It is credible that affluent access to food contributes to excess energy intake and metabolic disease(Reference Hill, Wyatt and Reed1). Thus, this obesogenic environment makes the strategy to treat and prevent obesity obvious namely, eat less and move more. However, the average over-consumption of energy which accumulates and result in overweight and obesity in a population over time is 41·84–104·6 kJ (10–25 kcal)/d(Reference Arner, Andersson and Bäckdahl2) and is virtually impossible to detect on a daily basis with current technology. Hence, it is challenging to be conscious of energy balance.
The type of food is arguably easier to modify as it is categorical, i.e. fish v. red meat or oil v. butter. The fatty acid (FA) composition of the food choices is important for the way the body responds to the energetic intake as FA are potent signalling molecules regulating gene transcription(Reference Afman and Müller3). Thus, instead of decreasing energy intake, interventions targeting dietary lipid composition may allow for a more metabolically benign weight gain via regulation of the transcriptional response to over-nutrition.
A benign weight gain is among other things characterised by less adverse metabolic alterations as compared to pernicious weight gain. This includes an efficient subcutaneous white adipose tissue (WAT) expansion in order to minimise the risk of ectopic lipid deposition, lipotoxicity followed by perturbed metabolic health(Reference Crewe, An and Scherer4). This review focuses on dietary FA and their effect on human WAT function.
White adipose tissue in health and disease
The WAT is the most plastic organ of the human body and can expand immensely in response to energy intake(Reference Rosen and Spiegelman5). Excess energy is stored as TAG within a monocular lipid droplet in the fat cell, the specific specialised cell in WAT. These cells can more than double in size in obese individuals when compared with lean. During hypoenergetic conditions or during physical performance, the TAG are hydrolysed in a process termed lipolysis which results in a release of free FA and glycerol into the circulation. However, lipolysis is a continuous process which occurs at a low unstimulated (basal) rate and is acutely influenced by hormones. In human subjects, lipolysis is regulated by three hormone systems, catecholamines and natriuretic peptides, which are stimulatory, and insulin which is inhibitory(Reference Lafontan and Langin6). Inefficient stimulated lipolysis is associated with the development of obesity while an increased basal lipolysis is associated with metabolic disease(Reference Arner and Langin7). Another important function of WAT, which was not known until the 1990s, is that it is an endocrine organ which secretes several factors termed adipokines including pro-inflammatory cytokines and chemokines(Reference Rosen and Spiegelman5). Metabolic health is closely linked to chronic low-grade inflammation in WAT. All these processes are synchronised in a healthy WAT and they are involved in maintaining homeostasis. Perturbation of one of these processes will disrupt the synchrony and also affect the other which then results in metabolic disease. In fact, defects in these processes have been individually linked to increased cardiovascular risk(Reference Rydén and Arner8–Reference Berg and Scherer10).
Furthermore, the WAT organ is heterogeneous with several depots but can be subdivided into two major ones, namely the subcutaneous and the visceral. The former is by far the largest depot and the latter associates strongly with a detrimental metabolic phenotype(Reference Gesta, Tseng and Kahn11). The subcutaneous depots are mainly located in the femoral and abdominal region while the visceral depots exist within the abdominal cavity. The regional fat deposition differs markedly between men and women. Women have a larger subcutaneous, and in particular femoral, depot which is characterised by a pear-shaped figure while men have an apple-shaped figure due to a larger visceral depot(Reference Gesta, Tseng and Kahn11).
As previously mentioned, to complicate things further, these depots can be subdivided into several sub-depots which all are somewhat unique in their biology. However, herein we mainly focus on the abdominal subcutaneous and the visceral omental depot since they are frequently studied probably due to their accessibility. Visceral WAT mass has been hypothesised to cause metabolic disease by directly influencing the liver and pancreas with free FA and adipokines via the portal vein. Nevertheless, the location of the depot might not be the only factor since they also differ in their biology. These depots expand differentially in response to hyperenergetic conditions(Reference Petrus, Mejhert and Corrales12–Reference Jeffery, Church and Holtrup14). The subcutaneous adipose tissue expands mainly via increased fat cell size in comparison with the visceral which is characterised by a hyperplastic expansion (increased fat cell number). Furthermore, the visceral depot has a more active lipolysis together with a more pro-inflammatory secretome. Identifying interventions that control the regional body fat distribution would help in preventing metabolic disease. Hence, the largest component of this tissue, the lipids, has attracted a lot of focus from scientists with the aim to treat and prevent metabolic disease. The FA composition in WAT is influenced by the composition in the diet(Reference Hodson, Skeaff and Fielding15); thus, nutritional interventions with alterations in FA species has been performed in human subjects and are reviewed herein (Table 1).
Table 1. Interventions, reviewed herein, comparing dietary fatty acids (FA) and their effects in white adipose tissue (WAT)
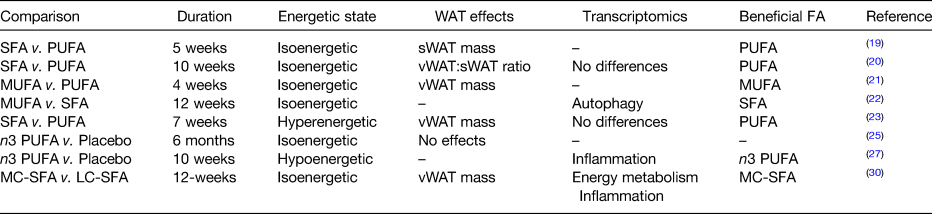
MC, medium chain; LC, long chain; sWAT, subcutaneous WAT; vWAT, visceral WAT.
Dietary fatty acids and white adipose tissue function
FA are monocarboxylic aliphatic molecules which exist in a variety of carbon lengths and saturation degrees. In human subjects, the fat is mainly composed of TAG built up by FA with chain lengths of 12–22 carbon atoms and saturation degrees of 0–6 double bonds(Reference Seidelin16). FA are often named numerically with the number of carbon atom first and the number of double bonds after with a colon punctuation mark dividing the numbers. Furthermore, the position of the double bond influences the properties of the FA and therefore information on the positioning is given when relevant(17). Most commonly mentioned is n-3 and n-6 FA.
The prevalence of CVD has increased in parallel with the rapidly evolved society which has changed the dietary habits of the population. Thus, nutritional interventions such as altering the dietary FA composition have been performed in order to prevent and treat CVD. These studies collectively suggest that replacing SFA with PUFA lowers the risk of cardiovascular events(Reference Mozaffarian, Micha and Wallace18). Novel interventions to treat cardio-metabolic disease could be developed by elucidating the mechanisms of action as regards FA composition on various organs, including the WAT.
The FA composition of the diet impacts on the regional fat distribution with PUFA having a more favourable effect. In isoenergetic conditions, a PUFA-rich diet instead of SFA lowers the total subcutaneous WAT area along with improved insulin sensitivity and blood lipids(Reference Summers, Fielding and Bradshaw19). The protective effects of PUFA on measures for cardio-metabolic health were confirmed by others(Reference Bjermo and Iggman20), however, with no effects on WAT gene expression. Furthermore, PUFA intake was compared with MUFA during isoenergetic conditions where the latter had more beneficial effects with reduced visceral adiposity(Reference Liu, Kris-Etherton and West21). The transcriptional response to MUFA in subcutaneous WAT has been suggested to be mediated via the regulation of apoptosis-related genes(Reference Camargo, Rangel-Zúñiga and Alcalá-Díaz22). In an overfeeding study comparing PUFA with SFA, the former resulted in a larger lean tissue mass expansion while the latter resulted in the same amount of weight gain but more via visceral WAT expansion(Reference Rosqvist, Iggman and Kullberg23). These findings are supported by the self-reported intake of PUFA and SFA(Reference Cardel, Lemas and Jackson24). Nevertheless, the effects of dietary FA composition on measures for metabolic health are not likely to pertain to WAT function since neither Rosqvist et al. (Reference Rosqvist, Iggman and Kullberg23) nor others(Reference Hames, Morgan-Bathke and Harteneck25) found any major effects on subcutaneous WAT function. Furthermore, PUFA v. SFA overfeeding displayed minor epigenetic differences which also suggest that the beneficial effects of these dietary interventions are not meditated via the WAT(Reference Perfilyev, Dahlman and Gillberg26). In contrast, others identify the effects on extracellular matrix remodelling pathways by n-3 FA supplementation(Reference Huerta, Prieto-Hontoria and Sainz27). These controversies might be explained by the type of PUFA studied, dosage, study population and habitual diet.
Most studies investigating the effects of SFA have focused on the most prevalent one namely palmitic acid which is a long-chain FA. In fact, medium-chain FA have been shown to have beneficial effects on body composition, body fat distribution(Reference Mumme and Stonehouse28,Reference Bueno, de Melo and Florêncio29) and WAT function by regulating the transcriptome to favour oxidative phosphorylation and anti-inflammatory mechanisms(Reference Matualatupauw, Bohl and Gregersen30). Hence, classifying FA into SFA, MUFA and PUFA could be misleading since the individual role of each FA species could vary significantly and independently of the degree of saturation.
As afore-mentioned, dietary FA might not have an acute effect on WAT function. Conversely, the WAT might function as a reservoir for dietary FA and regulate physiology in the long term. In fact, subcutaneous linoleic acid levels were found to be inversely associated with all-cause mortality in elderly men albeit not to death caused by cardiovascular events alone(Reference Iggman, Ärnlöv and Cederholm31). The absent protective effect of α-linoleic acid on myocardial infarction has also been suggested by others(Reference Bork, Jakobsen and Lundbye-Christensen32). Nonetheless, WAT FA composition could influence the tissue function locally as well as systemically in the long term. Additionally, the effects could be mediated via the effects solely on the visceral WAT and not on subcutaneous fat which is not well studied due to the inconvenience to biopsy visceral fat as well as ethical issues. In fact, the FA composition in omental visceral WAT but not subcutaneous fat was associated with a negative association between waist:hip ratio (a proxy for regional WAT distribution) and PUFA content(Reference Garaulet, Pérez-Llamas and Pérez-Ayala33). Instead subcutaneous WAT MUFA content was associated with beneficial metabolic phenotypes (i.e. negative association with BMI, body fat percentage and visceral adiposity). Conversely, the FA composition is associated with distinct transcriptional profiles within the two depots confirming the regional heterogenic association between WAT function and FA composition(Reference Petrus, Edholm and Rosqvist34).
Taken together, dietary FA impact on various regional depots specifically with PUFA possibly having more beneficial effects in the visceral depot while MUFA having protective effects by affecting the subcutaneous depot. Nevertheless, intervention studies summarised herein suggest that the most pronounced response on subcutaneous WAT is obtained by replacing long-chain SFA with medium-chain SFA. These effects can at least in part be caused by depot-specific changes in gene expression between the fat depots. Moreover, it is important to highlight that reducing SFA intake should be done cautiously since the medium-chain sub-class has shown to have metabolically beneficial effects. In order to improve the awareness of the consumers, SFA content in food products should be reported as percentage medium-chain FA just like carbohydrates are sub-divided into sugars and non-sugars.
Mechanistic links between dietary fatty acids: white adipose tissue and disease
FA modulate cell biology at several levels(Reference Papackova and Cahova35). The saturation degree of FA regulates the fluidity of the cell membrane which then changes its properties such as protein composition and ability to transduce signals. FA could also regulate the activity of several transcription factors as well as transduce signals via cell membrane receptors. FA may re-wire metabolism and alter the levels of several intermediary metabolites that could crosstalk with the epigenome(Reference Berger and Sassone-Corsi36,Reference Papsdorf and Brunet37) . In fact, the levels of free FA function as co-enzymes for the sirtuin 6 which functions as a de-acetylase and is present in the nucleus(Reference Etchegaray and Mostoslavsky38). Conversely, acetyl-CoA is a product of lipid oxidation and functions as a substrate for acetylation processes(Reference Papsdorf and Brunet37). These mechanisms and how they are regulated by dietary FA in human adipose tissue remains to be uncovered. Many of the transcription factors that are activated by total or specific FA have been studied in the liver(Reference Papackova and Cahova35) and it might not be de facto in WAT. FA and prostaglandins have been described to modulate adipogenesis in WAT by binding and activating PPARγ(Reference Tontonoz, Hu and Spiegelman39,Reference Forman, Tontonoz and Chen40) ; however, these pathways were not found to be regulated in human interventions with various FA and energetic conditions(Reference Bjermo and Iggman20,Reference Rosqvist, Iggman and Kullberg23,Reference Dahlman, Linder and Arvidsson Nordström41) . Moreover, these studies did not observe any inflammatory effects of SFA either which has long been considered to be Toll-like receptor agonists. In fact, it was recently shown that the SFA palmitate is not a Toll-like receptor 4 but the receptor is needed for palmitate-induced inflammation(Reference Lancaster, Langley and Berglund42). Taken together, these discordant findings could be explained by several factors such as levels of dietary FA consumed, duration of intervention, WAT depot specific, species-specific effects or a combination. The species specificity is supported by studies in rats and murine 3T3-L1 suggesting that medium-chain SFA regulate body fat mass via inhibition of adipogenesis(Reference Han, Hamilton and Kirkland43); an observation not made in man after a dietary medium-chain SFA feeding intervention(Reference Matualatupauw, Bohl and Gregersen30).
The beneficial effects of the medium-chain SFA could be attributed to their unique way of being transported and metabolised. In fact, these FA are not incorporated into chylomicrons, rather they are transported as free FA(Reference Bach and Babayan44). Furthermore, they can translocate via the mitochondrial membrane carnitine independently, which allows a more efficient β oxidation. It is tempting to speculate that the enhanced β oxidation would regulate the anti-inflammatory effects of these SFA(Reference Matualatupauw, Bohl and Gregersen30) in the WAT via modulating acetyl-CoA levels and hence histone acetylation. Such hypotheses could be confirmed using current technology in randomised controlled trials followed by epigenetic analysis in WAT.
This leaves us with a rather unclear view of how dietary FA modulate body fat composition, WAT function and metabolic health. It is interesting that the visceral adiposity characterised by an apple-shaped phenotype that is enhanced by dietary SFA(Reference Bjermo and Iggman20,Reference Rosqvist, Iggman and Kullberg23) is also observed in Cushing's syndrome patients which could be mediated via elevated expression of 11-β-hydroxysteroid dehydrogenase type 1(Reference Bujalska, Kumar and Stewart45). The deletion of this enzyme results in a reduced visceral adipose tissue(Reference Wamil, Battle and Turban46). It is therefore interesting that overfeeding SFA instead of PUFA induced the expression of 11-β-hydroxysteroid dehydrogenase type 1 in rat visceral WAT(Reference Vara Prasad, Jeya Kumar and Kumar47) and the levels of SFA in visceral human WAT correlated with the gene and protein expression of the enzyme(Reference Petrus, Rosqvist and Edholm48).
Taken together, the previous mechanistic type of studies suggests that different types of FA have specific regulatory functions in adipose tissue. This includes the effects at the cell membrane level, gene transcriptional activity and co-enzyme activities. However, the detailed molecular mechanisms remain to be established.
Conclusions
Regional adipose tissue expansion and function are tightly linked to the development of CVD, which, in part, is influenced by the lipid composition of the diet. PUFA, MUFA and medium-chain SFA may induce a reduced mass and/or improved function of visceral WAT in comparison to long-chain SFA. The latter FA may cause expansion and/or worsened function of subcutaneous WAT. The mechanism of action remains to be discovered since many of the previously proposed pathways of FA as signalling molecules do seem to be activated in human WAT in vivo. Dietary FA may regulate body fat distribution via interplay between SFA and cortisol metabolism in visceral WAT. Further studies are needed in order to confirm this conceivable link and to understand FA action on WAT at the molecular level.
Acknowledgement
We would like to thank the members of the lipid laboratory at the Karolinska Institute as well as the reviewer for their input.
Financial Support
We thank the Karolinska Institute for financial support.
Conflict of Interest
None.