Introduction
Whether from bacterial or animal origin, the surface of a cell is globally negatively charged. The anionic molecules that are found at cell surfaces and that constitute and organise the cell membrane are chemically very different according to the bacterial or animal origin, and endow the respective membranes with different physicochemical properties in terms of reactivity, non-covalent interactions, deformation and plasticity (Harayama and Riezman, Reference Harayama and Riezman2018). These differences in the physicochemical properties of membrane cells are crucial, since a membranotropic molecule such as a cell-penetrating peptide (CPP) or an antimicrobial peptide (AMP) does not interact with the same partners at the cell surface of a bacteria or a mammal cell, and thus has distinct activities: CPPs are indeed expected to enter cells without causing any damage to the cell membrane, whereas membrane-targeted AMPs must somehow disrupt or disorganise irreversibly the membrane to exert their antibacterial activities.
On the one side for procaryotes, Gram-negative bacteria contain two lipid membranes (the outer and the inner one), when Gram-positive have only one lipid membrane (Fig. 1a and b). In these membranes (outer one for Gram-negative), the inner leaflet is composed of different phospholipids when the outer leaflet also incorporates glycoconjugates. The lipid bilayer content differs from one species to the other, but phosphatidylethanolamine (PE), phosphatidylglycerol (PG) and cardiolipin (CL) are generally shared among all (Sohlenkamp and Geiger, Reference Sohlenkamp and Geiger2016). For Escherichia coli for example, the lipid bilayer contains about 75% of PE (zwitterionic polar head), 20% of PG (anionic polar head) and 5% of CL (anionic polar head). For Gram-negative and -positive bacteria the outer cell envelopes are different. In Gram-positive bacteria, there is a thick layer of crosslinked peptidoglycan interweaved by long anionic polymers, called teichoic acids, which surrounds the cytoplasmic membrane and forms a matrix conferring negative charge and rigidity to the bacterial cell. In Gram-negative cells, the inner and outer membranes are separated by a thin peptidoglycan cell wall. The outer leaflet of the outer membrane is generally formed by lipopolysaccharides (LPS), which consists of lipid A modified by a core polysaccharide and a very variable O-antigen.
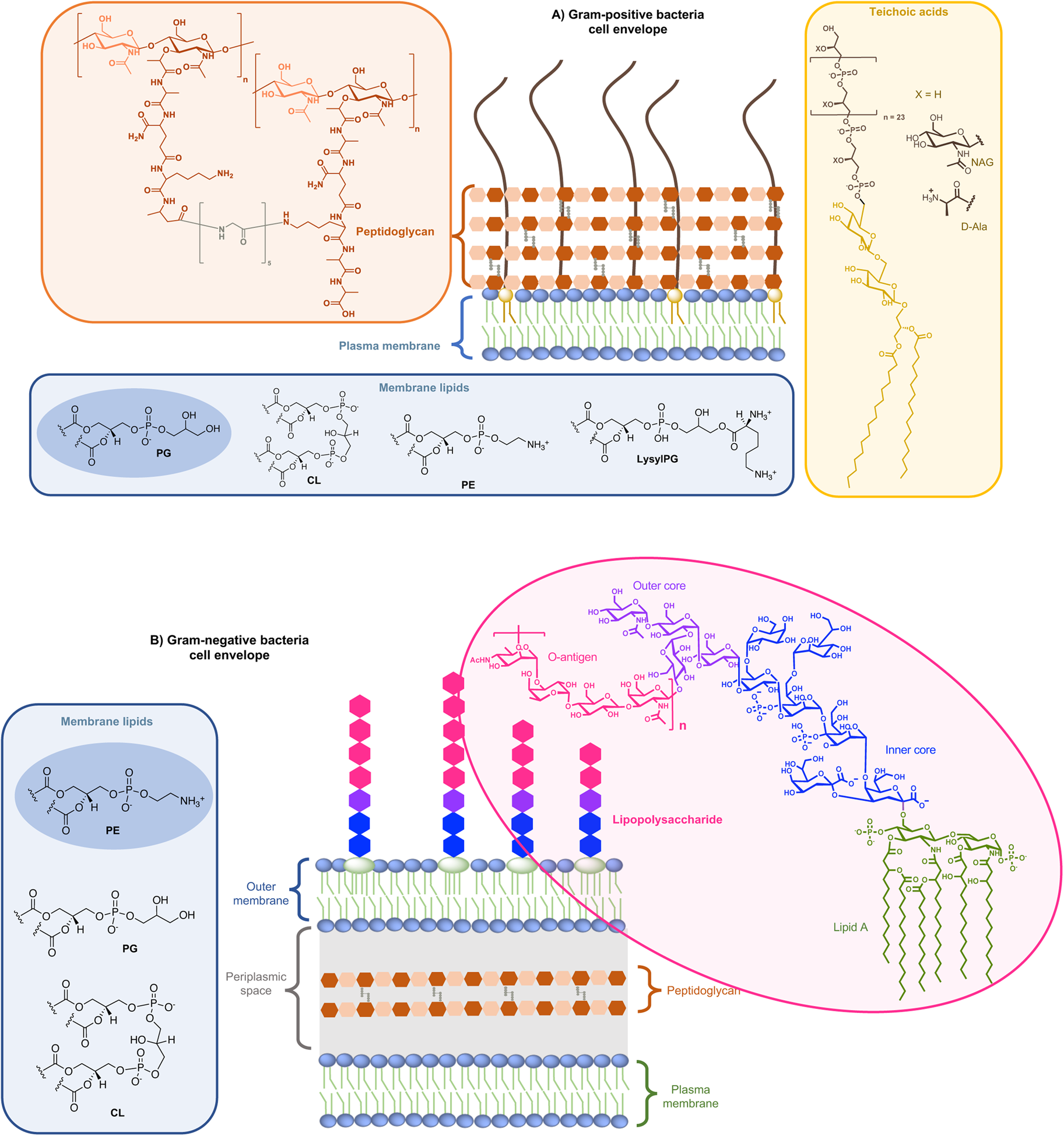
Fig. 1. Structure of glycoconjugates and lipids of Gram-positive (a) and Gram-negative (b) cell envelopes. The most abundant lipids are highlighted.
On the other side, for animal cells (Fig. 2), 65–75% of phosphatidylcholine (PC, zwitterionic polar head) and >85% of sphingomyelin (SM, zwitterionic polar head), are found in the outer leaflet of the plasma membrane, whereas 80–85% of PE, >96% of phosphatidylserine (PS, anionic polar head) and 80% of phosphatidylinositol (PI, anionic polar head) and phosphatidylinositol-4,5-bisphosphate (PI(4,5)P2, anionic polar head), are located in the inner leaflet (Kobayashi and Menon, Reference Kobayashi and Menon2018). Embedded within the lipid bilayer and pointing to the exterior of the cells, proteoglycans carrying long and negatively charged glycosaminoglycans (GAGs) form, with other oligo- and polysaccharides, a viscous and anionic matrix layer surrounding the animal cells (Theocharis et al., Reference Theocharis, Skandalis, Gialeli and Karamanos2016)
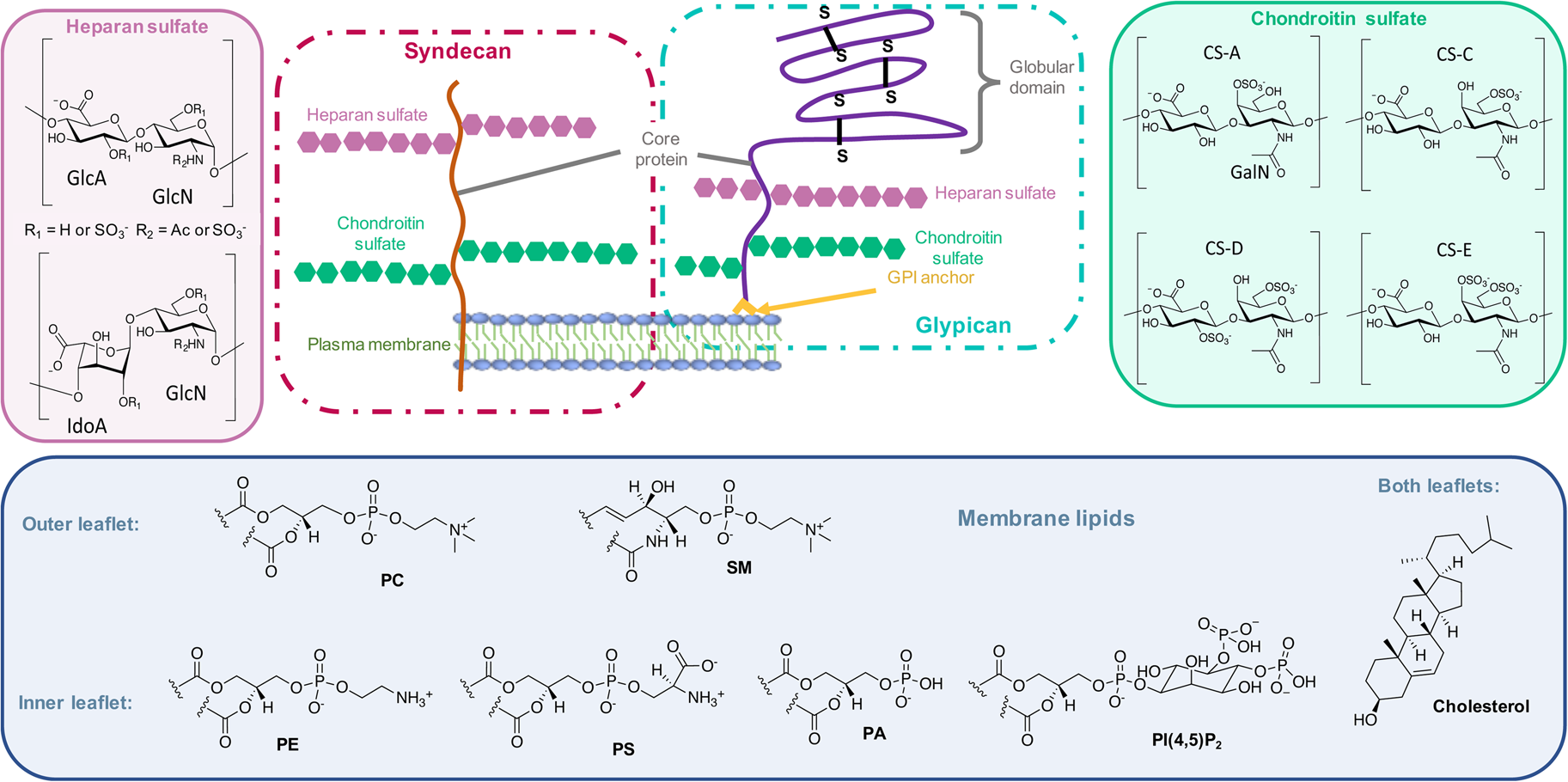
Fig. 2. Structure of glycoconjugates and lipids of animal cell membranes.
Thus, for both bacteria and animal cells, negatively charged layers of glycolipids, glycoproteins, oligosaccharides, and polysaccharides represent a barrier with the extracellular medium in addition to the lipid bilayer.
In this context, in the first step of their membranotropic activity, AMPs and CPPs meet this anionic matrix before eventually accumulating at the vicinity of the lipid bilayer. Thus, interactions between these cationic peptides with these anionic components of the membranes must be taken into account in the antibacterial (Malanovic and Lohner, Reference Malanovic and Lohner2016b; Savini et al., Reference Savini, Loffredo, Troiano, Bobone, Malanovic, Eichmann, Caprio, Canale, Park, Mangoni and Stella2020) or cell-penetrating (Walrant, Bechara, et al., Reference Walrant, Bechara, Alves and Sagan2012a; Walrant et al., Reference Walrant, Cardon, Burlina and Sagan2017) activities of the peptides, in addition to their interaction with lipid bilayers.
Among AMP and CPP sequences, we found those containing Trp residues of utmost interest. Trp is indeed unique among the natural aminoacids and can establish peculiar interactions, in particular in the context of lipid membranes (Khemaissa et al., Reference Khemaissa, Sagan and Walrant2021). Taken alone, out of a peptide sequence context, this essential amino acid spends indeed long periods of time at the water-membrane interface, and is able to bridge a few lipids and cholesterol chains by means of hydrogen-bonds (Lu and Martí, Reference Lu and Martí2018). Given its rich electronic cloud and dipole moment, Trp can establish and be involved in both polar interactions, such as hydrogen-bonds or π-interactions, and hydrophobic interactions. In the following review, we intend to give clues and evidences for the crucial role of Trp in cationic sequences of membranotropic peptides: AMPs, CPPs or both and to show, when this aspect has been studied and reported, how these unique properties can explain the interactions and activities at the cell-membrane.
Trp, unique electronic and dipole properties
For many reasons, Trp holds a very peculiar position amongst the twenty naturally encoded amino-acids. It has the largest side chain, it is part of the aromatic family, together with Phe, Tyr and His, and has many interesting physico-chemical properties (Fig. 3a). Depending on how hydrophobicity is evaluated, Trp can be regarded as the most hydrophobic amino-acid (Wimley and White, Reference Wimley and White1996), moderately hydrophobic (Kyte and Doolittle, Reference Kyte and Doolittle1982) or even only very moderately hydrophobic (Moon and Fleming, Reference Moon and Fleming2011). Interestingly, in membrane-proteins, Trp is found at the water-bilayer interface rather than deeply buried in the membrane. For example, this was observed for the AMP Gramicidin, which forms channels in lipid bilayers (Koeppe et al., Reference Koeppe, Killian and Greathouse1994; Yau et al., Reference Yau, Wimley, Gawrisch and White1998).
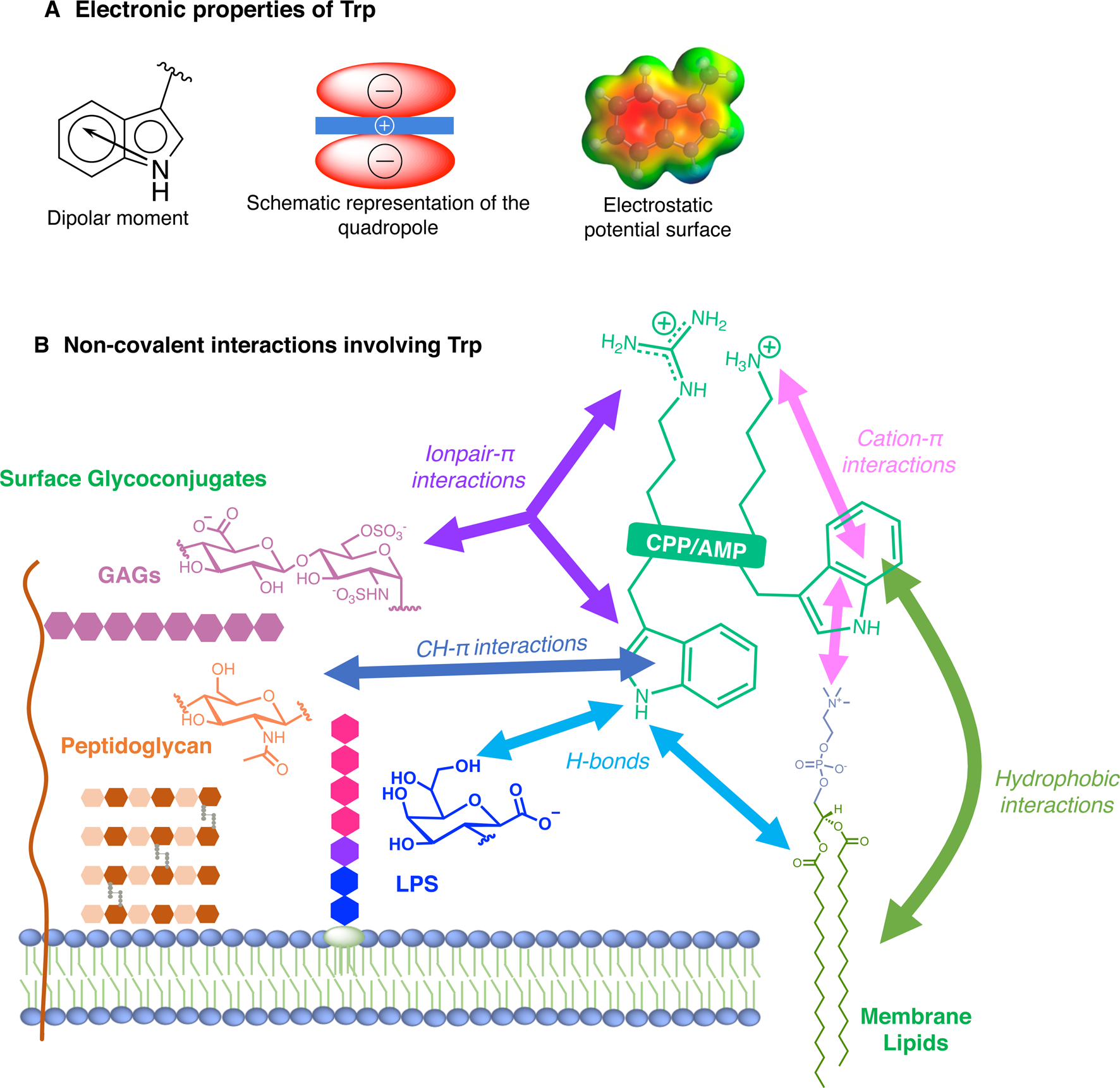
Fig. 3. Electronic properties of Trp (a) and summary of the interactions of Trp with membrane lipids and glycoconjugates (b).
More precisely, Trp localises in the region of acyl carbonyl groups of the lipid bilayer (Yau et al., Reference Yau, Wimley, Gawrisch and White1998; Sanderson, Reference Sanderson2005). Regarding its orientation, the benzene moiety of the indole localises in the hydrophobic core whereas the pyrrole moiety points towards the more hydrophilic part of the lipid bilayer, the so-called polar head groups: serine, ethanolamine, choline, glycerol etc (Landolt-Marticorena et al., Reference Landolt-Marticorena, Williams, Deber and Reithmeier1993).
The benzene ring and the pyrrole ring confer to Trp a permanent dipole moment (2.1 D) pointing from the nitrogen (N1) in the five-membered ring to the carbon C5 in the six-membered ring. The indole ring thus contains a cyclic secondary amine surrounded by a more positively charged area because of its slightly acidic properties (Fig. 3a). The aromaticity of the ring indeed implies that the lone pair of electrons on the nitrogen atom is not available for protonation. This unique π system of indole can lead to strengthened cation-π (electrostatic nature) interactions (Klein-Seetharaman et al., Reference Klein-Seetharaman, Oikawa, Grimshaw, Wirmer, Duchardt, Ueda, Imoto, Smith, Dobson and Schwalbe2002) and also to anion-π (ion-induced polarisability and electrostatic nature) interactions (Schottel et al., Reference Schottel, Chifotides and Dunbar2008; Frontera et al., Reference Frontera, Gamez, Mascal, Mooibroek and Reedijk2011).
Trp can be engaged in hydrogen-bonds either through its NH group or its two aromatic rings. The NH group of Trp is a hydrogen-bond donor and rings are acceptors. It is worth mentioning that conventional hydrogen bonds of the N-H-Y type are stronger compared to X-H-π ones, about twice more in terms of energetics (Scheiner et al., Reference Scheiner, Kar and Pattanayak2002). When the NH group is engaged in a hydrogen-bond, the system adopts a planar configuration due to the planar aromatic ring, with a typical distance between the donor and the acceptor of about 0.3 ± 0.02 nm. In the case of the aromatic rings, the X-H-π hydrogen bond is perpendicularly directed to the centre of the rings (Scheiner et al., Reference Scheiner, Kar and Pattanayak2002).
Additionally, it is now well-documented that aromatic amino acids can establish C-H-π interactions, by the combination of electronic and hydrophobic forces (Kiessling and Diehl, Reference Kiessling and Diehl2021). Of utmost interest is that these C-H-π bonds are crucial in glycan/protein interactions, such as for the case of lectins (Gabius et al., Reference Gabius, André, Jiménez-Barbero, Romero and Solís2011). Generally, Trp is the most frequent amino acid found within a distance of 0.4 nm between a protein and a noncovalently bound glycan, compared to its frequency in the UniProt database (Hudson et al., Reference Hudson, Bartlett, Diehl, Agirre, Gallagher, Kiessling and Woolfson2015). More than one correctly oriented C-H bonds, and generally three, are necessary to interact with the aromatic residue, meaning that some carbohydrates, such as β-galactose, form stronger C-H-π interactions than others, such as N-acetylglucosamine. Additionally, the more acidic the C-H, the better the interaction in terms of energetics (Ramírez-Gualito et al., Reference Ramírez-Gualito, Alonso-Ríos, Quiroz-García, Rojas-Aguilar, Díaz, Jiménez-Barbero and Cuevas2009).
Trp can thus establish and be involved in many types of interactions with membrane components. To summarise, Trp can engage in hydrogen-bonds as a donor or acceptor, dipole-dipole and dipole-charge interactions, hydrophobic C-H-π interactions, as well as π-π and π-cation interactions. Recently, anion-π interactions involving Trp residues and phospholipid head groups have been shown in a membrane protein SERCA1 (Chakravarty et al., Reference Chakravarty, Ung, Moore, Shore and Alshamrani2018), and ion pair-π interactions involving Trp residues in a CPP evidenced (Walrant et al., Reference Walrant, Bauzá, Girardet, Alves, Lecomte, Illien, Cardon, Chaianantakul, Pallerla, Burlina, Frontera and Sagan2020). All possible interactions involving Trp are summarised in Fig. 3b.
Therefore, for a comprehensive analysis of the role of Trp in membranotropic peptides, one should take into account not only the interactions of this amino acid with the lipid bilayer, but also all potential partners at the cell surface, being either a bacteria or an animal cell.
In order to study the impact of Trp residues in peptides and proteins, it is possible to substitute it by either natural aromatic amino-acids such as Phe or Tyr, but also by non-natural analogues. These are particularly easy to introduce by peptide synthesis, but some can also be introduced through recombinant expression (Arias et al., Reference Arias, Hoffarth, Ishida, Aramini and Vogel2016). These non-natural analogues are useful tools to better understand the role of Trp residues in the activity of membrane-active peptides. These analogues can be polar or apolar, have an enriched electronic cloud or not, can retain the ability to engage in hydrogen bonding or not, or have specific properties, such as fluorescence. The structures of the analogues cited in this review are presented in Fig. 4.
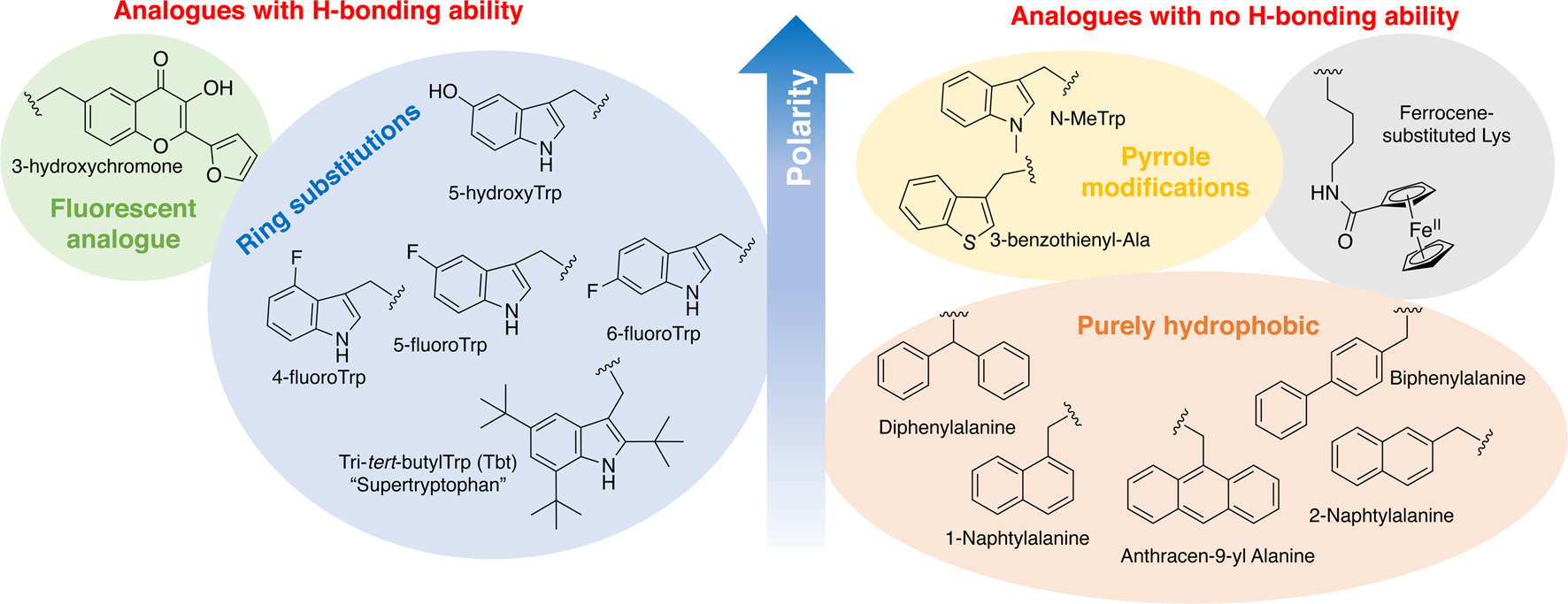
Fig. 4. Structure and properties of Trp analogues cited in this review.
Effect of Trp residues in CPP internalisation
CPPs are a sub-family of membrane-active peptides that are able to cross cell-membranes without causing damage. The first CPPs, Penetratin (or antennapedia peptide, penetratin, Antp) and Tat were identified in the early 90'S and since then a broad variety of cell-penetrating sequences have been discovered. CPPs are very diverse in terms of origin and sequence, a few examples are given in Table 1.
Table 1. Sequences of natural and rationally designed CPPs
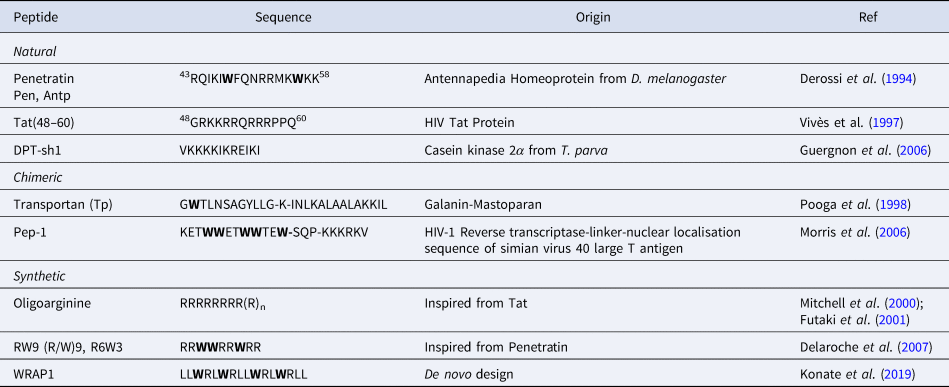
Trp48 in homeodomain-derived CPPs
Penetratin, which was discovered in 1994, corresponds to the third helix of the homeodomain of the homeoprotein Antennapedia from Drosophila (Derossi et al., Reference Derossi, Joliot, Chassaing and Prochiantz1994). Homeoproteins are a family of important transcription factors responsible for neuronal differentiation during embryogenesis and development (Le Roux et al., Reference Le Roux, Henri Joliot, Bloch-Gallego, Prochiantz and Volovitch1993). These cationic proteins are secreted and internalised in different cell populations and have the capacity to bind DNA via a region called homeodomain. Homeodomains are highly conserved 60 amino acids sequences with a characteristic folding composed of three alpha helices. The homeodomain region of homeoproteins is responsible for cell-penetration properties (Prochiantz, Reference Prochiantz2000). Sequence alignment studies on 346 homeoproteins revealed that Trp48 is conserved in more than 95% of homeodomains whereas Trp56 is only conserved at 32%. (Gehring et al., Reference Gehring, Affolter and Bürglin1994).
In addition to Penetratin, several homeodomain-derived CPPs have been identified. They all correspond to the third helix of a homeodomain (Balayssac et al., Reference Balayssac, Burlina, Convert, Bolbach, Chassaing and Lequin2006). Interestingly, they all share the highly conserved Trp48 (Table 2). On the other hand, only Penetratin has a Trp in position 56.
Table 2. sequences of homeodomain derived CPPs showing the conservation of Trp48 (Trp52 in Knott, due to a shift of three residues)
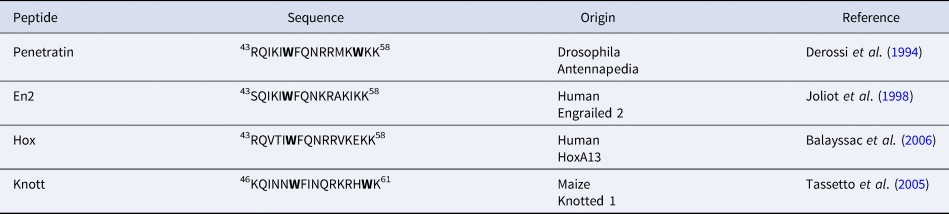
The involvement of both Trp in the internalisation of Penetratin and Antennapedia homeoprotein has been heavily studied. Deletion of residues Trp48 and Phe49 in Antennapedia homeodomain leads to abolished internalisation of the homeodomain (Le Roux et al., Reference Le Roux, Henri Joliot, Bloch-Gallego, Prochiantz and Volovitch1993). Similarly, a double mutant [W48F, W56F]-Penetratin is no longer internalised (Derossi et al., Reference Derossi, Joliot, Chassaing and Prochiantz1994). Surprisingly, a few years later the same double mutant was found to be as efficiently internalised as Penetratin, but with slower kinetics (Thorén et al., Reference Thorén, Persson, Isakson, Goksör, Önfelt and Nordén2003). Single mutant studies allowed to evaluate the importance of Trp48 and Trp56 separately. Ala-scan type studies performed in the early 2000's provided contradictory results. Fischer et al., showed that substitution of Trp56 by Ala led to a 50% decrease in internalisation whereas with substitution of Trp48, the mutant peptide was still internalised up to 75% of wild-type Penetratin (Fischer et al., Reference Fischer, Zhelev, Wang, Melville, Fåhraeus and Lane2000). A year later, Drin et al., showed that substitution of any hydrophobic residue, including Trp48 and Trp56 by Ala led to a large decrease of uptake (Drin et al., Reference Drin, Mazel, Clair, Mathieu, Kaczorek and Temsamani2001), suggesting no specific role for Trp. Finally, in 2004, two single mutants W48F-Pen and W56F-Pen were studied and it was showed that the W48F mutation affected direct translocation more than W56F (Christiaens et al., Reference Christiaens, Grooten, Reusens, Joliot, Goethals, Vandekerckhove, Prochiantz and Rosseneu2004). It is also interesting to point that amongst four homeodomain-derived CPPs, only Penetratin has a Trp in position 56, but all four peptides are internalised to the same extent as Penetratin, or even more, confirming that Trp56 is less critical than Trp48 for internalisation (Balayssac et al., Reference Balayssac, Burlina, Convert, Bolbach, Chassaing and Lequin2006).
Rationally-designed CPPs from penetratin
An interesting case is that of Penetratin-derived R/W peptides. In 1998, a very simplified hexadecapeptide composed solely of Arg and Trp residues was proposed as a Penetratin model (Chassaing and Prochiantz, Reference Chassaing and Prochiantz1997; Derossi et al., Reference Derossi, Chassaing and Prochiantz1998). This peptide, called RW16, or (R/W)16 retains the amphipathic alpha helical structure proposed for Penetratin, and is as efficiently internalised. The analogue composed of Arg and Leu (RL16), on the other hand, is not internalised. Both RW16 and RL16 peptides are highly cytotoxic (Alves et al., Reference Alves, Goasdoué, Correia, Aubry, Galanth, Sagan, Lavielle and Chassaing2008; Delaroche et al., Reference Delaroche, Cantrelle, Subra, Van Heijenoort, Guittet, Jiao, Blanchoin, Chassaing, Lavielle, Auclair and Sagan2010). In 2007, a shorter analogue of RW16 is introduced (Delaroche et al., Reference Delaroche, Aussedat, Aubry, Chassaing, Burlina, Clodic, Bolbach, Lavielle and Sagan2007). This peptide is called RW9, (R/W)9, R6W3 or R6/W3 in the literature. RW9 is efficiently internalised in various cell lines (Delaroche et al., Reference Delaroche, Aussedat, Aubry, Chassaing, Burlina, Clodic, Bolbach, Lavielle and Sagan2007; Aubry et al., Reference Aubry, Burlina, Dupont, Delaroche, Joliot, Lavielle, Chassaing and Sagan2009), whereas its Arg/Leu counterpart RL9 is not (Walrant et al., Reference Walrant, Correia, Jiao, Lequin, Bent, Goasdoué, Lacombe, Chassaing, Sagan and Alves2011). RW9 also has antimicrobial properties, unlike RL9. The substitution of the three Trp residues by Phe in RW9 leads to an abolition of the internalisation, and the substitution of only one or two Trp residues diminishes significantly the uptake (Jobin et al., Reference Jobin, Blanchet, Henry, Chaignepain, Manigand, Castano, Lecomte, Burlina, Sagan and Alves2015). Similarly, the analogues where Trp residues are substituted by amino-acids with galactosyl-modified side-chains are also poorly internalised (Dutot et al., Reference Dutot, Lécorché, Burlina, Marquant, Point, Sagan, Chassaing, Mallet and Lavielle2010; Lécorché et al., Reference Lécorché, Walrant, Burlina, Dutot, Sagan, Mallet, Desbat, Chassaing, Alves and Lavielle2012). A cyclic analogue of RW9 has also been designed and it shows slightly enhanced internalisation properties compared to linear RW9 (Amoura et al., Reference Amoura, Illien, Joliot, Guitot, Offer, Sagan and Burlina2019). RW9 was recently shown to stimulate endocytic uptake by promoting membrane curvature (Masuda et al., Reference Masuda, Hirose, Baba, Walrant, Sagan, Inagaki, Fujimoto and Futaki2020). The ability of RW9 and analogues to transfect plasmid DNA by forming DNA/peptide nanoparticles was also assessed (Alhakamy, Dhar, and Berkland, Reference Alhakamy, Dhar and Berkland2016). Only RW9 and R9 showed high transfection abilities, whereas RL9, RA9 and RH9 were not as effective.
Addition of Trp in cationic sequences
Even though Trp residues are critical for the internalisation of homeodomain-derived CPPs, it is not an absolute requirement for the efficient internalisation of natural CPPs. Tat peptide, derived from the Tat protein of HIV-1 is indeed devoid of Trp residues. Interestingly, the Tat protein contains a single Trp residue at position 11, upstream of the CPP sequence, also called the Protein Transduction Domain (Tat peptide). Despite a high mutation rate of HIV-1, this residue is largely conserved. Mutation of this residue does not affect the endocytosis of Tat protein, but this residue is required for the translocation of the Tat protein across the endosomal membrane (Yezid et al., Reference Yezid, Konate, Debaisieux, Bonhoure and Beaumelle2009). In addition, a mutant Tat peptide where Pro59 is substituted by a Trp has enhanced internalisation properties (Thorén et al., Reference Thorén, Persson, Isakson, Goksör, Önfelt and Nordén2003).
Purely synthetic and simplified CPP sequences based on Tat peptide have been designed. For example, the purely cationic Tat sequence inspired the design of oligoarginines, which are now extensively used as efficient CPPs. Addition of a single Trp residue at the C-terminus of heptaarginine sequences (R7W) (Thorén et al., Reference Thorén, Persson, Isakson, Goksör, Önfelt and Nordén2003; Maiolo et al., Reference Maiolo, Ferrer and Ottinger2005), or hexaarginine (R6W) (Mishra et al., Reference Mishra, Lai, Schmidt, Sun, Rodriguez, Tong, Tang, Cheng, Deming, Kamei and Wong2011) is associated with enhanced internalisation. On the other hand, the addition of a single Trp residue in a cyclic tetraarginine sequence does not promote cellular uptake (Amoura et al., Reference Amoura, Illien, Joliot, Guitot, Offer, Sagan and Burlina2019).
Recently, simplified peptides derived from DPT-sh1, a lysine-rich CPP from C2Kα (Guergnon et al., Reference Guergnon, Dessauge, Dominguez, Viallet, Bonnefoy, Yuste, Mercereau-Puijalon, Cayla, Rebollo, Susin, Bost and Garcia2006), were designed. In these peptides, Trp and/or Arg residues were introduced in the sequence and this led to enhanced penetration properties (Zhang et al., Reference Zhang, Brossas, Parizot, Zini and Rebollo2018).
Purely synthetic Trp-containing CPPs
One of the first examples of designed CPPs is the chimeric peptide Transportan, composed of the minimal active sequence of the neuropeptide Galanin linked to pore-forming peptide Mastoparan from Wasp venom (Pooga et al., Reference Pooga, Hällbrink, Zorko and Langel1998). Pooga et al., first suggested that Trp2 and Tyr9 residues could play the same role as in Penetratin. A few years later, however, the same group introduced truncated analogues and showed that Tp10 (AGYLLGKINLKALAALAKKIL), containing no Trp residue was still efficiently internalised (Soomets et al., Reference Soomets, Lindgren, Gallet, Hällbrink, Elmquist, Balaspiri, Zorko, Pooga, Brasseur and Langel2000).
Amongst rationally designed chimeric Trp-rich CPPs, Pep-1 can be cited. This peptide is composed of a hydrophilic region, corresponding to the nuclear localisation sequence of simian virus 40 large T antigen, linked to a Trp-rich hydrophobic domain derived from the dimerisation motif of HIV-1 reverse transcriptase by a SQP linker (Morris et al., Reference Morris, Depollier, Heitz and Divita2006). Pep-1 was designed to deliver peptides and proteins to cells through a non-covalent strategy by forming nanoparticles. More recently, a new set of Trp-rich peptides for non-covalent delivery of nucleic acids, the WRAP peptides were designed (Konate et al., Reference Konate, Dussot, Aldrian, Vaissière, Viguier, Neira, Couillaud, Vivès, Boisguerin and Deshayes2019). In these peptides, Trp residues have a double function: interactions with cell membrane components to promote internalisation, and interactions with oligonucleotides by intercalation of the indole ring in the minor groove of double-stranded structures.
Since then, many Arg/Trp CPPs have been designed and studied, where the impact of the position and number of Trp residues was rationally studied. These model peptides will be described in a dedicated section below.
Importance of Trp in the activity of AMPs
Another class of membrane-active peptides that share some features with CPPs are AMPs. Trp residues can also be crucial for antimicrobial activity and are often found in natural or synthetic AMPs. One example of a class of AMPs with a highly conserved Trp residue is the Dermaseptin family, which are AMPs isolated from the skin of South American amphibians (Nicolas and El Amri, Reference Nicolas and El Amri2009). More than 80 Dermaseptin family members have been identified, and they almost all share a Trp residue at position 3.
The diversity of AMP sequences is much bigger than that of CPPs. Our aim is only to give a few examples of the role of Trp residues in AMP sequences from natural or synthetic origin (Table 3). Besides, Trp-rich AMPs have previously been extensively reviewed (Chan et al., Reference Chan, Prenner and Vogel2006; Shagaghi et al., Reference Shagaghi, Palombo, Clayton and Bhave2016).
Table 3. Sequences of Trp-containing AMPs
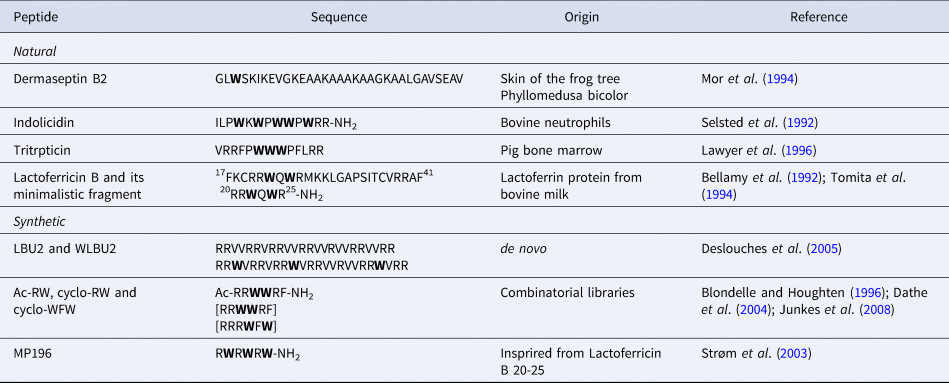
Indolicidin, a Trp-rich cell-penetrating AMP
Some natural AMPs have sequences that are particularly enriched in Trp residues. An archetypal example is Indolicidin, a peptide that was isolated from cytoplasmic granules of the bovine neutrophils (Selsted et al., Reference Selsted, Novotny, Morris, Tang, Smith and Cullor1992). Indolicidin has a very broad spectrum of activity against Gram-positive and Gram-negative bacteria, fungi, parasites and viruses, as well as toxicity towards T lymphocytes and haemolytic properties (Shagaghi et al., Reference Shagaghi, Palombo, Clayton and Bhave2016). Indolicidin acts on multiple targets as it permeabilises membranes in a non-lytic way, and tightly binds DNA thus inhibiting replication and transcription. In that perspective, Indolicin can be considered as both an AMP and a CPP.
A first structure-activity relationship (SAR) study on single Trp-to-Leu mutants showed that Trp at positions 4, 8 and 11 seem important for both antibacterial and haemolytic activities (Subbalakshmi et al., Reference Subbalakshmi, Bikshapathy, Sitaram and Nagaraj2000). On the other hand, an Ala-scan approach revealed that single substitution of Trp by Ala at all positions reduced antimicrobial activity but did not change haemolytic properties of indolicidin (Staubitz et al., Reference Staubitz, Peschel, Nieuwenhuizen, Otto, Götz, Jung and Jack2001). In the same study, substitution of all Trp by different residues (Val, Ile, Leu, Phe, Tyr, Ser, His, Lys and Glu) was investigated. Val, Ser, His, Lys and Glu analogues lost all activity. Substitution of all Trp by Leu, Ile and Tyr led to reduced antimicrobial and haemolytic activities. Finally, the Phe analogue retained its antimicrobial activity but had much reduced haemolytic properties. This is completely consistent with what was previously observed for the same Phe analogue (Subbalakshmi et al., Reference Subbalakshmi, Krishnakumari, Nagaraj and Sitaram1996). A few years later, other analogues with systematic substitution of all Trp by Phe, 1-naphtyl-Ala, 2-naphtyl-Ala or N-substituted Gly were studied (Ryge et al., Reference Ryge, Doisy, Ifrah, Olsen and Hansen2004). Substitution of Trp by 2-naphtyl-Ala led to enhanced antimicrobial properties, but also increased haemolysis.
Another study (Ando et al., Reference Ando, Mitsuyasu, Soeda, Hidaka, Ito, Matsubara, Shindo, Uchida and Aoyagi2010) suggested that substitution of Trp at position 9 by Leu leads to enhanced antimicrobial activity and reduced haemolytic properties, while the same mutation at position 11 only impacted haemolysis. The double mutant at positions 9 and 11 loses all biological activity. Interestingly, the analogue with all Trp substituted by Leu exhibits high lytic activity against Gram-negative bacteria and erythrocytes, suggesting a switch in membrane lysis mechanism.
More recently, 45 analogues of Indolicidin were studied (Smirnova et al., Reference Smirnova, Kolodkin, Kolobov, Afonin, Afonina, Stefanenko, Shpen’ and Shamova2020). Trp were substituted by Phe, para-substituted derivatives of Phe and other unnatural aromatic amino acids, such as 1-naphtyl-Ala, 3-benzothienyl-Ala or 3,3-Diphenyl-Ala. Para-substituted Phe analogues had reduced toxicity, but very variable antimicrobial activities. Electro-attractive substituents on the aromatic ring lead to increased antimicrobial activity. Analogues with non-natural aromatic amino acids have high antimicrobial activity and toxicity.
Tritrpticin and its unusual hydrophobic core
Tritrpticin is another AMP of the cathelicidin family and was first predicted from the cDNA sequence of porcine bone marrow (Lawyer et al., Reference Lawyer, Pai, Watabe, Borgia, Mashimo, Eagleton and Watabe1996). Tritrpticin has broad range antimicrobial activity against Gram-negative bacteria, Gram-positive bacteria and fungi and is significantly haemolytic. It has an interesting sequence with hydrophilic N- and C-termini, with Arg doublets flanking a hydrophobic core, with a Trp triplet at its centre. A minimalistic sequence without the terminal Arg residues is no longer active (Lawyer et al., Reference Lawyer, Pai, Watabe, Borgia, Mashimo, Eagleton and Watabe1996). SAR studies revealed that C-terminal amidation leads to enhanced activity (Schibli et al., Reference Schibli, Nguyen, Kernaghan, Rekdal and Vogel2006). Substitutions of the 3 Trp residues by Phe seem to enhance the antimicrobial activity of the peptide, while reducing its haemolytic properties (Yang et al., Reference Yang, Shin, Kim, Kim, Hahm and Kim2002; Schibli et al., Reference Schibli, Nguyen, Kernaghan, Rekdal and Vogel2006). On the other hand, substituting Trp by Tyr leads to reduced activity towards Gram-negative bacteria and reduced haemolysis (Schibli et al., Reference Schibli, Nguyen, Kernaghan, Rekdal and Vogel2006). Substitution of Trp by Ala totally abolishes the activity (Yang et al., Reference Yang, Shin, Kim, Kim, Hahm and Kim2002). Interestingly, substituting one or all Trp by 5-hydroxy Trp has very little impact on the antimicrobial activity of the peptide, whereas it strongly reduces its ability to permeabilise bacterial membranes, suggesting a non-membranolytic mechanism of action (Arias et al., Reference Arias, Jensen, Nguyen, Storey and Vogel2015). Finally, the substitution of Trp by fluorinated analogues 4-, 5- or 6-fluoro Trp has no impact on antimicrobial or bacterial membrane permeabilisation properties (Arias et al., Reference Arias, Hoffarth, Ishida, Aramini and Vogel2016).
Lactoferricin B and its minimal version
Lactoferricin B is a peptide derived from the bovine protein lactoferrin (Bellamy et al., Reference Bellamy, Takase, Yamauchi, Wakabayashi, Kawase and Tomita1992) and has broad-spectrum activity against Gram-positive and Gram-negative bacteria. An Ala-scan study revealed that Trp6 and Trp8 were essential for the antimicrobial activity of lactoferricin B (Strøm et al., Reference Strøm, Svendsen and Rekdal2000). Interestingly, human, porcine and caprine analogues, which only have one Trp at position 6, had significant increased antibacterial activity against E. coli when the residue at the position 8 was substituted by a Trp. Several shorter fragments of lactoferricin B have the similar antimicrobial activity to the parent peptide (Vogel et al., Reference Vogel, Schibli, Jing, Lohmeier-Vogel, Epand and Epand2002). Substitution of Trp residues by non-natural analogues in a shorter 15-residue analogue was studied (Haug and Svendsen, Reference Haug and Svendsen2001). Replacement of Trp by 3-benzothienyl-Ala and 2-naphtyl-Ala led to increase antibacterial activity, while 1-naphtyl-Ala analogues were somewhat less active against S. aureus, suggesting that the NH hydrogen-bond donor on the pyrrole ring is not essential for activity and that the orientation of the aromatic ring is important. Substitution of one or both Trp by other bulky aromatic residues: 3,3-diphenylalanine, biphenylalanine, anthracenylalanine and tri-tertbutyl-Trp, also led to enhanced antimicrobial activity against E. coli and S. aureus (Haug et al., Reference Haug, Skar and Svendsen2001).
Interestingly, the minimalistic amidated 6-mer 20RRWQWR25-NH2 retained significant activity and was identified as the ‘antimicrobial active site’ of lactoferricin B (Tomita et al., Reference Tomita, Takase, Bellamy and Shimamura1994). This minimalistic sequence was used as a model to develop rationally designed peptides, as described further. This short peptide, as well as the 5-mer RRWQW, also has CPP properties (Fang et al., Reference Fang, Guo, Zhang, Jiang and Ren2013a; Liu et al., Reference Liu, Huang, Aronstam and Lee2016).
Rationally designed Trp-rich AMPs
Trp residues play an important role in synthetic-designed peptides. For example, in 2005, Deslouches et al., synthesised a series of peptides based on a 12-mer lytic base unit (LBU) composed of Arg and Val residues and structured in an amphipathic α-helix (Deslouches et al., Reference Deslouches, Phadke, Lazarevic, Cascio, Islam, Montelaro and Mietzner2005). They show that the 24-residue LBU2 peptide is a potent AMP, and that substituting three Val by Trp on the hydrophobic face leads to enhanced antimicrobial activity against P. aeruginosa and S. aureus, without increasing its cytotoxicity in a selective toxicity assay.
Novel antimicrobial peptides (AMP) can be identified through combinatorial approaches. It is the case of the Ac-RRWWRF-NH2 sequence, which was identified by the screening of a combinatorial library (Blondelle and Houghten, Reference Blondelle and Houghten1996). In 2004, Dathe et al., showed that head-to-tail cyclisation (c-RW) led to enhanced antimicrobial activity (Dathe et al., Reference Dathe, Nikolenko, Klose and Bienert2004). Interestingly, Trp to Tyr substitutions abolished the antimicrobial activity in both linear and cyclic versions, consistent with what was observed with indolicidin and tritrpticin. On the other hand, the substitution of Trp by naphtyl-Ala led to enhanced antimicrobial activity, again consistent with what was observed with indolicidin. The same peptide with all D amino acids had comparable activity to the L peptide. Later, the same group showed that the analogue cyclo-RRRWFW (c-WFW) was even more active (Junkes et al., Reference Junkes, Wessolowski, Farnaud, Evans, Good, Bienet and Dathe2008). The modulation of the activity of c-WFW by substituting Trp by analogous residues was studied (Bagheri, Keller, and Dathe, Reference Bagheri, Keller and Dathe2011). Only the 5-fluoroTrp analogue was slightly more active than the original peptide, and the least hydrophobic analogues had significantly reduced activity. c-WFW is a membrane-targeted AMP, but it has an unusual mode of action. It is active against both Gram-positive and Gram-negative bacteria. In Gram-negative bacteria, it interacts with LPS, but its target is the cytoplasmic membrane. It appears that c-WFW does not exert its activity by permeabilising membranes, but rather by strongly partitioning in PE/CL domains (Scheinpflug et al., Reference Scheinpflug, Krylova, Nikolenko, Thurm and Dathe2015) and reducing membrane fluidity (Scheinpflug et al., Reference Scheinpflug, Wenzel, Krylova, Bandow, Dathe and Strahl2017). Recently, the substitution of Trp by a 3-hydroxychromone fluorophore was used to track c-WFW in cells and zebrafish embryo (Afonin et al., Reference Afonin, Koniev, Préau, Takamiya, Strizhak, Babii, Hrebonkin, Pivovarenko, Dathe, le Noble, Rastegar, Strähle, Ulrich and Komarov2021). The incorporation of the probe did not impair the antimicrobial activity of the peptide but led to a slight increase of haemolytic properties.
The group of Svendsen also studied Arg/Trp sequences, based on lactoferricin and the Ac-RRWWRF-NH2 presented above (Strøm et al., Reference Strøm, Rekdal and Svendsen2002). In 2003, in an attempt to identify the pharmacophore of cationic AMP, they designed multiple Arg/Trp sequences, which will be further discussed in the paragraph dedicated to systematic studies on Arg/Trp sequences. In this study, the authors identified the sequence RWRWRW-NH2 which presented interesting antibacterial activities against E. coli and S. aureus (Strøm et al., Reference Strøm, Haug, Skar, Stensen, Stiberg and Svendsen2003). This short simple peptide was further extensively studied. This peptide, also called MP196, is particularly efficient against Gram-positive bacteria, targets bacterial membranes and delocalises peripheral membrane proteins involved in respiration and cell-wall biosynthesis (Wenzel et al., Reference Wenzel, Chiriac, Otto, Zweytick, May, Schumacher, Gust, Bauke Albada, Penkova, Krämer, Erdmann, Metzler-Nolte, Straus, Bremer, Becher, Brötz-Oesterhelt, Sahl and Bandow2014). This peptide has a very fast bactericidal action and is promising for clinical applications, but unfortunately it is not efficient against all Gram-positive strains, in particular not all methicillin-resistant Staphylococcus aureus (MRSA), and is associated with toxicity towards erythrocytes in vivo (Wenzel et al., Reference Wenzel, Prochnow, Mowbray, Vuong, Höxtermann, Stepanek, Bauke Albada, Hall, Metzler-Nolte and Bandow2016a). Several chemical modifications of this peptide were proposed to modulate its properties. One of them is the introduction of metallocenes at the N-terminus (Chantson et al., Reference Chantson, Falzacappa, Crovella and Metzler-Nolte2006; Albada et al., Reference Albada, Prochnow, Bobersky, Langklotz, Schriek, Bandow and Metzler-Nolte2012) or by substituting Trp by ferrocene-modified lysines (Albada and Metzler-Nolte Reference Albada and Metzler-Nolte2017). These modifications did not impair the activity of MP196 against Gram-positive strains and enhanced its activity against Acinetobacter baumannii. Other modifications were studied, in particular, L-to-D substitutions did not impair the activity against MRSA but led to decreased haemolysis (Albada et al., Reference Albada, Prochnow, Bobersky, Langklotz, Bandow and Metzler-Nolte2013). Finally, lipidation of MP196 leads to enhanced activity, especially against Gram-negative bacteria, without impairing the mechanism of action of the peptide (Albada et al., Reference Albada, Prochnow, Bobersky, Langklotz, Schriek, Bandow and Metzler-Nolte2012; Wenzel et al., Reference Wenzel, Schriek, Prochnow, Bauke Albada, Metzler-Nolte and Bandow2016b).
Importance of the number and position of Trp
In CPP sequences
In many studies, increasing the number of Trp in a peptide sequence is accompanied by a clear improvement in the internalisation efficiency of the peptides. For example, as mentioned above, RW9 analogues with only one or two Trp residues (other Trp residues being substituted by Phe) are better internalised than RF9, but they are much less internalised than RW9 thus highlighting the major role of the number of Trp residue in a CPP sequence (Jobin et al., Reference Jobin, Blanchet, Henry, Chaignepain, Manigand, Castano, Lecomte, Burlina, Sagan and Alves2015).
However, there is no linear or direct relation between the number of Trp and the uptake efficiency. In a further study, RW9 nonapeptide analogues with varying Arg/Trp balance were designed (Walrant et al., Reference Walrant, Bauzá, Girardet, Alves, Lecomte, Illien, Cardon, Chaianantakul, Pallerla, Burlina, Frontera and Sagan2020). These peptides contain one (R8W), two (R7W2), three (R6W3) or four (R5W4) Trp residues. In this study, R7W2 analogues are the least internalised, showing that Arg residues can somehow compensate for Trp loss. The position of the Trp residues in the sequences was also studied. The peptides were designed so that they could adopt an amphipathic structure, like the original RW9, or not. Very surprisingly, the data suggested that an amphipathic secondary structure was not needed for efficient internalisation. In particular, an analogue of RW9 incorporating D-Trp that prevents the potential formation of a α-helical structure, was internalised to the same extent as the original all-L RW9. Finally, sequences with four Trp were more cytotoxic.
The group of Nordén has also extensively studied model Arg/Trp peptides. A study with oligoarginine sequences incorporating variable number of Trp residues showed a similar trend, that is an increase in the internalisation efficiency by increasing the number of Trp in the sequences either at the N-terminus, as a block in the middle of the sequence or scattered in the sequence (Rydberg et al., Reference Rydberg, Carlsson and Nordén2012a, Reference Rydberg, Matson, Åmand, Esbjörner and Nordén2012b, Reference Rydberg, Kunze, Carlsson, Altgärde, Svedhem and Nordén2014). Increasing the number of Trp in the sequence globally led to an increase in internalisation properties, but this is also highly dependent of the position of the Trp in the sequences. Indeed, a block of three Trp residues in the middle of the sequence leads to higher internalisation than four Trp at the N-terminus. The most efficient combination is the one in which the Trp residues are scattered in the sequence (RWmix), suggesting that Trp backbone spacing is an important parameter. Again, interestingly, an increased number of Trp was associated with increased cytotoxicity, especially when present as a block at the N-terminus.
Model Arg/Trp sequences were also studied by Shirani et al. (Shirani et al., Reference Shirani, Mojarrad, Farkhani, Khosroshahi, Zakeri-Milani, Samadi, Sharifi, Mohammadi and Valizadeh2015). In this study, block peptides were designed, with either 2 or 3 Trp blocks flanking 4 Arg (W 2R 4W 2, W 3R 4W 3) or the opposite, with Arg flanking a block of 4 Trp (R 2W 4R 2 or R 3W 4R 3). In this study, the peptides with a central block of Trp are better internalised than the ones with central Arg, consistent with what was observed by Rydberg et al. (Rydberg et al., Reference Rydberg, Carlsson and Nordén2012a, Reference Rydberg, Matson, Åmand, Esbjörner and Nordén2012b). The peptide with flanking blocks of two Trp, W 2R 4W 2 is better internalised than W 3R 4W 3. The authors also explain that a simple comparison of the amphipathic nature of the different sequences cannot explain the observed differences in cellular uptake.
In the WRAP peptides series mentioned previously, Trp residues can be either scattered (WRAP1) or clustered (WRAP4,5 and 6) in the sequence, as in the RW peptides designed by Rydberg et al., The authors suggest that Trp play an essential role for nanoparticle-based nuclei acid delivery and have to be clustered, either in the primary structure or in the secondary structure, upon folding (Konate et al., Reference Konate, Dussot, Aldrian, Vaissière, Viguier, Neira, Couillaud, Vivès, Boisguerin and Deshayes2019).
Finally, cyclic peptides composed of an increasing number of RW motifs, [RW]n, with n = 5, 6, 7, 8 or 9, were tested for their ability to promote the cell uptake of a fluorescent phosphopeptide (Hanna et al., Reference Hanna, Mozaffari, Tiwari and Parang2018). The authors show that increasing the number of units leads to better cell penetration properties and slightly increased cytotoxicity, even though all peptides are not cytotoxic at low concentrations. Very recently, the same team designed hybrid cyclic/linear peptides, based on [RW]5 to which a linear (RW)n tail, where n = 1, 2, 3, 4 or 5, is grafted on the side chain of a Lys. The peptide with the longest tail, [(RW)5K](RW)5 was the most efficient to deliver a fluorescent phosphopeptide.
Taken together, these studies show that it is yet extremely difficult to rationalise the design of Arg/Trp peptides for efficient internalisation, and that, in addition to Arg/Trp balance and Trp position, many other parameters such as sequence length or global charge which have not been discussed here are to be taken into account.
In AMP sequences
As described above, many AMPs have Trp residues which are critical for their efficient antimicrobial activity. As for CPPs, several research groups systematically studied the antimicrobial properties of rationally designed Arg/Trp peptides.
In 2003, the group of Svendsen studied several Arg/Trp trimers to hexamers (Strøm et al., Reference Strøm, Haug, Skar, Stensen, Stiberg and Svendsen2003). They showed that the trimer WRW-NH2 was slightly more active than RWR-NH2 against S. aureus. Longer peptides were globally more active. The two most active sequences were RWRWRW-NH2 (described above) and RRRWWW-NH2. The authors suggest that peptides with a Trp at the C-terminus are globally more active. Another interesting point is that the dipeptide RW-OBzl still retains very good activity against S. aureus. A few years later, the same group showed that the minimalistic RTbtR-NH2 tripeptide was active against Gram-negative E. coli and P. aeruginosa, and very active against S. aureus and multiresistant staphylococci strains, and non haemolytic (Haug et al., Reference Haug, Stensen, Kalaaji, Rekdal and Svendsen2008). Longer RW sequences (RW)n, where n = 1, 2, 3, 4, 5, 6 or 8, were studied by other groups (Liu et al., Reference Liu, Brady, Young, Rasimick, Chen, Zhou and Kallenbach2007; Phambu et al., Reference Phambu, Almarwani, Garcia, Hamza, Muhsen, Baidoo and Sunda-Meya2017). Increasing peptide length is associated to increased antimicrobial properties and haemolysis. The (RW)3 sequence appears as the best compromise in terms of antimicrobial activity versus haemolysis, and ease of synthesis.
Multivalency effects were also tested, by grafting short azide-modified RW sequences on alkyne-modified, mono, di or trivalent benzene scaffolds through copper-catalysed alkyne-azide cycloaddition. Synergistic effects of the trivalent display were observed for short (2 or 3 residues) RW sequences, whereas it was not the case for longer peptides (Hoffknecht et al., Reference Hoffknecht, Worm, Bobersky, Prochnow, Bandow and Metzler-Nolte2015).
The Arg/Trp CPPs studied by the group of Nordén and presented above (Table 4) were also evaluated for their antimicrobial properties (Rydberg et al., Reference Rydberg, Carlsson and Nordén2012a). None of them presented significant activity on Gram-negative bacteria, but were efficient against Gram-positive S. aureus and S. pyogenes. For S. aureus, W4R8 and RWR, thus presenting a block of 4 Trp were the most efficient. Not surprisingly, WR8 was the least efficient of all sequences.
Table 4. List of systematic studies on Arg/Trp-rich CPP sequences
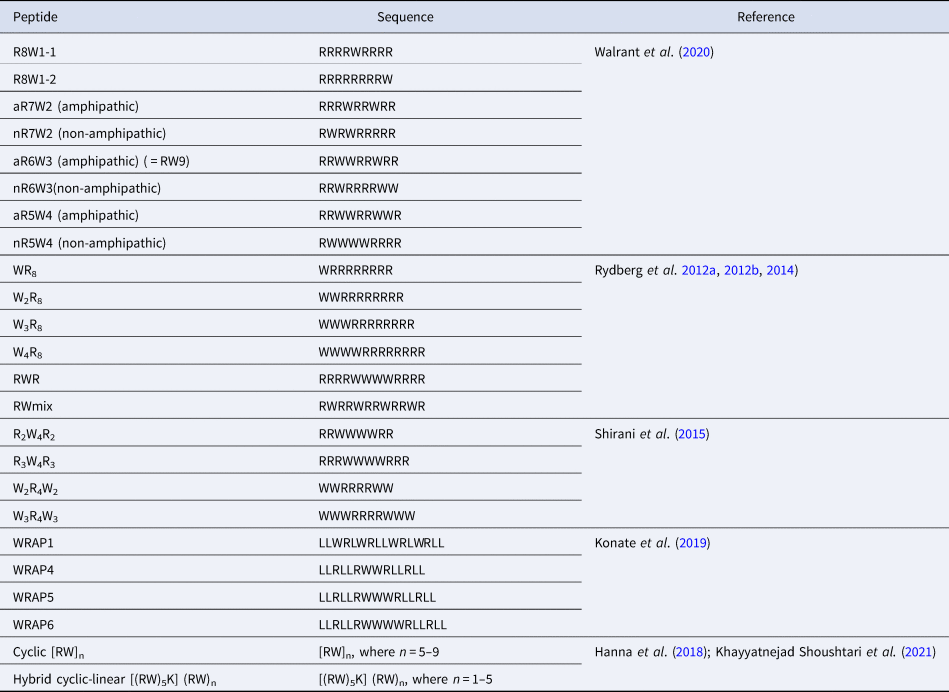
Regarding linear peptides, very recently, Clark et al., proposed a systematic study of all Arg/Trp combinations in peptides ranging from 1 to 7 residues (Clark et al., Reference Clark, Jowitt, Harris, Knight and Dobson2021). Globally, their peptides were more active on Gram-positive S. aureus compared to Gram-negative P. aeruginosa. A minimal length of 4–5 residues is required to observe antimicrobial activity. For all organisms, they observe a peak activity for a content of 60% Trp. Finally, they observe that adjacent Trp duplets or triplets confer enhanced antimicrobial activity, consistent with what was observed with other peptides described above.
Finally, the effect of cyclisation of Arg/Trp peptides was also studied. R4W4 and R4W3 were tested for their antimicrobial activity against MRSA and P. aeruginosa in their linear and cyclic versions (Oh et al., Reference Oh, Sun, Shirazi, Laplante, Rowley and Parang2014). Cyclic versions were always more active than linear versions and peptides with 4 Trp were more efficient than with 3 Trp. Cyclic [R4W4] was particularly efficient against MRSA and also showed CPP properties. A few years later, the same group studied the substitution of Trp residues by other aromatic residues or N-Methyl-Trp (Riahifard et al., Reference Riahifard, Mozaffari, Aldakhil, Nunez, Alshammari, Alshammari, Yamaki, Parang and Tiwari2018), which always led to a decrease in antimicrobial activity, showing the critical role of Trp. They also increased the size of the macrocycle with [R7W5], [R7W6] and [R7W7], but these larger peptides were less active than the original [R4W4]. Interestingly, these cyclic AMPs were designed by the same group as the cyclic CPPs described above. The ‘block’ design was privileged for AMPs whereas the alternate RW designed was privileged for CPPs.
Trp-containing CPPs and polysaccharides
Polysaccharides as cell-surface partners
The impact of cell-surface oligosaccharides on the internalisation of various CPPs in cells has been widely studied by numerous research teams world-wide (Console et al., Reference Console, Marty, García-Echeverría, Schwendener and Ballmer-Hofer2003; Fuchs and Raines, Reference Fuchs and Raines2004; Marty et al., Reference Marty, Meylan, Schott, Ballmer-Hofer and Schwendener2004; Coupade et al., Reference Coupade, Fittipaldi, Chagnas, Michel, Carlier, Tasciotti, Darmon, Ravel, Kearsey, Giacca and Cailler2005; Gonçalves et al., Reference Gonçalves, Kitas and Seelig2005; Mano et al., Reference Mano, Teodósio, Paiva, Simões and Pedroso de Lima2005; Magzoub et l., Reference Magzoub, Pramanik and Gräslund2005; Ziegler et al., Reference Ziegler, Nervi, Dürrenberger and Seelig2005; Nascimento et al., Reference Nascimento, Hayashi, Kerkis, Oliveira, Oliveira, Rádis-Baptista, Nader, Yamane, dos Santos Tersariol and Kerkis2007; Poon and Gariépy, Reference Poon and Gariépy2007; Lundin et al., Reference Lundin, Johansson, Guterstam, Holm, Hansen, Langel and Andaloussi2008; Ram et al., Reference Ram, Aroui, Jaumain, Bichraoui, Mabrouk, Ronjat, Lortat-Jacob and De Waard2008; Jiao et al., Reference Jiao, Delaroche, Burlina, Alves, Chassaing and Sagan2009; Nakase et al., Reference Nakase, Hirose, Tanaka, Tadokoro, Kobayashi, Takeuchi and Futaki2009; Letoha et al., Reference Letoha, Keller-Pintér, Kusz, Kolozsi, Bozsó, Tóth, Vizler, Oláh and Szilák2010; Alves et al., Reference Alves, Bechara, Walrant, Zaltsman, Jiao and Sagan2011; Lécorché et al., Reference Lécorché, Walrant, Burlina, Dutot, Sagan, Mallet, Desbat, Chassaing, Alves and Lavielle2012; Subrizi et al., Reference Subrizi, Tuominen, Bunker, Róg, Antopolsky and Urtti2012; Bechara et al., Reference Bechara, Pallerla, Zaltsman, Burlina, Alves, Lequin and Sagan2013; Fang et al., Reference Fang, Fan, Fu, Chen, Hwang, Hung, Lin and Chang2013b; Verdurmen et al., Reference Verdurmen, Wallbrecher, Schmidt, Eilander, Bovee-Geurts, Fanghänel, Bürck, Wadhwani, Ulrich and Brock2013; Favretto et al., Reference Favretto, Wallbrecher, Schmidt, Van De Putte and Brock2014; Tchoumi Neree et al., Reference Tchoumi Neree, Nguyen, Chatenet, Fournier and Bourgault2014; Wallbrecher et al., Reference Wallbrecher, Verdurmen, Schmidt, Bovee-Geurts, Broecker, Reinhardt, van Kuppevelt, Seeberger and Brock2014; Bechara et al., Reference Bechara, Pallerla, Burlina, Illien, Cribier and Sagan2015; Kawaguchi et al., Reference Kawaguchi, Takeuchi, Kuwata, Chiba, Hatanaka, Nakase and Futaki2016; Pae et al., Reference Pae, Liivamägi, Lubenets, Arukuusk, Langel and Pooga2016; Takechi-Haraya et al., Reference Takechi-Haraya, Aki, Tohyama, Harano, Kawakami, Saito and Okamura2017; Walrant et al., Reference Walrant, Bauzá, Girardet, Alves, Lecomte, Illien, Cardon, Chaianantakul, Pallerla, Burlina, Frontera and Sagan2020). Since most CPPs are cationic sequences and oligo- and polysaccharides mainly anionic, peptide/oligosaccharide complexes are easily formed through electrostatic interactions at the cell-surface. For most CPPs, some studies reported that this first interaction step with polysaccharides triggers internalisation through different endocytosis pathways (Ziegler, Reference Ziegler2008; Alves et al., Reference Alves, Jiao, Aubry, Aussedat, Burlina, Chassaing and Sagan2010; Wittrup et al., Reference Wittrup, Zhang and Belting2011). For other CPPs however, it was suggested that their interaction with anionic oligosaccharides leads to the capture of the peptide at the cell-surface without any link with its subsequent internalisation in cells (Fuchs and Raines, Reference Fuchs and Raines2004; Verdurmen et al., Reference Verdurmen, Wallbrecher, Schmidt, Eilander, Bovee-Geurts, Fanghänel, Bürck, Wadhwani, Ulrich and Brock2013), or even that this capture by polysaccharides prevents further internalisation (Alves et al., Reference Alves, Bechara, Walrant, Zaltsman, Jiao and Sagan2011; Walrant et al., Reference Walrant, Bechara, Alves and Sagan2012a). It is worth mentioning that various types of oligosaccharides (sialic acids, hyaluronic acids, heparan sulphates, chondroitin sulphates etc.) have been identified to play these different or opposite roles in the internalisation process of CPPs.
As an important additional result, it should be underlined that beside the convergent role of GAGs in endocytosis, a few studies also recently highlighted their role in a non-endocytotic internalisation pathway of CPPs, that is through direct translocation at the plasma membrane (Bechara et al., Reference Bechara, Pallerla, Burlina, Illien, Cribier and Sagan2015; Pae et al., Reference Pae, Liivamägi, Lubenets, Arukuusk, Langel and Pooga2016; Takechi-Haraya et al., Reference Takechi-Haraya, Aki, Tohyama, Harano, Kawakami, Saito and Okamura2017; Takechi-Haraya and Saito, Reference Takechi-Haraya and Saito2018). This role of GAGs in the translocation mechanism implies a cross-talk between the peptide, the lipid bilayer and cell-surface GAGs as recently proposed for homeoproteins (Cardon et al., Reference Cardon, Bolbach, Hervis, Lopin-Bon, Jacquinet, Illien, Walrant, Ravault, He, Molina, Burlina, Lequin, Joliot, Carlier and Sagan2021). In this study, at a temperature at which endocytotic pathways are all inhibited (4 °C), internalisation is reduced in GAG-deficient cells compared to wild-type ones, an observation that clearly supports the role of GAGs in the translocation process. Interestingly, homeoproteins for which internalisation properties have been demonstrated all share similar contents in basic and hydrophobic amino acids, and in particular, a central Trp-Phe dipeptide motif is highly conserved (Sagan et al., Reference Sagan, Burlina, Alves, Bechara, Dupont and Joliot2013; Di Nardo et al., Reference Di Nardo, Fuchs, Joshi, Moya and Prochiantz2018) (Table 2).
As described above, different studies reported that Trp is a crucial amino acid for CPP internalisation (Derossi et al., Reference Derossi, Joliot, Chassaing and Prochiantz1994; Fischer et al., Reference Fischer, Zhelev, Wang, Melville, Fåhraeus and Lane2000; Dom et al., Reference Dom, Shaw-Jackson, Matis, Bouffioux, Picard, Prochiantz, Mingeot-Leclerq, Brasseur and Rezsohazy2003; Letoha et al., Reference Letoha, Gaál, Somlai, Czajlik, Perczel and Penke2003; Letoha et al., Reference Letoha, Gaál, Somlai, Czajlik, Perczel and Penke2003; Lindberg et al., Reference Lindberg, Biverståhl, Gräslund and Mäler2003; Christiaens et al., Reference Christiaens, Grooten, Reusens, Joliot, Goethals, Vandekerckhove, Prochiantz and Rosseneu2004; Walrant et al., Reference Walrant, Correia, Jiao, Lequin, Bent, Goasdoué, Lacombe, Chassaing, Sagan and Alves2011; Bechara et al., Reference Bechara, Pallerla, Zaltsman, Burlina, Alves, Lequin and Sagan2013; Bechara et al., Reference Bechara, Pallerla, Burlina, Illien, Cribier and Sagan2015).
In this context, Trp must be seen as a singular amino acid in terms of its interactions with cell-surface components (Khemaissa et al., Reference Khemaissa, Sagan and Walrant2021). Trp is indeed unique among the natural amino acids. As described above, Trp has a hydrophobic character but can also be engaged in many types of interactions (Fig. 3b), such as π–cation, hydrogen-bonds and more recently described, ion-pair π interactions (Walrant et al., Reference Walrant, Bauzá, Girardet, Alves, Lecomte, Illien, Cardon, Chaianantakul, Pallerla, Burlina, Frontera and Sagan2020). All these interactions are of particular importance for the cross-talk mentioned above between the peptide, the lipid bilayer and cell-surface GAGs, and further internalisation of the peptides. We further detail below how Trp in a peptide sequence impacts the thermodynamics of the interaction and the internalisation pathways, as well as the peptide conformation and structure in interaction with cell-surface components.
Thermodynamics CPPs/ GAGs complexes
Interaction of cationic CPPs with GAGs is ensured by electrostatic interactions but also by hydrogen-bonds and hydrophobic interactions resulting in the formation of clusters as demonstrated by heparin affinity chromatography, clustering experiments, dynamic light scattering (DLS) and microscopy analyses (Åmand et al., Reference Åmand, Rydberg, Fornander, Lincoln, Nordén and Esbjörner2012). It is known that Trp in proteins can form clusters through hydrophobic interactions (Klein-Seetharaman et al., Reference Klein-Seetharaman, Oikawa, Grimshaw, Wirmer, Duchardt, Ueda, Imoto, Smith, Dobson and Schwalbe2002). As already mentioned, thanks to its particular structure, Trp can be involved in both hydrophobic interactions and electrostatic interactions (Khemaissa et al., Reference Khemaissa, Sagan and Walrant2021). For instance, it can interact with positive partial charges from carbohydrates C-H protons through C-H-π bonds (Kiessling and Diehl, Reference Kiessling and Diehl2021). This suggests a better interaction between Trp and polarised C-H sugar bonds such as β-galactose whereas less polarised carbohydrates including α-mannose are not able to establish such interactions. Cation-π is the only interaction that does not directly involve Trp and GAGs. However, it can occur between Trp and a basic residue (in general Arg) belonging to the peptide that increases the pKa of the guanidinium. Consequently, Arg is mainly found in the protonated form that strengthens the electrostatic interaction with GAGs.
As seen above, Trp has a strong impact on the uptake efficiency depending on its occurrence and its position in the sequence of a peptide. The number of Trp in CPP sequences has a major contribution in the peptide affinity for GAGs whereas its position has little effect on thermodynamic parameters according to isothermal titration calorimetry (ITC) and nuclear magnetic resonance (NMR) experiments (Bechara et al., Reference Bechara, Pallerla, Zaltsman, Burlina, Alves, Lequin and Sagan2013). Indeed, an increase in the number of Trp in cationic peptides differing from each other in size and amino acid content, translates into a linear increase in the free energy in the interaction with heparin, monosulphated chondroitin 4-sulphated chondroitin sulphate (CS-A) (4S-CS) and CS-C (6S-CS) and disulphated chondroitin 2,6-sulphated chondroitin sulphate (CS-D) (2S, 6S-CS) and 4,6-sulphated chondroitin sulphate (CS-E) (4S,6S-CS). This enhancement of the Gibbs free energy includes both an enthalpic and an entropic contribution. On the one hand, a favourable enthalpy increase is observed when increasing the number of Trp, whereas no straightforward relationship is observed with the number of positive charge changes. This result suggests an involvement of other types of interactions than simple electrostatic interactions in the formation of peptide / GAGs complexes. On the other hand, the increase in the number of Trp leads to an increase in defavourable entropy. At a first glance, one could expect the entropy to be favourable since the interaction of peptides with GAGs causes the release of water molecules initially bound to GAGs and/or peptides. However, the unfavourable entropy coming from the conformational penalty should also be considered. The binding of the peptide to GAGs limits the number of degrees of freedom of the molecules and, consequently restricts the number of their possible conformations, thus impacting the binding entropy. In addition, interaction with GAGs restricts Trp rotational dynamic, thus also explaining the unfavourable entropy (Takechi-Haraya et al., Reference Takechi-Haraya, Nadai, Kimura, Nishitsuji, Uchimura, Sakai-Kato, Kawakami, Shigenaga, Kawakami, Otaka, Hojo, Sakashita and Saito2016).
The enhancement of Gibbs free energy was obvious for Trp-containing peptides which could lead to stable aggregated complexes with GAGs. Interestingly, Rullo et al., reported that Penetratin–heparin aggregates or clusters are thermodynamically and kinetically more stable than Tat–heparin ones (Rullo et al., Reference Rullo, Qian and Nitz2011). Tat contains no Trp, whereas Penetratin has two Trp.
Concerning structural aspects, cationic peptides containing Trp interact with and form β-strand or β-sheet organised aggregates whereas in the absence of Trp, α-helices are observed without aggregate formation (Bechara et al., Reference Bechara, Pallerla, Zaltsman, Burlina, Alves, Lequin and Sagan2013). In β-strand peptides, hydrogen bonds are more energetic than for α-helical peptides, which could explain then the strong enthalpy measured by ITC. (Bechara et al., Reference Bechara, Pallerla, Zaltsman, Burlina, Alves, Lequin and Sagan2013).
The interactions of Trp-containing CPPs with GAGs were further studied within a series of nonapeptides containing only Arg (5 to 9) and Trp (0 to 4) (Table 4) (Walrant et al., Reference Walrant, Bauzá, Girardet, Alves, Lecomte, Illien, Cardon, Chaianantakul, Pallerla, Burlina, Frontera and Sagan2020). The study confirmed the increase in the favourable enthalpy and defavourable entropy according to the number of Trp in the sequence. In addition, density functional theory (DFT) analyses clearly demonstrated that ionpair-π interactions between Trp (indole), Arg (guanidinium) and the sulphate or carboxylate groups of carbohydrates in GAGs could enhance the free energy of the complex, thus the time of residence of the peptide in interaction with GAGs. In addition, enhancing the number of Trp also resulted in an increase in the binding stoichiometry. Interestingly, similar effects on enthalpy were also recorded for the interaction of the same peptide sequences with unilamellar vesicles composed of negatively charged PG. In this case, the entropy was favourable but decreased with the number of Trp. The presence of Trp is fundamental for peptide insertion inside the membrane lipid bilayer, as shown by Trp substitution with Arg, even if the interaction enthalpy with GAGs increased. Of utmost interest, the introduction of D-Trp in the nonapeptide sequence, which impairs the structuration of the peptide, had no impact on the interactions of the resulting peptide with lipids and GAGs. This result supports the idea already suggested that a well-defined secondary structure, in particular one displaying facial amphiphilicity, is not an important feature for CPP internalisation and interaction with membrane partners (Derossi et al., Reference Derossi, Calvet, Trembleau, Brunissen, Chassaing and Prochiantz1996), at least in this series of Arg/Trp peptides.
The presence of Trp in a peptide sequence does not necessarily imply a direct interaction with GAGs only, and other interaction partners should be considered such as lipids. Indeed, a study on the interactions of the peptide GBP (NYRWRCKNQN) with heparin and dodecylphosphocholine (DPC) micelles by NMR and fluorescence measurements suggest no influence of the polysaccharide on lipid/Trp interaction (Hung et al., Reference Hung, Jiang, Chen, Lu, Hsieh, Kuo, Hung, Wang, Chang and Sue2017). Interestingly, Trp is a crucial amino acid for membrane insertion of GBP, as its replacement with Arg abolishes cell penetration, although it significantly improves the heparin-binding capacity of the peptide. Hung and co-workers concluded that Trp4 is responsible for lipid insertion, and Arg5 and Lys7 for GAG binding. However, in our current understanding, a Trp/Arg interaction promoting peptide / GAG complexes through ion-pair π interactions and cell internalisation (Walrant et al., Reference Walrant, Bauzá, Girardet, Alves, Lecomte, Illien, Cardon, Chaianantakul, Pallerla, Burlina, Frontera and Sagan2020) cannot be excluded in this case.
Consequences on internalisation pathways
All these interactions with GAGs constitute the first step for CPP internalisation through an endocytic pathway (Bechara et al., Reference Bechara, Pallerla, Zaltsman, Burlina, Alves, Lequin and Sagan2013; Bechara and Sagan, Reference Bechara and Sagan2013; Walrant et al., Reference Walrant, Bauzá, Girardet, Alves, Lecomte, Illien, Cardon, Chaianantakul, Pallerla, Burlina, Frontera and Sagan2020). The implication of GAGs in the endocytic uptake has been reported in many studies (Belting et al., Reference Belting, Mani, Jönsson, Cheng, Sandgren, Jonsson, Ding, Delcros and Fransson2003; Poon and Gariépy, Reference Poon and Gariépy2007; Sarrazin et al., Reference Sarrazin, Wilson, Sly, Tor and Esko2010). They are also involved in cargo delivery by CPPs, including proteins, DNA and small fluorescent molecules (Console et al., Reference Console, Marty, García-Echeverría, Schwendener and Ballmer-Hofer2003; Wadia et al., Reference Wadia, Stan and Dowdy2004; Wittrup et al., Reference Wittrup, Zhang, ten Dam, van Kuppevelt, Bengtson, Johansson, Welch, Mörgelin and Belting2009). Their role in PDX-1 transduction has also been highlighted (Ueda et al., Reference Ueda, Matsumoto, Hayashi, Kobayashi and Noguchi2008). The direct or signalling role of GAGs in drug delivery vector has also been reviewed (Favretto et al., Reference Favretto, Wallbrecher, Schmidt, Van De Putte and Brock2014).
A comparison between Tp and Tp10 that does not contain a Trp residue shows that Tp has a better interaction with GAGs and can reorganise the membrane of GPMVs in order to form GAG clusters thanks to its Trp residue (Pae et al., Reference Pae, Liivamägi, Lubenets, Arukuusk, Langel and Pooga2016).
There is a favourable relationship between the binding stoichiometry and the uptake efficiency in the case of Trp-containing cationic peptides (Bechara et al., Reference Bechara, Pallerla, Zaltsman, Burlina, Alves, Lequin and Sagan2013; Favretto et al., Reference Favretto, Wallbrecher, Schmidt, Van De Putte and Brock2014). This tendency appears to be specific to amphipathic peptides because cationic peptide translocation across the plasma membrane does not seem to be GAG-dependent (Pae et al., Reference Pae, Liivamägi, Lubenets, Arukuusk, Langel and Pooga2016). Furthermore, a negative correlation between the binding stoichiometry peptide/GAG complexes and the peptide uptake efficiency has been pointed out (Wallbrecher et al., Reference Wallbrecher, Verdurmen, Schmidt, Bovee-Geurts, Broecker, Reinhardt, van Kuppevelt, Seeberger and Brock2014).
Amphipathic CPP clustering with HS does not always lead to a better internalisation (Verdurmen et al., Reference Verdurmen, Wallbrecher, Schmidt, Eilander, Bovee-Geurts, Fanghänel, Bürck, Wadhwani, Ulrich and Brock2013). In order to better understand the role of clusters formed with GAGs in the uptake mechanism, Arg and Trp residues have been pegylated in WR9. This way, no cluster can be formed with HS due to steric hindrance. This study highlighted an endocytic pathway for the pegylated peptide, whereas both endocytosis and translocation are observed depending on the peptide concentration for WR9. Thus, HS clustering does not seem to always be required for endocytosis (Ziegler and Seelig Reference Ziegler and Seelig2011).
All these results are consistent with complex mechanisms involving GAGs. One can assume that in the absence of possible clustering, other ways of endocytosis involving GAGs without clustering can be triggered. Many studies are in progress to elucidate the different uptake mechanisms and interactions involving CPPs.
In conclusion, thanks to GAG interactions, Trp in cationic sequences can be possibly involved in direct plasma membrane translocation and endocytosis of CPPs.
Trp-containing AMPs and glycoconjugates
Glycoconjugates and cationic AMPs activity
As for CPPs and the extracellular matrix, AMPs first meet negatively charged LPS in Gram-negative and lipoteichoic acid (LTA)/wall teichoic acid (WTA) interweaved in peptidoglycan in Gram-positive bacteria. This first interaction has thus to be considered since it contributes to the antimicrobial activity of the peptides.
It has been indeed reported for some AMP that are inactive against Gram-negative bacteria that the outer membrane and LPS represents a major barrier that prevents the peptides to be active (Papo et al., Reference Papo, Oren, Pag, Sahl and Shai2002; Papo and Shai, Reference Papo and Shai2005). In their study, Papo and Shai have for instance studied the antimicrobial dodecapeptide (K5L7) and its diastereomer (4D-K5L7), which contains four D-aminoacids preventing any well-defined folding (α-helix, β-strand etc.) of the peptide. The authors reported that the two peptides bind LPS and depolarise the bacteria membrane potential, but that only the D-aminoacid containing diastereomer kills Gram-negative bacteria. The authors could establish that K5L7 first binds LPS and that this interaction facilitates self-association of the peptide, which prevents further crossing of the lipid bilayer, likely because the aggregated peptide is no longer accessible for interactions with the lipid bilayer. In contrast, the 4D-K5L7 peptide stays as monomers once bound to LPS. The monomeric state of the peptide facilitates its accumulation on the surface of the lipid bilayer until a threshold concentration is reached at which the peptide can induce pores via micelles formation and cell death (Papo and Shai, Reference Papo and Shai2005).
It was also shown from the bacterial wall point of view that chemical modifications of lipid A that lead to removal or decrease of the net negative charge of the bacteria wall, reduce the killing activity of AMP (Needham and Trent, Reference Needham and Trent2013). In addition, when the LPS of bacteria Helicobacter pylori is modified to alter the charge of the outer membrane, the bacteria became highly resistant to AMP such as polymixins secreted by the host cells (Cullen et al., Reference Cullen, Giles, Wolf, Ecobichon, Boneca and Trent2011).
A calorimetric study of the interaction of polymyxin B with different bacterial strains showed a direct correlation between the minimal inhibitory concentration and the heat produced by the interaction of the peptide with the surface of bacterial cells (Howe, Hammer, and Brandenburg, Reference Howe, Hammer and Brandenburg2007). The authors suggest this is directly linked with the different net charge carried by the core oligosaccharide and lipid A.
Regarding Gram-positive bacteria, peptidoglycan and LTA have been reported to act as either peptide ‘sponges’, increasing their local concentration, or as peptide ‘traps’, keeping them away from the lipid membrane (Malanovic and Lohner, Reference Malanovic and Lohner2016a). A recent study strongly suggested that WTA and LTA strongly impeded the access of variable molecules to the surface of S. aureus membrane (Ferraro et al., Reference Ferraro, Kim, Im and Pires2021).
Trp impact on cationic AMP interactions
Melittin (GIGAVLKVLTT11GL13PALISW19IKRKRQQ26) is a well-studied bee venom toxin peptide that has antimicrobial activity against both Gram-negative and Gram-positive bacteria. The binding of melittin with LPS and lipid A has been studied by ITC, NMR and fluorescence spectroscopy (Bhunia et al., Reference Bhunia, Domadia and Bhattacharjya2007). Bhunia and coauthors reported that the C-terminal part (from residues 13 to 26) of the peptide adopts a helical conformation in interaction with LPS. Saturation transfer difference (STD) NMR studies reveal in particular the close proximity of Trp19 to LPS. Trp19 is in addition exposed to the solvent in the absence of LPS and becomes buried in the melittin-LPS complex. The interaction of the peptide with Lipid A was found favourable and entropically driven. Bhunia and coauthors suggested the involvement of hydrophobic interactions in the formation of the complex. The interfacial properties of Trp are likely crucial to allow these hydrophobic interactions with the lipid moiety of Lipid A, although other interactions could be involved as well, as described previously. Of utmost interest, in aqueous solvent or in complex with lipids, melittin adopts helical structures at its N- and C-termini with a kink at residues Thr11 and Gly12 (Terwilliger and Eisenberg, Reference Terwilliger and Eisenberg1982). Thus, AMPs adopt different structures and conformations depending on the membrane component they bind to.
The role of aromatic clusters in the interaction of cyclo-FWF (Table 4) with LPS was studied by ITC and membrane-partionning assays, using peptide analogues incorporating modified Trp residues (Bagheri et al., Reference Bagheri, Keller and Dathe2011). The authors showed stronger partionning of peptides in 1-palmitoyl-2-oleoyl-sn-glycero-3-phosphocholine (POPC)-smooth LPS (complete native LPS) compared to POPC-rough LPS (lacking O-antigen), demonstrating a key role of outer oligosaccharide for peptide interaction. The author also showed that reduced hydrophobicity was associated with reduced binding. Enhanced hydrogen-bonding abilities and dipole moment were associated with enhanced enthalpic contributions to binding. Finally, low salt dependency strongly supported a minor role of electrostatics in the LPS/cyclo-WFW interaction.
Recently, Wang and coworkers designed a series of Trp-rich peptides based on the template (VKKX)4, to obtain paired Trp at the i and i + 4 position on the backbone of peptides (where X is W, A, or L), and to study the effect of intramolecular aromatic interactions on the bioactivity of these AMPs (Wang et al., Reference Wang, Li, Li, Li, Shang, Chou, Lyu and Shan2021). The peptides contain either 2 Trp, (VKKWVKKAVKKWVKKA; VKKWVKKWVKKAVKKA), 3 Trp (VKKWVKKWVKKAVKKW; VKKWVKKWVKKWVKKA), or 4 Trp, (VKKWVKKWVKKWVKKW). Interestingly, the peptide containing 4 Trp was the most active against a panel of Gram-negative and Gram-positive bacteria. In parallel, this peptide was also the more efficient to bind LPS, as demonstrated by a fluorescence-based displacement assay, even better than melittin.
In another study, it was found again that Arg- and Trp-rich sequences (H-RRWTWWWTWRR-NH2 and H-RRWSWWWSWRR-NH2) have a broad spectrum of antimicrobial activity against Gram-negative and Gram-positive bacteria (Wang et al., Reference Wang, Li, Li, Li, Shang, Chou, Lyu and Shan2021). Regarding their interactions with cell wall components, the two peptides interact with both LPS and LTA, although their affinity seems to be lower than melittin.
All these studies show that cationic AMPs containing Trp are good binders of negatively charged components of the cell wall. Although the type of interactions that are involved are not fully described, the capacity of a peptide sequence to bind such cell wall components seems to be useful and must be considered to predict the antimicrobial ability of a peptide, and consequently to design new putative AMP sequences.
Trp in the interactions of peptides with lipids
Beside polysaccharides and glycoconjugates, the favourable interaction between Trp and lipids has also been reported and probed by NMR, ultraviolet resonance Raman, fluorescence spectroscopy, molecular modelling etc, and is generally very well documented. At the level of the lipid headgroup, this interaction generally consists in a cation-π interaction involving the indole ring, a hydrogen bond between the indole-NH and the lipid phosphate group and carbonyl-cation interactions (Sanderson and Whelan, Reference Sanderson and Whelan2004; Chan et al., Reference Chan, Prenner and Vogel2006; Sanderson, Reference Sanderson2007; Sanchez et al., Reference Sanchez, Kang, Wu and Kim2011). NMR and hydrogen/deuterium exchange in combination with mass spectrometry highlighted a strong positional preference of Trp near the lipid carbonyl (De Planque et al., Reference De Planque, Bonev, Demmers, Greathouse, Koeppe, Separovic, Watts and Killian2003). Trp is also able to be involved in cation-π interactions with positively charged lipids (Aliste et al., Reference Aliste, MacCallum and Peter Tieleman2003; Sanchez et al., Reference Sanchez, Kang, Wu and Kim2011). Therefore, Trp localisation at the membrane interface is thermodynamically favourable (White and Wimley, Reference White and Wimley1999).
With anionic lipids, a first electrostatic interaction leads to cationic peptide accumulation at the lipid membrane surface. Consequently, peptide/peptide oligomer charge repulsion generates membrane destabilisation and flips the peptide inside the hydrophobic core to avoid this repulsion. Finally, cation-π interactions with Arg residues or lipid headgroups partially mask the charge of cationic peptides, making its internalisation more favourable (Herce et al., Reference Herce, Garcia and Cristina Cardoso2014; Jobin et al., Reference Jobin, Blanchet, Henry, Chaignepain, Manigand, Castano, Lecomte, Burlina, Sagan and Alves2015).
Trp is able to insert deeper inside the hydrophobic core where it is located below the carbonyl region of bilayer lipids but, one or two hydrogen bonds with the water at the interface remain as illustrated by molecular dynamics simulations (Aliste et al., Reference Aliste, MacCallum and Peter Tieleman2003). Interestingly, photolabeling approaches can help deciphering the insertion depth of Trp-containing cationic peptides in lipid models (Jiao et al., Reference Jiao, Sachon, Alves, Chassaing, Bolbach and Sagan2017; Bechtella et al., Reference Bechtella, Kirschbaum, Cosset, Clodic, Matheron, Bolbach, Sagan, Walrant and Sachon2019). In the AMP bacteriocin BM21, the Trp residue located at the N-terminal position seems to drive the interaction with lipids through hydrophobic interactions as suggested by molecular dynamic simulations (Shepherd et al., Reference Shepherd, Vogel and Peter Tieleman2003). These hydrophobic interactions are at the origin of Trp preferential location at PC lipid interface as well as in octanol/water system usually used to determine hydrophobic scales (Wimley and White, Reference Wimley and White1996). Finally, hydrophobic interactions represent about 60 to 70% of total interactions occurring at the bilayer of which 13% involves Trp residues interacting with the hydrophobic core of lipids (Jacobs and White, Reference Jacobs and White1989).
Trp-containing CPPs and the lipid bilayer
The role of Trp in the interaction of cationic CPPs with model membrane has been explored in several studies. In 2012, Rydberg and coworkers demonstrated that the position of tryptophan in the sequence of a series of Arg/Trp peptides (Table 4) can affect both their internalisation efficiency into mammalian cells and their ability to inhibit bacterial growth (Rydberg et al., Reference Rydberg, Carlsson and Nordén2012a, Reference Rydberg, Matson, Åmand, Esbjörner and Nordén2012b). These authors further explored the impact of the position of 4 Trp combined with 8 Arg, on the interaction of the three dodecapeptides with supported lipid bilayers (Rydberg et al., Reference Rydberg, Kunze, Carlsson, Altgärde, Svedhem and Nordén2014). Their results indicate that the position of the Trp in the sequence alters the way the peptides interact with lipid membranes. Interestingly, in the presence of cholesterol, the dodecapeptide with a regular distribution of Trp (at positions 2, 5, 8 and 11) cause greater membrane disturbance (but not cytotoxicity), and internalisation efficiency compared to the two other peptides that contain a cluster of 4 Trp.
The role of Trp interacting with lipid bilayers was alos extensively studied with analogues of RW9 (Table 4). Quantification of the cellular uptake of the RW9 analogues in which Trp were stepwise replaced by Phe residues (Jobin et al., Reference Jobin, Blanchet, Henry, Chaignepain, Manigand, Castano, Lecomte, Burlina, Sagan and Alves2015), reveals that this substitution strongly reduces the internalisation of all peptides despite the fact that they strongly accumulate in the cell membrane. All peptides partition in the membrane similarly and locate just below the lipid headgroups (~1 nm) with slight different insertion depths for the different peptide analogues. Interestingly, it was shown that there is a direct relationship between the number of Trp residues in the peptide sequences and the reversibility of their interactions with membrane, as demonstrated by the resistance to washing steps. Altogether, a more interfacial location of a CPP renders its interaction with the membrane core more adjustable and transitory, and enhances its internalisation ability. Therefore, one may wonder how these hydrophobic interactions affect so differently peptide penetration efficacies.
In the case of RW9 and RL9 peptides (Walrant et al., Reference Walrant, Correia, Jiao, Lequin, Bent, Goasdoué, Lacombe, Chassaing, Sagan and Alves2011), large differences in their internalisation abilities are observed, RW9 being an efficient CPP when RL9 poorly enters into cells. The effect of these peptides on the thermotropic phase behaviour of a zwitterionic lipid (1,2-dimyristoyl-sn-glycero-3-phosphocholine, DMPC) and an anionic lipid (1,2-dimyristoyl-sn-glycero-3-phosphoglycerol, DMPG) has first shown that the presence of negative charges on the lipid headgroups are required to trigger peptide/lipid interactions. Interestingly, RW9 has more affinity for negatively charged membranes than RL9 (Walrant et al., Reference Walrant, Correia, Jiao, Lequin, Bent, Goasdoué, Lacombe, Chassaing, Sagan and Alves2011; Witte et al., Reference Witte, Olausson, Walrant, Alves and Vogel2013). RW9 also increases the bilayer fluidity and interferes with the fatty acid chain packing more than RL9 (Walrant et al., Reference Walrant, Correia, Jiao, Lequin, Bent, Goasdoué, Lacombe, Chassaing, Sagan and Alves2011). An increase in the lipid bilayer fluidity renders the bilayer more prone to lipid supramolecular reorganisation (formation of lipid domains, membrane bending and curvature) and protein rearrangements occurring during peptide translocation. Indeed, these two peptides do not have the same penetration depth, and they do not lead to the same lipid organisation while having a different insertion kinetic. The insertion of these peptides has been followed by surface pressure measurement that shows an increase in the surface pressure for RW9 in presence of a DMPC/DMPG mixture, which is not the case of RL9. These different behaviours are due to a more fluidising bilayer effect of RW9 rather than RL9 as revealed by attenuated total reflectance-Fourier transform infrared spectroscopy (ATR-FTIR) and differential scanning calorimetry (DSC) (Walrant et al., Reference Walrant, Correia, Jiao, Lequin, Bent, Goasdoué, Lacombe, Chassaing, Sagan and Alves2011, Reference Walrant, Vogel, Correia, Lequin, Olausson, Desbat, Sagan and Alves2012b). On the other hand, NMR experiments and molecular dynamic simulations revealed that RL9 inserts deeper into membranes (Walrant et al., Reference Walrant, Vogel, Correia, Lequin, Olausson, Desbat, Sagan and Alves2012b). Trp insertion inside the hydrophobic core disturbs lipid acyl chain cohesive hydrophobic interactions due to its bulky side chain which is entropically unfavourable (Yau et al., Reference Yau, Wimley, Gawrisch and White1998).
Studies of the longer analogues RL16 and RW16, and their original model Penetratin also provide interesting information on the role of Trp in the interaction with lipid membranes. It was first showed that Penetratin and RL16 do not share the same preference regarding their lipidic intretaction partners. Indeed, RL16 interacts preferentially with bilayers composed of zwitterionic PC whereas Penetratin prefers intercating with anionic PG, as determined by DSC (Alves et al., Reference Alves, Goasdoué, Correia, Aubry, Galanth, Sagan, Lavielle and Chassaing2008). Interestingly, RW16 perturbs both PC and PG bilayers (Jobin et al., Reference Jobin, Bonnafous, Temsamani, Dole, Grélard, Dufourc and Alves2013). Membrane perturbations can also be probed by calcein leakage. At low RW16 concentrations, less calcein leakage is obtained with 1,2-dioleoyl-sn-glycero-3-phosphoglycerol (DOPG) as compared to 1,2-dioleoyl-sn-glycero-3-phosphocholine (DOPC). One could expect that in the presence of zwitterionic lipids, peptides could be more inserted inside the hydrophobic core thanks to its hydrophobic part composed of Trp whereas electrostatic interactions with DOPG maintain the peptide at the membrane surface. RW16 insertion can be followed by Trp fluorescence, a blueshift being observed when the Trp is in a hydrophobic environment (Ladokhin and White, Reference Ladokhin and White2004; Thorén et al., Reference Thorén, Persson, Esbjörner, Goksör, Lincoln and Nordén2004). A smaller concentration of RW16 is required to observe a blueshift in the presence of DOPG large unilamellar vesicle (LUVs) than in the presence of DOPC LUVs. This implies either self-association of the peptide or a deeper insertion of this peptide inside the LUV. When taking all these information together, two different mechanisms could be proposed in which the Trp does not play the same role according to the nature of lipids (Jobin et al., Reference Jobin, Bonnafous, Temsamani, Dole, Grélard, Dufourc and Alves2013).
Very recently, the role of a particular minoritarian lipid, PI(4,5)P2, in the internalisation of Penetratin was studied (Bechtella et al., Reference Bechtella, Chalouhi, Rodríguez, Cosset, Ravault, Illien, Sagan, Carlier, Lequin, Fuchs, Sachon and Walrant2022). Bechtella et al., showed that this lipid was important for Penetratin uptake and that a less as 5% of this anionic lipid in a PC membrane could be enough to trigger the interaction of Penetratin with the membrane. NMR and molecular dynamic simualtions highlighted an interfacial positioning of Trp48, and showed a direct interaction of this residue with Arg52, which allows its unusually deep insertion in the membrane, and its involvement in a hydrogen-bond with sn1 carbonyl of PI(4,5)P 2.
Trp-containing AMPs and the lipid bilayer
The role of Trp in the membranotropic activity of AMPs is well-documented. It is indeed established that AMPs generally share a positive net charge, hydrophobicity and amphipathicity, that are essential properties for disruption and lytic activity of bacterial membranes (Hancock, Reference Hancock2001). In this regard, Trp is always described as an essential amino acid, thanks to its interfacial properties. Trp was often preferred over Tyr and Phe residues since various studies reported that Trp-containing AMPs are more active than those containing either Tyr or Phe (Subbalakshmi et al., Reference Subbalakshmi, Bikshapathy, Sitaram and Nagaraj2000; Strøm et al., Reference Strøm, Haug, Skar, Stensen, Stiberg and Svendsen2003; Dathe et al., Reference Dathe, Nikolenko, Klose and Bienert2004). The rational behind this preference of Trp over Tyr and Phe has been further studied. Trp is able to insert into membranes and to position at the membrane-water interface (Killian et al., Reference Killian, Salemink, De Planque, Lindblom, Koeppe and Greathouse1996; Wimley and White, Reference Wimley and White1996; Kachel et al., Reference Kachel, Asuncion-Punzalan and London2002). It is now well-established that Trp can be involved in more noncovalent interactions with the membrane components compared to Tyr and Phe (Khemaissa et al., Reference Khemaissa, Sagan and Walrant2021)).
Among natural Trp-containing AMPs, indolicidin (ILPWWKWPWWPWRR) is active against Gram-positive and Gram-negative bacteria (Selsted et al., Reference Selsted, Novotny, Morris, Tang, Smith and Cullor1992). In interaction with DPC micelles, the peptide adopts an extended structure (Rozek et al., Reference Rozek, Friedrich and Hancock2000) and is located at the water-membrane interface. Importantly, the latter study demonstrated that to kill bacteria, there is no need that the peptide structure conforms to a canonical secondary structure or be amphipathic. Tritrpticin (VRRFPWWWPFLRR) has also broad antimicrobial activity against Gram-positive and Gram-negative bacteria (Lawyer et al., Reference Lawyer, Pai, Watabe, Borgia, Mashimo, Eagleton and Watabe1996). In interaction with SDS micelles, the peptide adopts an amphipathic turn-turn conformation with the three Trp cluster inserting into the hydrophic core of the micelles (Schibli et al., Reference Schibli, Hwang and Vogel1999). Although of different peptide length and sequence, it was also shown that Tritrpticin, Indolicidin, Lactoferricin B (Lfcin B, FKCRRWQWRMKKLGAPSITCVRRAFA) and LfcinB4-9 (RRWQWR) share similarities in their interaction with lipid membranes. In particular, the more potent AMP are also those that are the more rigidly sequestered into the membrane (Schibli et al., Reference Schibli, Epand, Vogel and Epand2002).
The membranotropic activity of these peptides was explored using membrane models. Tritrpticin has channel-like activity (Salay et al., Reference Salay, Procopio, Oliveira, Nakaie and Schreier2004). The three Trp are crucial for the antimicrobial activity and their relative contribution in this activity has been examined (Arias et al., Reference Arias, Nguyen, Kuczynski, Lejon and Vogel2014). Permeabilisation assays of synthetic membranes established that Trp1 in the triad is the most important for membrane disruption and antimicrobial activity of the peptide, in contrast to Trp3, while Trp2 has an intermediate role. It is assumed that when tritrpticin inserts into the membrane, the Trp triad forms indeed a hydrophobic cluster, which causes lateral pressure in the bilayer and induces positive curvature strain (Schibli et al., Reference Schibli, Epand, Vogel and Epand2002). Finally, during irreversible pore formation, the interactions of the flanking Arg residues with the anionic lipid headgroups are predicted to pull the phospholipids along into the pore.
Indolicidin inserts at low concentration at the outer leaflet of the bilayer in the interface between the headgroup and tail core (Halevy et al., Reference Halevy, Rozek, Kolusheva, Hancock and Jelinek2003; Nielsen et al., Reference Nielsen, Lind, Lone, Gerelli, Hansen, Jenssen, Cárdenas and Lund2019). This insertion significantly decreases the lipid packing order of the bilayer. At higher concentrations, indolicidin penetrates deeper into the bilayer and evokes lipid removal that leads to a slight membrane thinning. These results suggest that indolicidin acts through interfacial activity rather than through the formation of stable pores. This membrane-thinning action of indolicidin was observed by molecular dynamics simulations (Hsu and Yip, Reference Hsu and Yip2007; Neale et al., Reference Neale, Hsu, Yip and Pomès2014). Finally, for Lactoferricin B, the membrane potential is important in the lytic activity of the peptide, when the mechanism involved is not clear yet (Hossain et al., Reference Hossain, Moghal, Islam, Moniruzzaman and Yamazaki2019).
Beside these natural AMP that contain Trp residues, different studies with designed AMPs containing only Arg /Lys and Trp residues have been reported. In 2003, Strøm and coworkers intended to determine the minimum requirement of net charge and bulky/lipophilic properties for AMP against Gram-negative and Gram-positive bacteria (Strøm et al., Reference Strøm, Haug, Skar, Stensen, Stiberg and Svendsen2003). For that purpose, they combined Arg and Trp residues in tripeptides (2R, 1W), tetrapeptides (2R, 2W), pentapeptides (3R, 2W) and hexapeptides (3R, 3W), as described previously. The recorded antimicrobial activities support the four aminoacids (2R, 2W) as the minimal pharmacophore. Using the same dipeptide repeat (RW)n-NH2 (n = 1 to 5), Liu determined that the antimicrobial and haemolytic activities of these peptides increase with the sequence length (Liu et al., Reference Liu, Brady, Young, Rasimick, Chen, Zhou and Kallenbach2007), but that the (RW)3 is the optimal peptide. Secondary structure analysis (circular dichroism, CD) indicated that these (RW)n peptides are unfolded in solution but acquire structure in the presence of phospholipids, although it was not analysed further because the indole chain interferes in the 225 nm region of the CD spectra. Hou further confirmed (RW)3 as an optimal antimicrobial sequence and showed that the hexa- and octapeptides (RW)3 and (RW)4 are in addition active against bacteria biofilms (Hou et al., Reference Hou, Liu, Young, Mark, Kallenbach and Ren2010).
Gopal designed a series of di- to pentapeptides with (KW)n repeats to evaluate their antimicrobial activity (Gopal et al., Reference Gopal, Seo, Song and Park2013). The results support the (KW)4 octapeptide as the most efficient antimicrobial sequence with low associated haemolytic activity. The authors proposed that the mechanism behind implies that the (KW)4 peptide binds first to phospholipid polar heads by electrostatic interactions, then the Trp promotes hydrophobic interactions with acyl chains and destabilisation of the lipid bilayer.
Conclusion and perspectives
Trp is an amino acid with unique physico-chemical properties allowing it to be involved in several interactions such as cation-π, anion-π, ion pair-π interactions, hydrogen bonds and hydrophobic contacts with various membrane partners. All these interactions make Trp a key element for the comprehensive understanding of internalisation and the antibacterial action of membrane-active peptides. How AMPs and CPPs exert their membranotropic activity is not yet fully understood. The reason behind is clearly resulting from the many interactions Trp can establish through various noncovalent bondings with different partners at the cell-surface. In this review, we focused mainly on its interactions with lipids, oligo- and polysaccharides and their conjugates but, since the membrane is a very complex environment, other partners should be probably considered. For instance, water molecules also constitute potential partners for Trp interactions. It has been demonstrated by classical, quantum mechanics/molecular mechanics molecular dynamics simulation (QM/MM) molecular dynamics simulations and NMR studies that the Trp residue in engrailed homeodomain interacts with water through a dynamic switching between OH-π hydrogen bonding and lone pair-π interactions. These interactions are in general involved in protein stabilisation and function (Kozelka, Reference Kozelka2017; Špačková et al., Reference Špačková, Trošanová, Šebesta, Jansen, Burda, Srb, Zachrdla, Žídek and Kozelka2018).
Furthermore, insertion of CPP for membrane translocation is also sensitive to the membrane potential difference between the inner and the outside of the membrane notably caused by the asymmetric distribution of ions. Increasing the transmembrane potential (hyperpolarisation) leads to an easier penetration because the energetical barrier required to cross the membrane is lower. Calculations show a smaller barrier for (RW)9 peptide than for R 9 probably due to its amphipathic character (Via et al., Reference Via, Klug, Wilke, Mayorga and Del Pópolo2018).
Thus, Trp appears to be an important amino acid for CPPs efficiency, consequently, it is a residue to take into account for the design of non-viral gene delivery systems for example (McErlean et al., Reference McErlean, Ziminska, McCrudden, McBride, Loughran, Cole, Mulholland, Kett, Buckley, Robson, Dunne and McCarthy2021).
The biological properties of these peptides can also be enhanced by cyclisation that can occurs either naturally thanks to Trp playing the role of linker or by chemical synthesis. These interesting peptides display large biological activities. Many total syntheses of cyclic peptides have been carried out over the past 80 years (Swain et al., Reference Swain, Walker, Calvert and Brimble2022).
Chemical modifications can also be introduced on tryptophan given its singular reactivity. These modifications allow a better understanding of the function post-translational modifications (PTMs) on natural proteins. They also enable the design of improved probes for bioimaging or the optimisation of protein/peptides-based drugs (Hu et al., Reference Hu, He and Li2021).
Recently, cyclic linear hydrid peptides containing alternately Arg and Trp residues have been developed, as described in the text. Their transport efficiency of molecules for therapeutic purposes such as anti-HIV drugs with short incubation time has been demonstrated (Khayyatnejad Shoushtari et al., Reference Khayyatnejad Shoushtari, Zoghebi, Sajid, Tiwari and Parang2021).
Tryptophan-containing peptides can also be used for detection and diagnostic purposes as illustrated by the cyclic peptide ligand, cy(WQETR) that binds to the terbium ion through its Trp residue enhancing thus the terbium luminescence thanks to the antenna effect. This system is able to detect tromethamine in biological media (Ji et al., Reference Ji, Shao, Li, Wang, Chaudhuri, Guo, Perkins, Sarkar and Xue2021).
The main disadvantage of CPPs is their lack of selectivity. To overcome this problem, a peptide containing 2 Trp residues and able to change its conformation from random coil to hairpin has recently been designed. This latter structure facilitates its internalisation in tumour cells, providing selectivity (Nishimura et al., Reference Nishimura, Nishida and Tanaka2022).
Another aspect that was not discussed in this review is the incorporation of Trp-like structures in peptidomimetics or polymers. For example, very small peptide mimics composed of β-homo-bis-Arg and Tbt showed interesting antimicrobial properties (Boullet et al., Reference Boullet, Bentot, Hequet, Ganem-Elbaz, Bechara, Pacreau, Launay, Sagan, Jolivalt, Lacombe, Moumné and Karoyan2019). Ribbon-like α-amino γ-lactam foldamers inspired by Arg/Trp peptides were efficiently internalised by cells (Vezenkov et al., Reference Vezenkov, Martin, Bettache, Simon, Messerschmitt, Legrand, Bantignies, Subra, Maynadier, Bellet, Garcia, Martinez and Amblard2017). Similarly, the incorporation of indole moieties in guanidinium-containing polymethacrylamide polymers significantly enhanced their transfection efficiency (Cokca et al., Reference Cokca, Zartner, Tabujew, Fischer and Peneva2020).
Altogether, we show herein that Trp must be considered in the design of new systems to address current issues in vectorisation, diagnostic and therapy. Many studies are currently in progress in order to better understand its various roles in the internalisation and antibacterial mechanisms.