. If only meat was cut, there is no rapid learning of new material, whereas cutting a range of materials would imply learning of new materials. The same applies to the expert cognition criterion of activity narrowness. In the case of early hominins, direct evidence for such behaviours remains speculative at best, and our stance is to revert to the simplest possible interpretations. In this instance, it would exclude the rapid learning of new materials and suggest limitation to narrow domains of activity, accompanied by experts responding automatically and with little active attention once they mastered cutting meat with a flake.
For hominins to use flakes effectively as cutting tools, Grade 1 causal understanding is represented by their perception that a carcass becomes more accessible by cutting it into portions. For flake use to become a standard practice in our genus, Grade 2 causal understanding had to be well developed. Thus, we can assume that some hominin groups quickly grasped that the action of another causes an effect that they could emulate by performing the same action. They were therefore able to understand the agency of conspecifics. Further, when one individual observes the use of a cutting tool by another to satisfy hunger, and proceeds to replicate the behaviour, a basic notion of another's intentions is reflected (i.e. basic shared attention/intention) as described for Grade 3 causal understanding. Flake use also represents limited self-awareness, and if flakes were transported, short-term planning, i.e. the perception of self in the immediate future, is implied. These are both aspects of Grade 3 causal understanding, but do not signal any sophistication in such reasoning.
Whereas social learning is inherent in the use of stone tools across generations, teaching associated with using flakes to cut is less obvious. Non-intentional teaching through enhancement could, however, have been involved. An example would be one individual providing another with access to a flake when needed. No mindreading is necessary, but empathy would help. Such provision behaviour could help explain the increasing need, production and use of stone tools throughout the Earlier Stone Age. However, because the performance can be mastered through copying, possibly expedited through non-intentional teaching, no intentional teaching needs to be involved.
The Lomekwi 3 passive hammer technique
The passive hammer technique is an example of proto-tool use during which knappers remove flakes by directly acting on the nodule as the object, striking it on an appropriate aid (location/anvil), normally a stationary rock or large movable stone (Pargeter & Duke Reference Pargeter and Duke2015). Using this technique, the Lomekwian knappers needed to select a nodule (intended as core) and a suitable anvil. They would then hold the nodule/core in both hands and bash it against the anvil. The blow must be positioned appropriately to facilitate flake detachment upon impact.
Flake production with the passive hammer technique consists of a single problem and three sub-problems, represented in one active and three passive foci (Fig. 7). During production, the active subject focus is the need for a cutting tool; both the nodule and the subsequent flake remain passive objects, as does the anvil serving as proto-tool. The perception-and-action sequence is completed in 13 operational steps, grouped in six phases. There is no direct effect of an active focus on a passive object; instead, the anvil has an effect on the nodule to produce what will be used later as an active tool. The activity of hitting the core against the anvil is repeated (operational steps 4–11 in Fig. 7), while the core might be slightly repositioned in the hands of the knapper for each hit. Variations in the operational steps are therefore discrete, represented by flake selection and the potential repositioning of the nodule/core.

Figure 7. Encoded perception-and-action sequence for flake production with the passive-hammer technique at Lomekwi 3. (Cognigram based on Harmand et al. Reference Harmand, Lewis and Feibel2015 and Lewis & Harmand Reference Lewis and Harmand2016.)
It takes some time and repetition to become an expert in passive hammer knapping (e.g. Stout et al. Reference Stout, Hecht, Khreisheh, Bradley and Chaminade2015). Once expertise has been reached, knappers would probably be able to perform with relative accuracy and reliability. Problem assessment for expert passive hammer knappers would be rapid (e.g. a quick assessment of the next best angle from which to produce a flake), but the activity does not require any in-depth problem assessment and is too short-lived to be hampered by memory loss when interrupted. Limited learning of new material is involved, as only stone is knapped in this manner, perhaps using different rock types. The performance is therefore limited to a narrow domain of activity and expert responses will automatically facilitate the small variations needed to complete the task successfully, such as dealing with variation in the natural shape and angles of nodules.
Causal cognition for passive hammer flake production can be facilitated within the first two grades. Assembling nodules and anvils indicates limited awareness of the self in the immediate future—touching on Grade 3 causal understanding. Whereas social learning is implied by the fact that flake production became a pan-Homo techno-behaviour, we do not know whether any form of teaching was involved in early passive hammer flaking. The technique is easy to imitate—through trial and error a novice will eventually succeed. No strategic judgements about planned actions are necessary (see discussion in Stout et al. Reference Stout, Hecht, Khreisheh, Bradley and Chaminade2015). Though teaching would have accelerated and improved the learning process, it was probably not essential for this techno-behaviour.
Passive hammer and flake use—non-modular
The intentional production of flakes is bound to their use as cutting tools. This link can be reconstructed in a non-modular and a modular way. In the non-modular way, the subject perceives hunger and the subsequent need of a cutting tool and actions necessary to produce it as a single sequence. The production and use processes are the same as described for the separate perception-and-action sequences (Figs. 6 & 7), but the combination of activities results in an extended time line and/or problem-solution distance. The basic problem is to satisfy hunger, addressed by solving four sub-problems (Fig. 8), i.e. finding a carcass, finding a nodule, finding an anvil and producing a flake for cutting meat. The need to satisfy hunger and the cutting tool both require active attention/foci, whereas the anvil, nodule and carcass remain passive. Nineteen operational steps are executed in seven phases to complete the task, with the anvil affecting the nodule passively and the flake actively affecting the carcass. Multiple flakes can be produced by repeating some of the operational steps (steps 4–11 in Fig. 8).

Figure 8. Encoded perception-and-action sequence for flake production with the passive-hammer technique at Lomekwi 3 and immediate subsequent flake use—non-modular sequence.
Expert cognition is mostly the same as described for flake production using the passive hammer technique only, but we see some combination with another field of expertise when a stone knapper switches activities and starts cutting meat, representing two different activity domains. This indicates that the performance is less narrow, but expert execution can still be accomplished automatically with little or no active attention. With regard to causality, we see a small increase in planning depth, i.e. self-awareness in time. The modes of teaching remain the same as inferred for flake use and the passive hammer technique performed separately.
Passive hammer and flake use—modular
The modular way of linking the processes of production and use of flakes assumes a decoupling of the intermediate target of having a tool from the satisfaction of the basic need with this tool. Flake production is not only perceived as part of the perception-and-action sequence to fulfil the satisfaction of the current need ‘hunger’, but can be trained and executed separately. The shift from non-modular to modular is probably slight. However, once understood and increasingly performed, this shift could have eased learning as well as the application of parts of the sequence as modules in other contexts.
In its simplest manifestation, the individual is focused first on tool production and then on its use to fulfil a need. This represents a hierarchical staggering of perception-and-action sequences where more than one problem is addressed in separate, but subsequently linked performances. The time dimension becomes more pronounced, because the one action is further separated in time from the other, compared to the previous scenario. There are two distinct problems, the first resolved through three sub-problem solutions and the second by two (Fig. 9). Cumulatively, 21 (13 + 8) operational steps are needed to complete the two performances during which three active (1 + 2) and four (3 + 1) passive foci are used. The series of operational steps are facilitated in 11 phases.

Figure 9. Encoded perception-and-action sequence for flake production with the passive-hammer technique at Lomekwi 3 and future intended flake use—modular sequence.
Not much practice is needed to develop the skill of cutting meat with a flake, but the passive hammer knapping technique probably requires some time and practice to master. In both tasks, accuracy and reliability will increase as expertise develops. Experts will make rapid assessments of their sub-problem sets, and the depth of these assessments decreases slightly with a reduced time dimension for each of the tasks when performed separately. Yet the overall time depth of the whole process may increase markedly. Performers can probably be interrupted between the two tasks with little or no loss of information, i.e. the two memory chunks (how to produce a flake and how to use a cutting tool) can easily be linked into a chain of actions. Because tool production is decoupled here from the need to cut flesh to still the basic need of ‘hunger’, the learning of new material can also include the application of the tools in a cutting action on other items than flesh. Once mastered, expert responses are automatic, performed with little active attention.
Again, we see an increase in self-awareness through time when flakes are produced and used in a modular manner. This aspect of Grade 3 causal understanding therefore seems to undergo continuous expansion in small increments, whereas the other aspects of this grade (including the understanding of another's mind) do not require much development to perform the two tasks in series. There is no need for teaching, but social learning is apparent in the continuation of the behaviours through time. Although the bits to learn become smaller in the modular way, the link between production and tool use is probably not as evident as in the non-modular way and may require an additional learning process.
Thinking through the Lomekwi 3 bipolar flake production
At Lomekwi 3, the knappers also used a bipolar technique (Harmand et al. Reference Harmand, Lewis and Feibel2015; Lewis & Harmand Reference Lewis and Harmand2016). With this technique, the nodule/core is placed on an anvil, and the blow delivered with a hammerstone impacts vertically. On impact, the distal end of the core is compressed against the anvil, resulting in flake detachment from the core, both on the part struck by the hammerstone and the part compressed against the anvil (Pargeter & Duke Reference Pargeter and Duke2015).
Although technological attributes show that the knapping actions were poorly controlled (Harmand et al. Reference Harmand, Lewis and Feibel2015), to detach series of adjacent flakes repeatedly, the Lomekwi 3 knappers must have positioned the core with one hand simultaneously with hitting it with the hammerstone (Harmand et al. Reference Harmand, Lewis and Feibel2015; also see Lewis & Harmand Reference Lewis and Harmand2016). Shott and Tostevin (Reference Shott and Tostevin2015, 378) point out that this requires control of three objects (not to be confused with our use of the word ‘object’ in the cognigrams): ‘the hand holding the core (object 1) can vary the fracture mechanics by adjusting the orientation of the core relative to the anvil (object 2) while the percussion hand […] deliver[s] the blow from the hard hammer (object 3)’. Thus, knappers must learn to move their hammer-wielding arm in accordance with how a core is positioned, and to apply the correct amount of force. They must also learn good holding positions for the core. It is therefore crucial for knappers to understand platform orientation and to be able to coordinate the specific bimanual aspects of bipolar knapping (see Bril et al. Reference Bril, Parry and Dietrich2015).
Lomekwi 3 bipolar technique
The Lomekwian bipolar technique is an example of tool use with a single problem (the need to cut), addressed in the solution of four sub-problems (needs of hammerstone, anvil, raw material to knap and cutting tool) (Fig. 10). These problems are represented by two active foci (the subject and the hammerstone/tool) and three passive foci (anvil/proto-tool/aid, nodule/passive object and resulting flake). We now see the introduction of two sub-foci directed towards the passive focus on the nodule (object 2 in the cognigram and sub-problem 2 in the description: Fig. 10). These represent an awareness of the platform angle to be struck (0b1 in Fig. 10) and of controlling the front of the nodule for successful flake detachment (0b2 in Fig. 10). The performance consists of 18 operational steps facilitated in eight phases. The anvil has a passive effect on the active effect that the hammerstone has on the nodule.
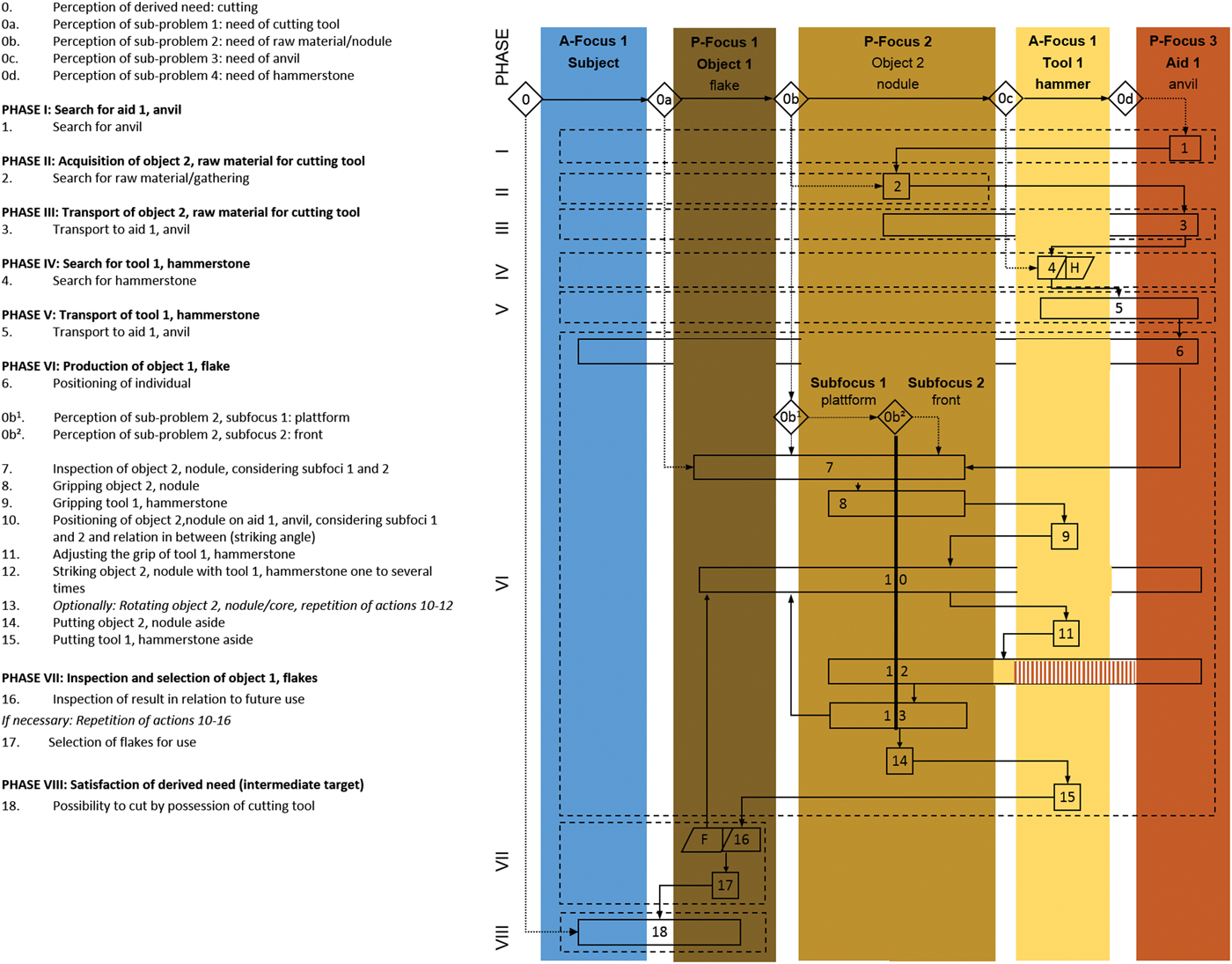
Figure 10. Encoded perception-and-action sequence for flake production with the bipolar technique at Lomekwi 3. (Cognigram based on Harmand et al. Reference Harmand, Lewis and Feibel2015 and Lewis & Harmand Reference Lewis and Harmand2016.)
Novices require time and practice to master this knapping technique and it takes expertise to perform with accuracy and reliability. Experts would be able to make rapid assessments of problems associated with raw material, and the depth of problem assessment is increased by the necessity to achieve the correct striking angle, core face aspect and force. Because the performance is short-lived and continuous, we do not see evidence of experts being able to retain memory during interruption. ‘New’ material might be represented by variation in rock type/quality. The performance is thus limited to a narrow domain of activity, and responses to problems become automatic with expertise.
Grades 1 and 2 causal understanding are necessary for bipolar knapping. Some (probably more than very basic) self-awareness (Grade 3 causal understanding) is necessary for the bimanual manipulation of objects and for assessing the correct amount of striking force. Duke and Pargeter (Reference Duke and Pargeter2015) demonstrated that this basic knapping technique requires skill to perform, and that it is not possible to master without being taught by an experienced knapper. This observation implies some mindreading of others, during which some attention and intention is shared in the context of Grade 3 causal understanding (conspecific mindreading).
Based on experimental observations (Duke & Pargeter Reference Duke and Pargeter2015), we assume that at least the non-intentional teaching modes of enhancement and evaluative feedback were in play, as well as a level of intentional evaluative feedback. Finley (Reference Finley2008) suggested that the position of the hand holding a bipolar core might obscure a direct view of the reduction technique from an observer. This would make the bipolar technique difficult to imitate accurately. Hence, we suggest that this technique probably required a teacher to draw attention to the different objects and their aspects that have to be controlled. This denotes joint attention and, at a minimum, necessitates pointing and/or gaze directing to communicate intention.
Bipolar knapping and flake use—non-modular
When bipolar flakes are produced in a non-modular sequence starting with the subject's perception of ‘hunger’ and its fulfilment as final target, it represents a single problem that is solved in five sub-problems (Fig. 11). Three active foci are in play (subject, hammerstone, flake), and three passive foci (nodule, anvil, carcass), but one of the passive foci requires attention on two sub-foci. For the activity to be completed, 24 operational steps take place in 10 phases, resulting in a slightly extended perception-and-action sequence compared to bipolar knapping only. Here two separate tools are actively used to achieve the ultimate goal of cutting meat, with the anvil as a passive aid.
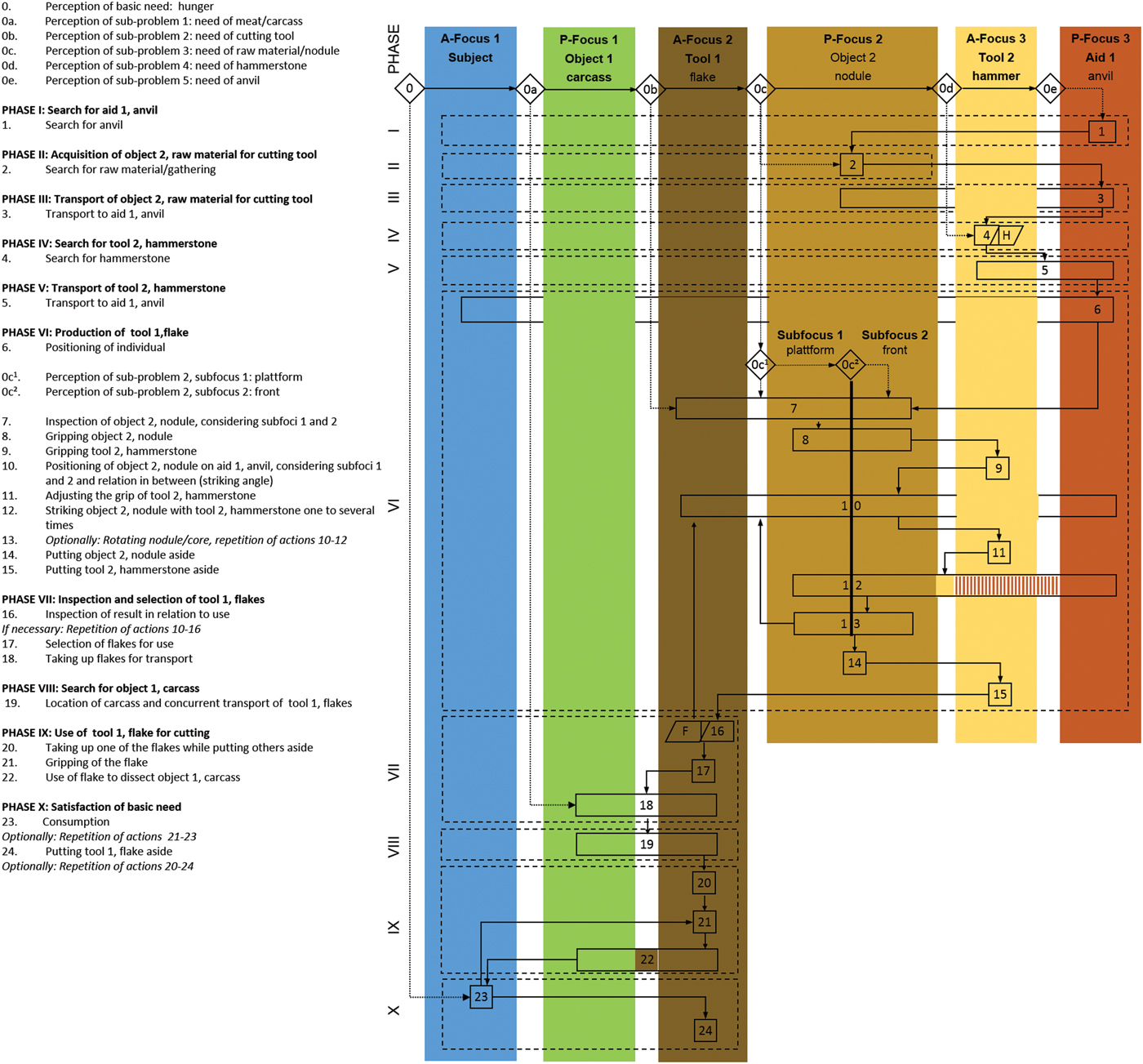
Figure 11. Encoded perception-and-action sequence for flake production with the bipolar technique at Lomekwi 3 and immediate subsequent flake use—non-modular sequence.
Expert cognition is the same as for the bipolar knapping performance alone, but having to process both stone and meat in a single sequence expands the learned material and range of activities slightly. Causal cognition requirements remain within the first three grades, with only a small increase in planning depth. No additional teaching modes are necessary compared with what we have suggested for bipolar knapping.
Bipolar knapping and flake use—modular
When bipolar flakes are produced and transported to a carcass, or used at a later stage, the perception-and-action sequence becomes bi-modular. Here we see the need to resolve two problem sets through their respective sub-problem solutions, four sub-problems for the knapping and two for the cutting, represented by their respective active (N = 2 + 2) and passive (N = 3 + 1) foci, including the two sub-foci (Fig. 12). The process requires the execution of 26 (18 + 8) operational steps in 13 (8 + 5) phases, and the active manipulation of two different tool types, one produced before it could be used.
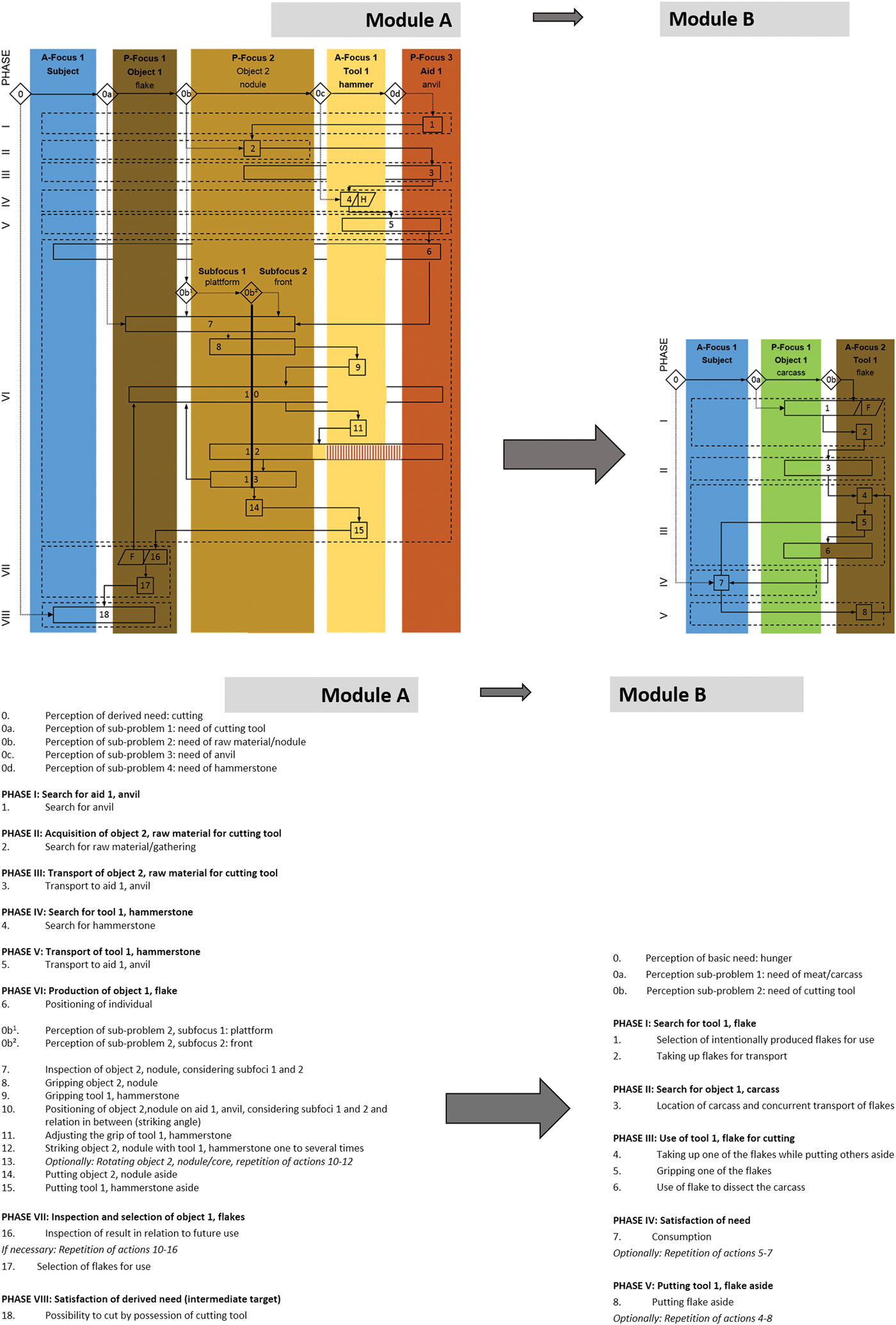
Figure 12. Encoded perception-and-action sequence for flake production with the bipolar technique at Lomekwi 3 and intended future flake use—modular sequence.
Apart from an increase in time and planning depth, which translates as an increase in the awareness of the self through time, causal understanding, levels of expert cognition and modes of teaching are the same as described for the bipolar knapping performance only. Although the bits to learn become smaller in the modular way, the link between production and use of the tool is probably not as evident as in the non-modular way and may require an additional learning process. However, this can be mastered with the modes of teaching mentioned in the non-modular variant. Thus, to summarize, if/when bipolar flakes produced at Lomekwi 3 were transported and/or used some time after their production, we suggest that the hominins that did so had the necessary thinking/cognitive abilities that enabled them to:
• Repeat actions to achieve success and to perform with increased accuracy;
• Make rapid problem assessments with some depth (but not in-depth);
• Probably be interrupted during short action chains (bi-modular) without the loss of information;
• Learn some (although limited) new materials;
• Deal with two activity domains in a single/bi-modular sequence;
• Automatically respond to variation in their activity sets without requiring much active attention;
• Perceive a direct connection between themselves and the effect that their force exerts;
• Understand that the actions of others cause effects that can be mirrored by acting in the same manner;
• Have adequate self-awareness to understand that a tool could be used to increase the body's ability for problem solution and have a basic awareness of the self in the immediate future, and some understanding of the desires and beliefs of their conspecifics;
• Learn socially through emulation and imitation;
• Teach non-intentionally through enhancement and non-evaluative feedback;
• Teach intentionally through evaluative feedback by correcting the behaviour of learners and through evaluative expression;
• Probably teach through drawing and sharing attention through pointing or gaze direction.
Comparative discussion
Thus far, our analyses have been group-specific, yet it is key to understand that our approach is applicable across species and contexts. Below, we therefore compare the techno-behaviours directly without always referring to the groups that performed them.
Whilst variation in cognigrams (Table 3) alone cannot be seen as a direct ‘measure of cognition’ (Haidle Reference Haidle2014), it does inform on some aspects related to cognition: a) the ability of the mind to focus simultaneously on the manipulation of a number of items (item-attention range); b) problem-solution distance (e.g. Lombard & Haidle Reference Lombard and Haidle2012); and c) the maximum attention span required to complete the longest phase within each techno-behaviour. Variability between some of the elements and their relationships can thus be used to hypothesize about factors that influenced the evolutionary trajectories of some techno-behaviours.
Table 3. Number of units presented in the cognigrams based on our interpretation of the perception-and-action sequences associated with each techno-behaviour.
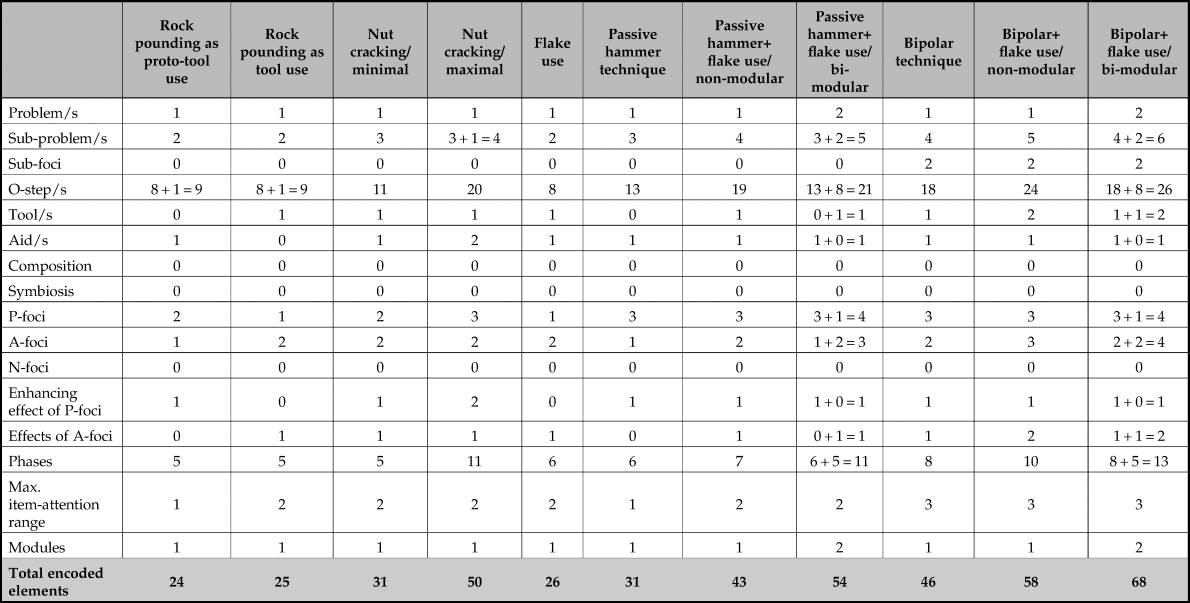
The ability to focus simultaneously on the manipulation of a number of items (Fig. 13) can be interpreted as the maximum item-attention range (the range of items that a subject holds in mind) at one time for a certain performance. In rock pounding as proto-tool use and passive hammer knapping, the subject only focuses on the object, the stone to be pounded or flaked; once chosen, the aid is not taken into account specifically. Assuming that capuchins use hammerstones as tools, we can argue that they keep an eye on the consequences of such use. Even if they do not actively control the object to be affected, they are aware of the tool and the object at the same time.
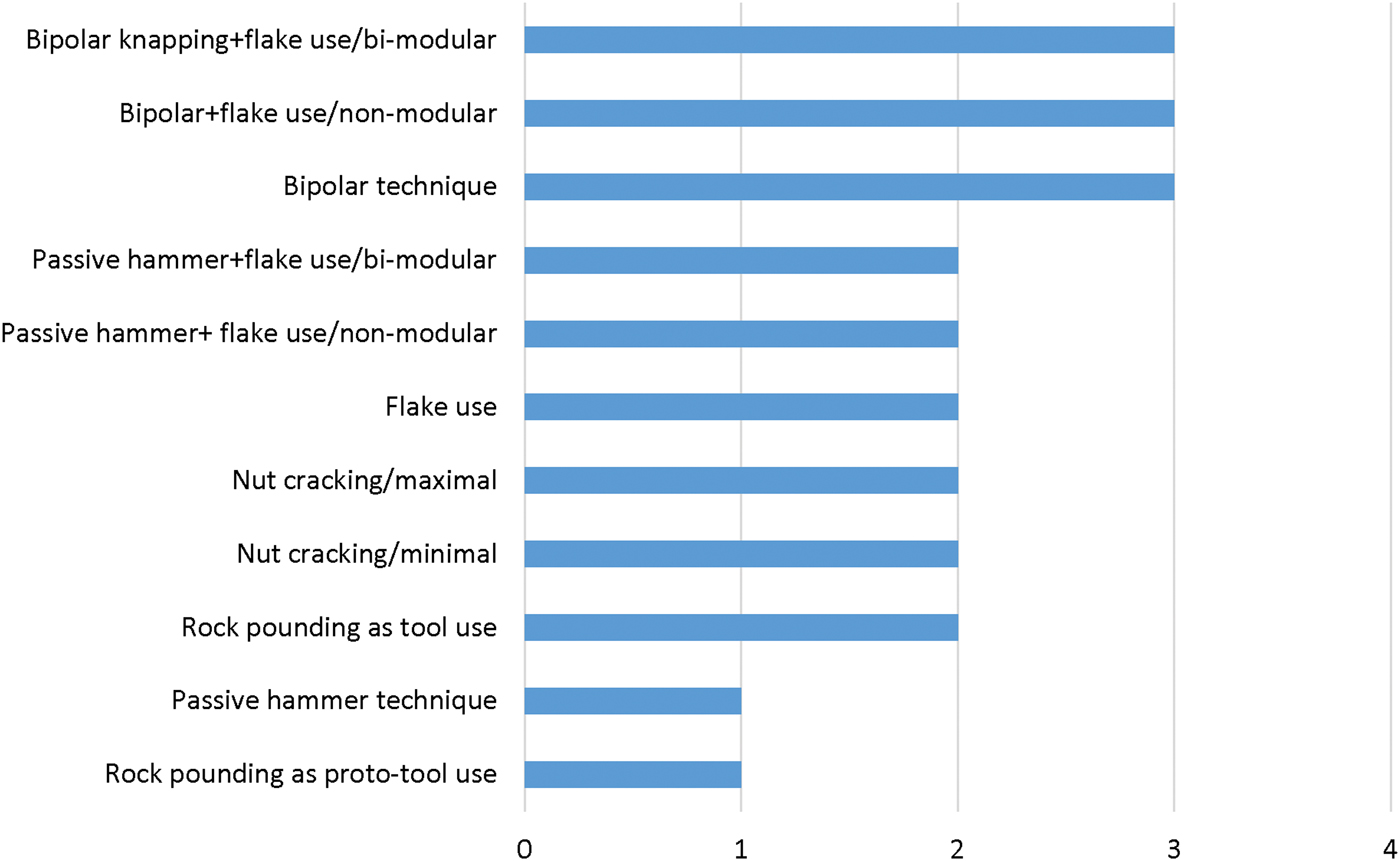
Figure 13. Ranking of the maximum item-attention range (range of items a subject can hold in mind at once during each of the performances in our analysis) based on our interpretation of the perception-and-action sequences associated with each techno-behaviour.
During minimal nut cracking, subjects control nut and hammer one after the other, but the attention is simultaneously on both. When wedges are used to adjust the anvil, subjects first focus on these two objects, and in the subsequent nut-cracking sequence, the attention is centred on nut and hammerstone. The use of a flake to cut something encompasses the manipulation of the tool and the awareness on where to cut. Thus, in cutting processes two things are simultaneously in focus.
The combination of passive hammer knapping and the use of the resulting flake to cut represents a sequence of: a) knapping with focus on one item only, and b) using the resulting flake to cut with the awareness of two items. The sequencing in non-modular as well as in bi-modular performance is comparable to adjusting the anvil with a wedge followed by nut cracking.
A special case is present in bipolar knapping where the focus is on two items, which are manipulated simultaneously. The nodule has to be monitored regarding both platform and front as sub-foci, while the hammerstone has to be controlled in adequately striking the platform (Figs. 11 & 12). In addition to an extension of the item-attention range up to three variables (nodule platform, nodule front, hammerstone), two hands manipulating different things at the same time increase the motor control. The combination of bipolar knapping and subsequent flake use represents a sequence similar to those in the maximum nut-cracking performance, and in passive hammer knapping followed by flake use. We therefore suggest that, once established, the extension of the item-attention range (as in the bipolar knapping technique) supported different kinds of tool performances, with training effects and mutational enhancement gradually impacting on cognitive evolution.
Problem-solution distance, or the ability to plan, can be interpreted as the aptitude to chunk and chain memory units without the loss of information. When we compare the numbers of problems, sub-problems, operational steps and phases (Table 3; Fig. 14), we see a direct correlation between perceived sub-problems and operational steps, i.e., the greater the number of sub-problems, the greater the number of operational steps required to complete the sequence. By arranging the 11 techno-behaviours in our sample from the smallest to the largest number of sub-problems and operational steps in our interpretation, variations between the problem-solution distances are revealed (Fig. 14). Flake use has the shortest distance, followed by pounding with proto-tools, pounding with tools and minimal nut cracking. Passive hammer knapping, bipolar knapping and the non-modular passive hammer + flake use scenarios all have shorter problem-solution distances compared to maximal nut cracking. Bi-modular passive hammer + flake use, non-modular bipolar knapping + flake use and bi-modular bipolar knapping + flake use respectively represent the most extended problem-solution distances.
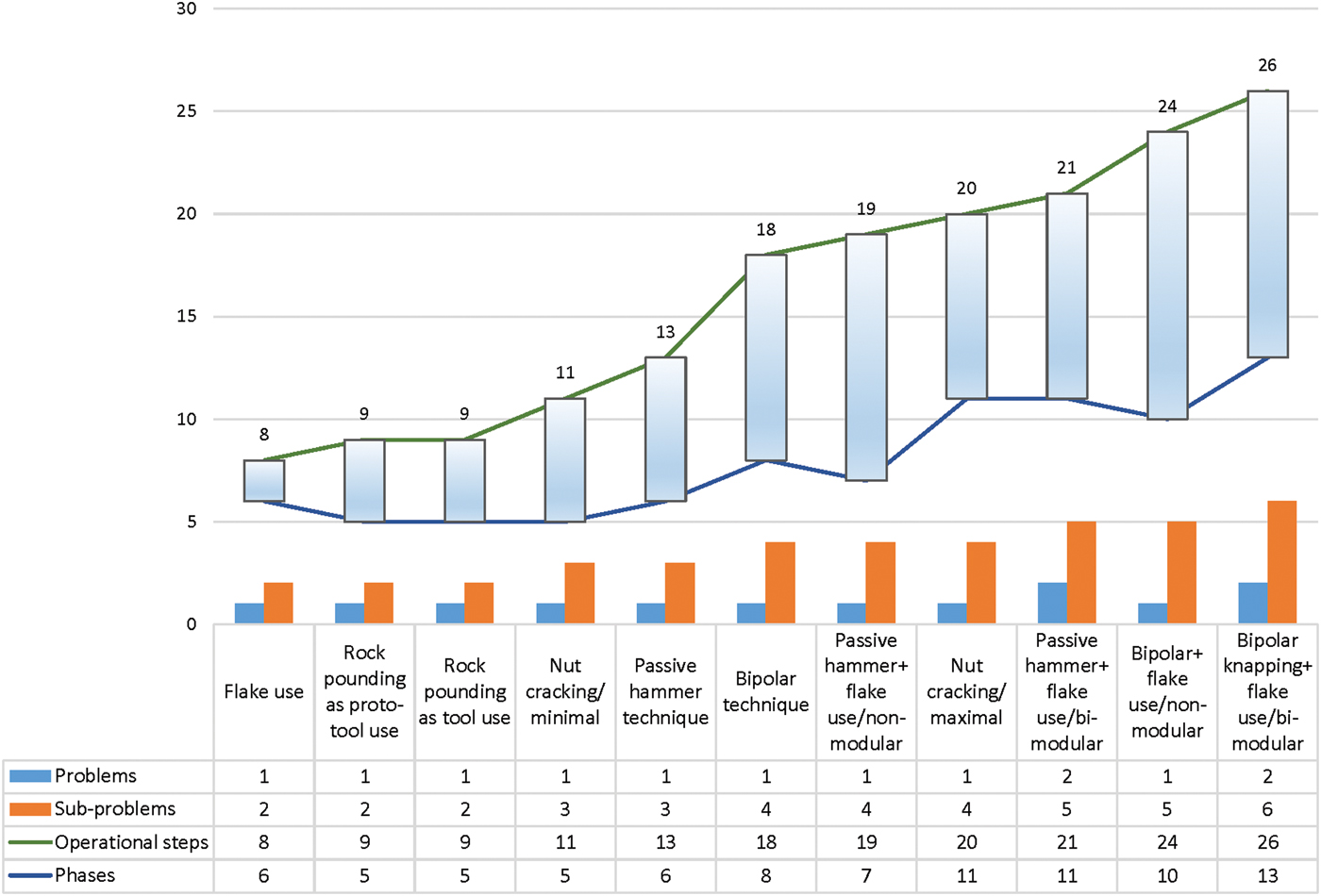
Figure 14. Number of sub-problems and operational steps arranged from smallest to largest (based on our interpretation of the perception-and-action sequences associated with each techno-behaviour) to assess variation in problem-solution distance.
Thus, it would appear that the Lomekwian knappers were able to handle longer problem-solution distances compared to pounding capuchin monkeys and nut-cracking chimpanzees (Fig. 14). Chimpanzees using wedges to stabilize anvils within their nut-cracking performance, however, are able to ‘out-plan’ some of the Lomekwian knapping performances. Based on our interpretation, we suggest that evidence for such behaviour in the hominin past may be able to contribute to discussions about their cognitive evolution.
Within any given phase of a techno-behaviour, clusters of actions have to be executed as a unit, or when interrupted, started again with the first action of the phase. Our hypothesis is that the most extended phase (with the most operational steps) performed within a techno-behaviour reflects the maximum attention span for each techno-behaviour (Fig. 15). For example, in the case of capuchin rock pounding as tool use (Fig. 3), phase IV with five operational steps represents the techno-behaviour's most extended phase—and therefore the maximum attention span for this behaviour. On the other hand, during all the bipolar performances (Figs. 10–12), we see that phase VI with 10 operational steps represents the most extended phase. Based on this interpretation, the passive hammer behaviours do not require greater attention spans than the two nut-cracking scenarios. The bipolar knapping performances, on the other hand, all require relatively long attention spans to complete the tasks successfully. We suggest that this might reflect a pull factor for growing attention spans in primate techno-behaviours.
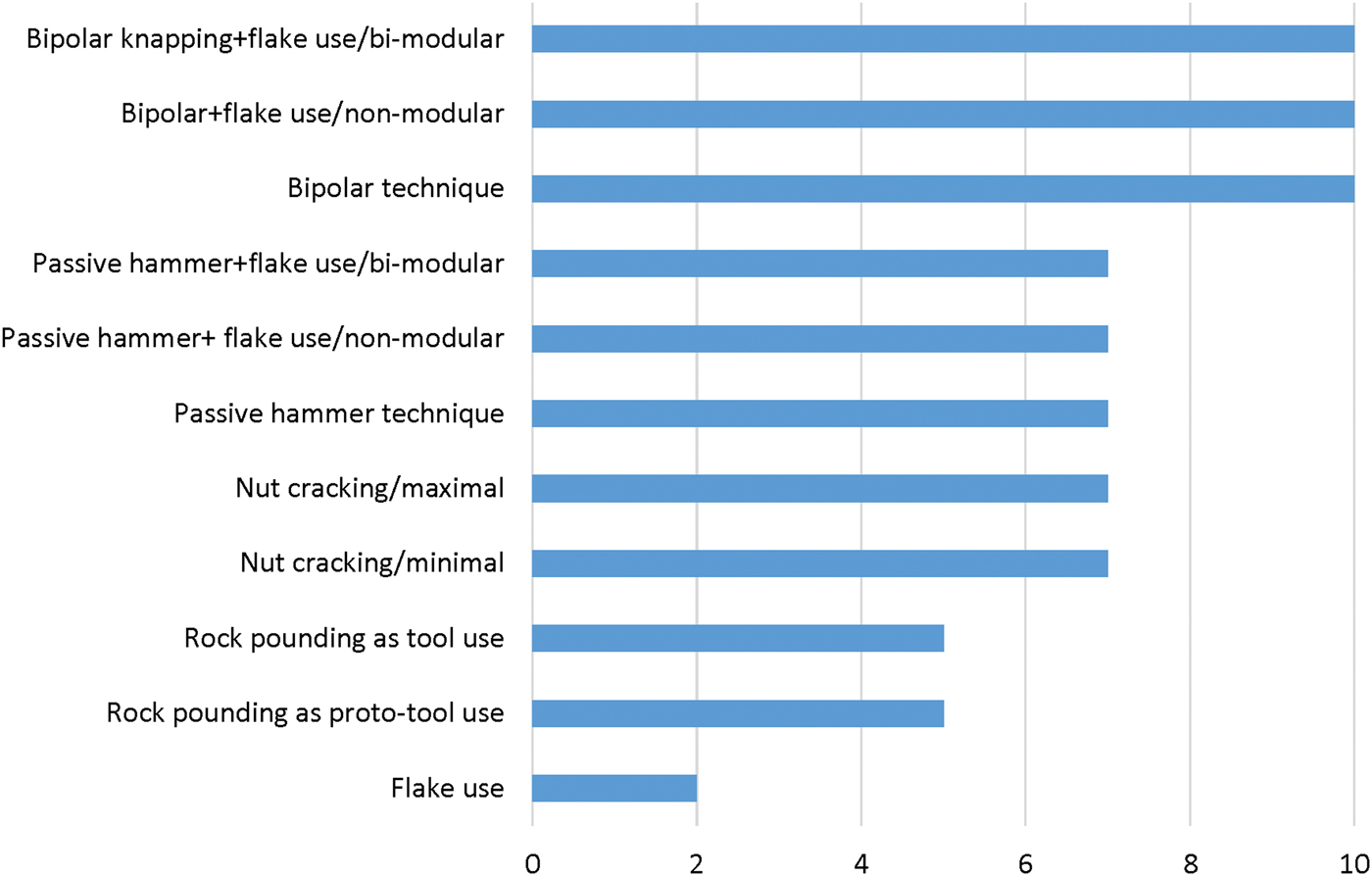
Figure 15. Interpretation of the maximum attention span required for each techno-behaviour based on its most extended phase.
To help guide comparative discussion, we use a scoring system to rank the techno-behaviours in terms of expert cognition, causal cognition and modes of teaching (Tables 4–6). The scoring systems for each table is explained in the accompanying heading. Details for our interpretations have been discussed in the ‘approach’ and ‘thinking through’ sections above, so that we now only present our summative readings for the techno-behaviours in the context of each model.
Table 4. Interpretation of expert cognition, based on what is known or can be reasonably argued for each techno-behaviour. The lowest score is 0 and the highest 5 with a ‘probably’ scored at 3, with grades of variation in between as indicated in the table. Note that the two categories below the double line are scored in reverse, i.e., a low score for presence, and a higher score for less narrow or less automatic responses. The techno-behaviours are then ranked from lowest to highest scoring.

Table 5. Interpretation of causal cognition, based on what is known or can be reasonably argued for each techno-behaviour. The lowest score is 0 and the highest 5, with grades of variation in-between as indicated in the table.

Table 6. Interpretation of teaching modes, based on what is known or can be reasonably argued for each techno-complex. Since each teaching mode builds on the former, the total score is based on accumulated numbers of comments in each column. No or No, not needed = no score. All other comments per cell give one score.
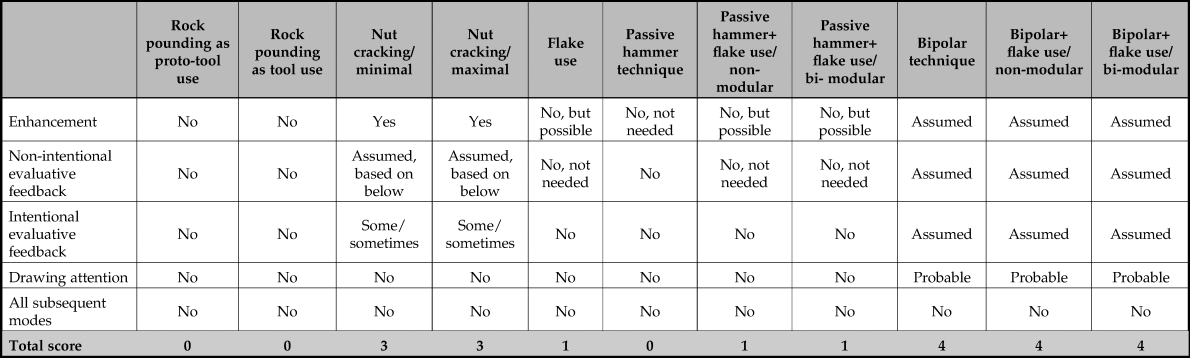
Our best-fit interpretation is that the two pounding behaviours display very little in terms of expert cognition (Fig. 16). In fact, such performances only conform to the narrow field of activity and automatic response categories (below the double line in Table 4), requiring none of the other cognitive skills associated with this kind of cognition. In this analysis, flake use and passive hammer knapping are equally ranked below the two nut-cracking performances. Maximal nut cracking slightly outranks the minimal performance, and bar the passive hammer technique, all the hominin knapping behaviours outrank nut cracking (Fig. 16). Analysed in detail and considering each of the seven characteristics of expert cognition separately, the comparison of the different percussive techniques reveals more differences than previously described (Wynn et al. Reference Wynn, Haidle, Lombard, Coolidge, Wynn and Coolidge2017, 30–34). Still, there is no apparent qualitative leap. Rather, based on our approach, a gradual extension of the different aspects of expert cognition can be recorded. We suggest that our analysis demonstrates the increasing pressure on effective memory chunking and chaining as techno-behaviours became expanded through time and across space.
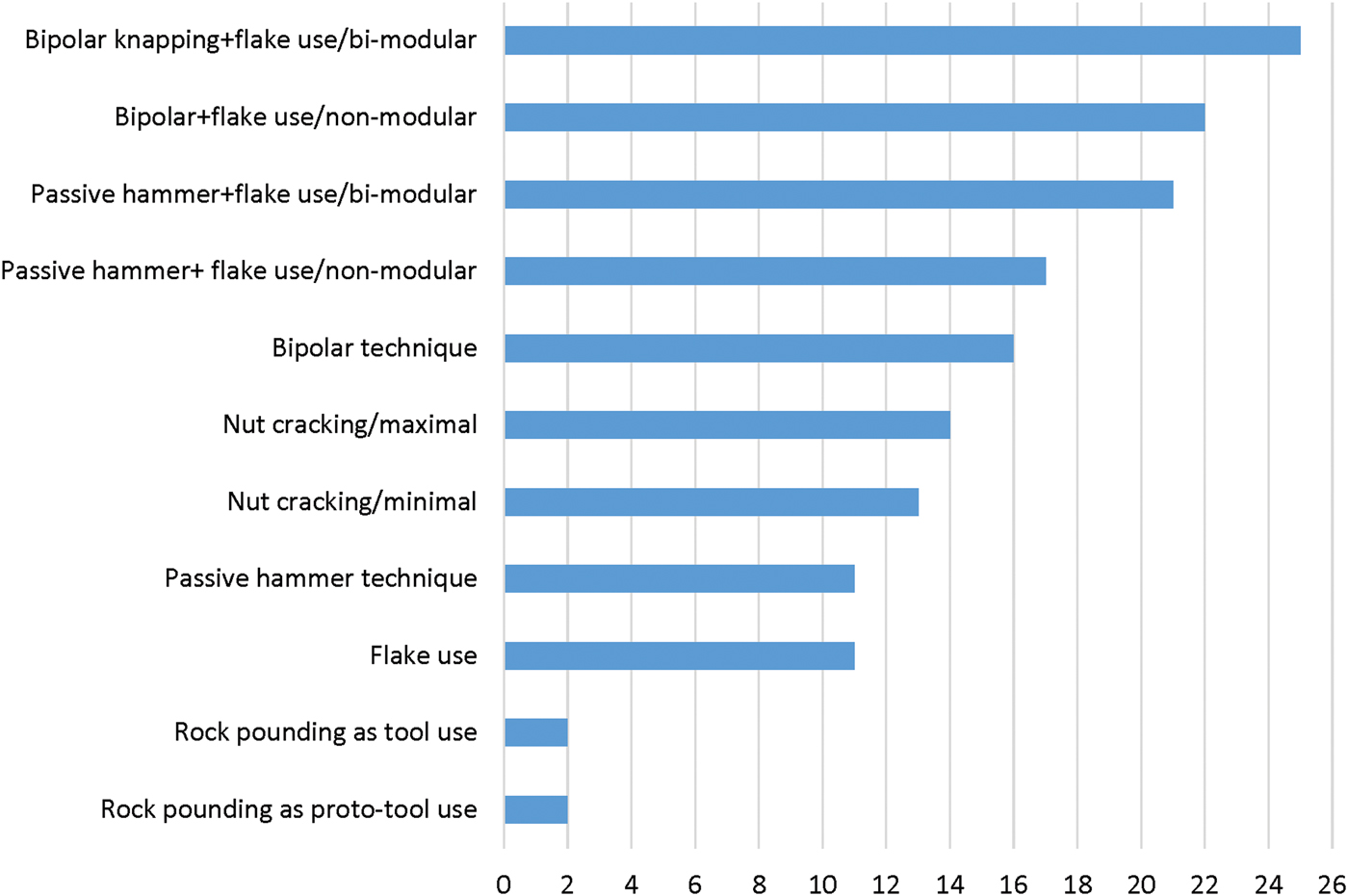
Figure 16. Ranking of expert cognition required for each techno-behaviour based on the total scores in Table 4.
Grade 1 causal cognition can be reasonably assumed for all the techno-behaviours. Grade 2, the understanding of a conspecific's agency, is partly displayed in the pounding performances, and presumably present in all the other techno-behaviours. We suggest that self-awareness (a form of Grade 3 causal cognition), which involves the ability to imagine oneself in the future and in the past, is absent in proto-tool pounding. Limited self-awareness is, however, present in pounding with a tool, both nut-cracking scenarios, flake use, passive hammer knapping and non-modular passive hammer + flake use.
For the remaining four techno-behaviours, some self-awareness is required. There is no reason to suggest Grade 3 conspecific mindreading for either of the pounding behaviours, but basic shared attention/intention is present in the same range of performances as suggested for limited self-awareness. Modular passive hammer + flake use, bipolar knapping, non-modular bipolar knapping + flake use and modular bipolar knapping + flake use require at least some shared attention/intention. We do not see evidence for any of the more sophisticated grades of causal cognition in any of the techno-behaviours in our study (Table 5). If our interpretation is correct, then the inferred variations in Grade 3 causal cognition reflect a steady increase in episodic memory, working memory and priority scheduling (Fig. 17) (also see Suddendorf & Corballis Reference Suddendorf and Corballis2007 on mental time travel). Increasing efficiency by using techno-behaviours such as bipolar knapping and modular performances could have helped stimulate the gradual evolution of these key cognitive elements in hominins.
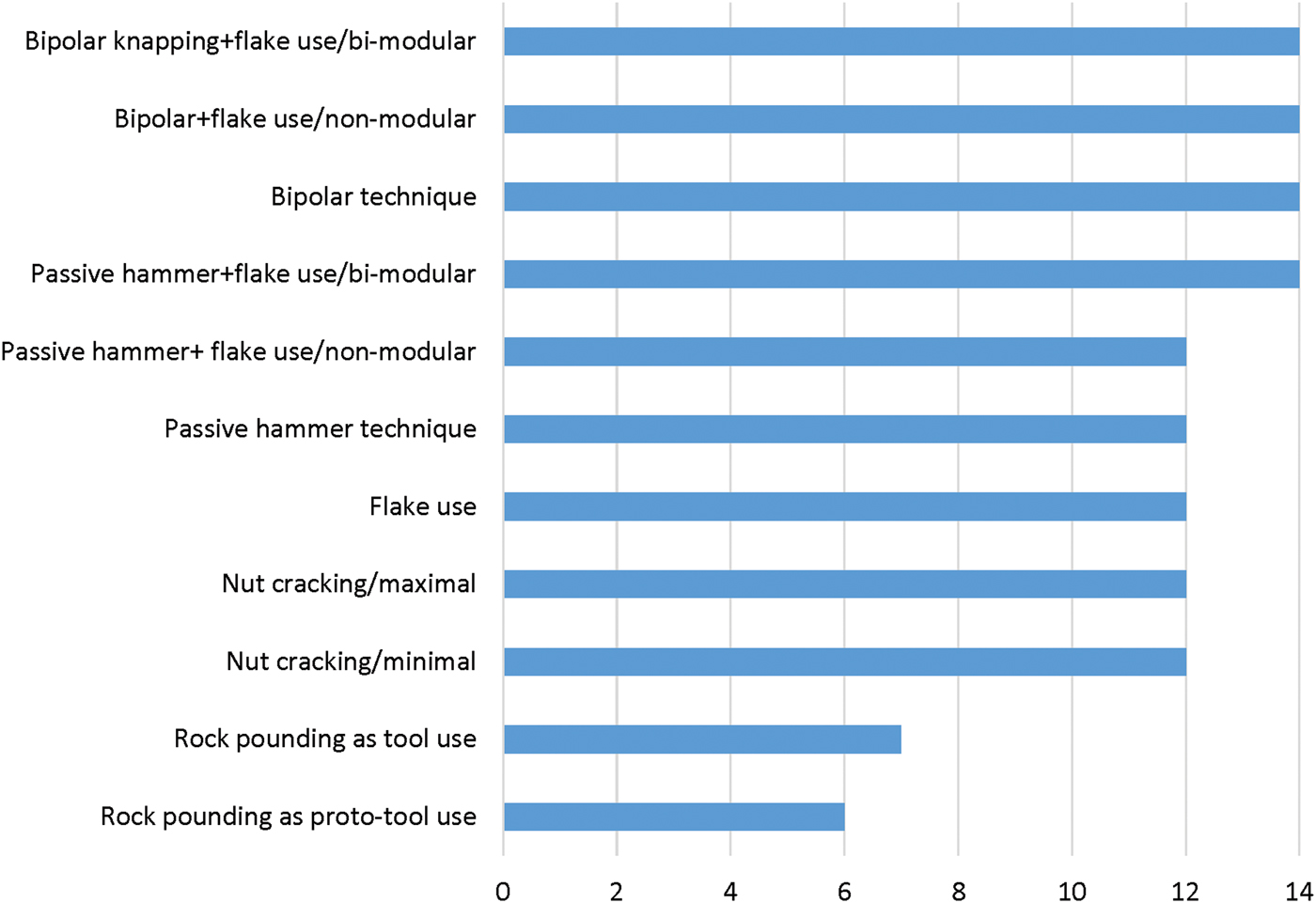
Figure 17. Ranking of causal cognition required for each techno-behaviour based on the total scores in Table 5.
Whereas some form of social learning is inherent in the pounding behaviours, there is no evidence thus far for the presence of intentional teaching. We acknowledge that some basic forms of non-intentional teaching could well be present in techno-behaviours such as cutting with a flake and passive hammer knapping, but that it is not a requirement. Non-modular and modular passive hammer and flake use possibly required some non-intentional teaching through enhancement to become entrenched in hominin behaviour.
Both nut-cracking scenarios incorporate teaching through enhancement, and a few occasions of intentional evaluative feedback have been reported. The latter implies that chimpanzees who provide intentional evaluative feedback to novice nut-crackers also have the capacity for non-intentional evaluative feedback. Teaching through drawing attention is probably required for all the bipolar knapping scenarios. It is therefore reasonable to assume that teaching skills such as providing enhancement and evaluative feedback (non-intentional and intentional) were already established in hominin groups who practised this techno-behaviour.
Teaching modes such as demonstrating, communicating concepts and explaining relationships between concepts are not required for any of the techno-behaviours in our study (Table 6; Fig. 18). We draw attention to the caveat of evidence for hominin knapping scenarios in this analysis. Hominins can no longer be observed during their performances, but living primates can.
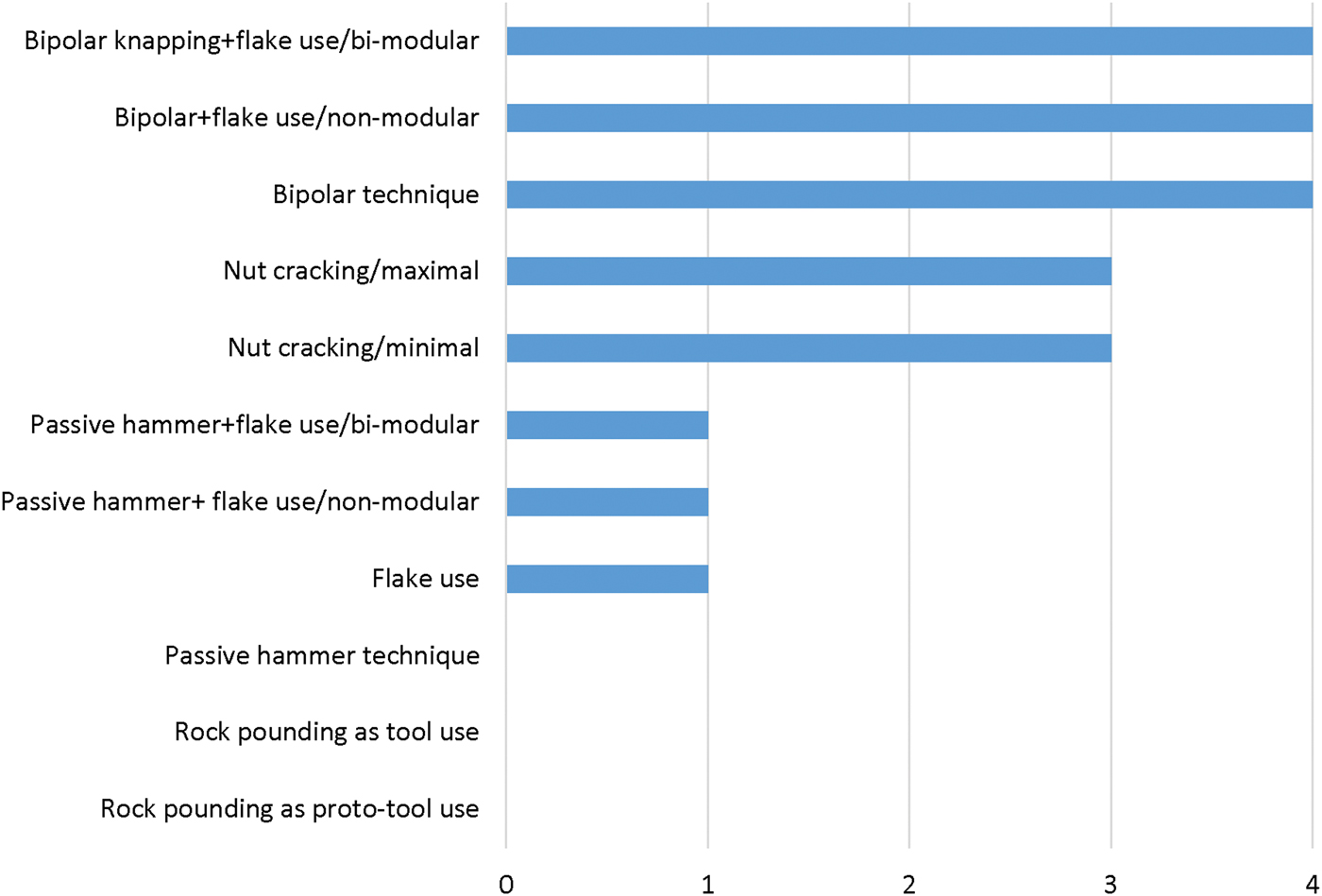
Figure 18. Ranking of teaching modes for each techno-behaviour based on the total scores in Table 6.
Concluding remarks
With our multi-model approach, we were able to highlight those behaviours that outrank others in terms of specific cognitive requisites (Figs. 13–18). We revealed marked differences between capuchin pounding activities and the bipolar knapping scenarios recorded at Lomekwi 3. Directly matched against each other, these techno-behaviours demonstrate variation in cognitive requirements. The pounding behaviours were consistently ranked lowest, and the bipolar techniques (non-modular and bi-modular) consistently outranked all of the other performances in terms of cognitive repertoire expressed in their execution. When we consider the overall ranking of all 11 techno-behaviours (Fig. 19), this observation is distinct. We therefore argue that the cognitive requirements for the pounding behaviours, as observed in some capuchin groups, are considerably less than those associated with all the knapping scenarios, and radically so for the knapping + flake use performances.

Figure 19. Comparison of the 11 techno-behaviours in relation to each of our four approaches, based on information contained in Tables 3–6. Note that units used for the horizontal axis are not comparable between the four approaches.
Although both the nut-cracking behaviours require less in terms of cognition compared to the bipolar knapping scenarios, they too clearly outrank the pounding behaviours. We therefore suggest a cognitive hierarchy between pounding behaviours (as observed in bearded capuchin groups), chimpanzee nut-cracking behaviours and the use of bipolar knapping techniques (Fig. 19). Our conclusion is that the hominins responsible for bipolar flake production at Lomekwi 3 were cognitively more advanced than chimpanzees cracking nuts today, and certainly more so than the rock-pounding bearded capuchin monkeys.
Acknowledgements
We thank Thomas Wynn, an anonymous reviewer and the editor for their constructive feedback on our initial submission. The research of Lombard is funded by an African Origins Platform Grant (number 98815) awarded by the National Research Foundation of South Africa. The research of Högberg is funded by the Swedish Research Council (grant number 721-2014-2100). Opinions and mistakes remain our own and cannot be ascribed to the funding agencies or our respective institutions.