INTRODUCTION
Effective vaccines are available for many protozoal diseases of animals (see Tables 1–4). In contrast, no vaccine is widely available for any protozoal disease of humans, despite great need and considerable effort. Some of the more important protozoal diseases of humans for which vaccines could be invaluable include falciparum malaria, vivax malaria, Chagas' disease, African trypanosomiasis, visceral leishmaniasis, cutaneous leishmaniasis, cryptosporidiosis, and giardiasis.
Table 1. Protozoal immunizations with live virulent organisms using controlled-exposure or infection-and-treatment protocols

Table 2. Protozoal vaccines that contain live attenuated organisms

Table 3. Protozoal vaccines that contain whole killed organisms

Table 4. Protozoal vaccines that contain defined antigens or antigen extracts

In comparison to animal vaccine research, human vaccine research has an extra high hurdle in needing to test vaccine efficacy within humans themselves. Nevertheless, this problem is not insurmountable, and numerous highly credible experiments and field trials have been performed on humans (Armijos et al. Reference Armijos, Weigel, Romero, Garcia and Salazar2003; Roestenberg et al. Reference Roestenberg, McCall, Hopman, Wiersma, Luty, van Gemert, van de Vegte-Bolmer, van Schaijk, Teelen, Arens, Spaarman, de Mast, Roeffen, Snounou, Renia, van der Ven, Hermsen and Sauerwein2009; Olotu et al. Reference Olotu, Fegan, Wambua, Nyangweso, Awuondo, Leach, Lievens, Leboulleux, Njuguna, Peshu, Marsh and Bejon2013; Seder et al. Reference Seder, Chang, Enama, Zephir, Sarwar, Gordon, Holman, James, Billingsley, Gunasekera, Richman, Chakravarty, Manoj, Velmurugan, Li, Ruben, Li, Eappen, Stafford, Plummer, Hendel, Novik, Costner, Mendoza, Saunders, Nason, Richardson, Murphy, Davidson, Richie, Sedegah, Sutamihardja, Fahle, Lyke, Laurens, Roederer, Tewari, Epstein, Sim, Ledgerwood, Graham and Hoffman2013). So why is it that protozoal vaccines are only available for animals? A number of factors are suggested later in this review.
Indeed, several effective veterinary vaccines do have designs that should be suitable for human vaccines. Vaccine designs that have had repetitive veterinary successes should be considered for adaptation for human vaccines. Protozoal vaccine scientists should familiarize themselves with the comparative performance records of the various design categories of experimental and commercial vaccines, and in particular they should analyse the properties and production methods of the best vaccines that have been used to combat animal diseases.
The following sections of this analytical review article describe the main classes of veterinary protozoal vaccine designs. Illustrative details are provided of the composition and performance of several vaccines, most of which are in current use; readers who prefer less detail may skip to the short descriptions listed in Tables 1–4. Experimental protozoal vaccines are then presented in two sections, the first describing experimental vaccines with good evidence of efficacy, and the second analysing several prominent types of experimental malaria vaccines; these are summarized in Tables 5 and 6. Factors are then suggested to account for the general differences in performance of each of these vaccine classes, and suggestions are offered to assist in the development of effective protozoal vaccines for both animals and humans.
Table 5. Experimental animal and human immunizations using whole protozoal organisms a
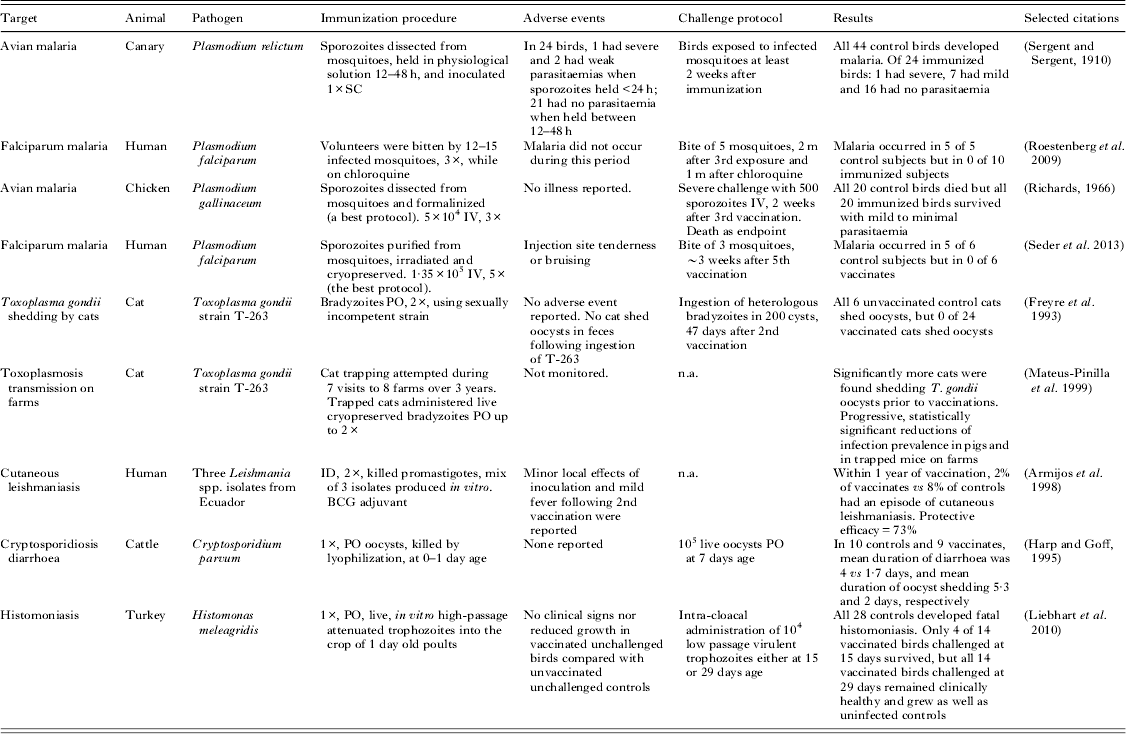
a All studies in this table used natural hosts and incorporated challenge controls or epidemiological comparisons.
Table 6. Representative comparisons of molecular malaria vaccine designs and their performance in artificially adapted animal models and human trials a

a To date, no protozoal vaccine based on these or similar molecular designs has achieved widespread success in humans or animals.
Throughout this manuscript, a vaccine is considered to have effective immunity or to demonstrate efficacy if it prevents infection or reduces disease incidence or severity, provided that such benefits extend for a useful period of time under field conditions. No assumptions are made about vaccine efficacy based on humoral, cellular, or innate immune response data, or experiments in vitro or within non-target animal models.
PROS AND CONS OF VETERINARY VERSUS HUMAN VACCINE RESEARCH
Comparative advantages for veterinary vaccine development
Vaccine development for animal diseases has the following advantages over vaccine development for human diseases.
Infectious challenge experiments
Development of vaccines for animals usually includes infectious challenge experiments using the natural animal host (Cornelissen and Schetters, Reference Cornelissen and Schetters1996; Shkap and Pipano, Reference Shkap and Pipano2000), enabling experiments with high predictive value to advance rapidly. Performing similar trials in humans has greater ethical and regulatory burdens and can be impossible or require compromise of experimental design. Therefore, initial efficacy data for humans may have to be acquired in the field under circumstances which can be difficult to control.
In vivo production of organisms
A second advantage in the development of animal protozoal vaccines is the option to manufacture vaccine organisms in vivo. Poultry coccidiosis vaccines are produced by infection of birds and collection and purification of organisms from their feces or intestines (Williams, Reference Williams2002; Sharman et al. Reference Sharman, Smith, Wallach and Katrib2010). Many babesiosis vaccines are produced by infection of splenectomized animals and harvesting the infected erythrocytes (Callow et al. Reference Callow, Dalgliesh and de Vos1997). Although this is a clear manufacturing advantage, any vaccine that contains animal products is subject to marketing and regulatory disadvantages regarding international registration and importation.
Less rigorous regulation
Licensing requirements for human vaccines are stringent in modern industrial nations, including a requirement to demonstrate efficacy (see Gruber, Reference Gruber2011 for a review of US regulations). In comparison, animal vaccines have often appeared to have lower regulatory hurdles, as evidenced by commercial sales of various products prior to clear demonstration of efficacy (Choromanski and Block, Reference Choromanski and Block2000; Witonsky et al. Reference Witonsky, Morrow, Leger, Dascanio, Buechner-Maxwell, Palmer, Kline and Cook2004). Regulation of veterinary products in the European Union and the USA is becoming more rigorous (as reviewed by Schetters and Gravendyck, Reference Schetters and Gravendyck2006).
Comparative advantages for human vaccine development
Research funding
Naturally, public demand is much greater for treatments and vaccines to combat human diseases than for animal diseases. As a result, vastly more funding is available for human disease research of all types in comparison with animal disease research. For example, the 2012 budget for the US National Institutes of Health (NIH, 2012), which exists to perform and support human medical research, was $25·38 billion. In comparison, the US Department of Agriculture funds intramural animal health research via the Agricultural Research Service (ARS), and extramural animal health research via the National Institute for Food and Agriculture (NIFA). The ARS budget in 2012 that was allocated for ‘animal protection’, denoting animal disease research, was $0·08 billion (USDA, 2012). The last thorough analysis of NIFA's extramural research support was for a period ending in 2007 (NIFA, 2009), a year when approximately $0·05 billion was awarded for animal disease research. Although it isn't possible to compare with precision, NIH (human) and USDA (animal) health and disease research budgets clearly differ by orders of magnitude, and similar funding trends are apparent in the size and number of private charities and commercial industries that are devoted to human or animal health. This general funding environment affects all types of disease research, including efforts to develop protozoal vaccines.
Product pricing
Manufacturers of human vaccines benefit from significantly higher prices and increased demand for their products as a result of human priorities, medical insurance, government and school policies, and international humanitarian efforts. Pricing of livestock vaccines can be meagre in comparison; poultry coccidiosis vaccines are examples of products from which manufacturers need high volumes to compensate for exceptionally low prices (Williams, Reference Williams2002).
TYPES OF PROTOZOAL VACCINES IN USE
Most existing protozoal vaccines are for pathogens in the phylum Apicomplexa. Members of this phylum are obligate intracellular parasites that actively invade host cells and have both asexual and sexual reproductive cycles.
A small number of vaccines have been produced for non-Apicomplexan protozoa. These organisms only have asexual reproduction. Amastigotes of Leishmania spp. multiply within phagocytes of the vertebrate host although they do not invade non-phagocytic cells, and the promastigote stages replicate extracellularly within arthropod vectors. Giardia lamblia and Tritrichomonas foetus are strictly extracellular parasites.
Tables 1–4 provide examples of the types of protozoal vaccines that are in current use or have been in recent distribution.
Human immunizations
Leishmanization
Old World and New World forms of cutaneous leishmaniasis are similar conditions caused by different species of Leishmania, which are transmitted by the bite of various phlebotomine sandflies. Infected bites may develop into chronic, ulcerated wounds (Ameen, Reference Ameen2010). Although most lesions ultimately resolve, disfiguring scars may result which can be particularly distressing when they occur on the face. Leishmanization is a controlled-exposure type of immunizing procedure that has been used to protect against cutaneous leishmaniasis (Table 1). Similar procedures were developed and used by several governments in the Middle East and central Asia, and related natural exposure practices (to sandflies) had been in prior use. In Iran, live virulent trophozoites of Leishmania major were produced by intraperitoneal mouse passage followed by limited axenic passage in broth (Nadim et al. Reference Nadim, Javadian, Tahvildar-Bidruni and Ghorbani1983). The fresh live inoculum was administered intradermally or by scarification at a site in an arm or shoulder. If the procedure was a ‘take’, then a cutaneous lesion would develop at the inoculation site. This wound could endure months and in some cases longer than a year before healing with a scar. Immunized individuals were protected from disfiguring scars on the face that may result from natural exposure to L. major-infected sandfly vectors.
Quality assurance of these biological products was difficult. Adverse events were reported, most notably including persistent sores. Despite these problems, leishmanization was credited with greater than 80% reduction of disease prevalence (Nadim et al. Reference Nadim, Javadian and Mohebali1997). A review by Dunning (Reference Dunning2009) reported that leishmanization was recently employed in Uzbekistan.
Malaria fever therapy
To the author's knowledge, no other human protozoal immunizations have achieved widespread use, other than the possible historical inclusion of virulent malaria inoculations for fever therapy of neurosyphilis (‘general paralysis of the insane’) practiced in the early and mid-20th century. The intention was not to immunize against malaria, but rather to induce a high fever that would kill or control Treponema pallidum and thereby halt or reduce neurological impairment (as reviewed by Kragh, Reference Kragh2010) . Case reports have been compiled and examined for the effect upon the course of malaria itself when induced in patients more than one time, but highly varied treatment regimens and the understandable lack of controls make conclusions difficult (Collins and Jeffery, Reference Collins and Jeffery1999).
Immunization using controlled-exposure and infection-and-treatment protocols (see Table 1)
A logical improvement upon immunization with virulent organisms without treatment, where the infection is allowed to run its full course (controlled-exposure), is to infect patients with virulent organisms and then administer a prophylactic antimicrobial (infection-and-treatment). This practice is still widely used for East coast fever of cattle, and until recently was a common technique for control of poultry coccidiosis. An infection-and-treatment protocol was also recently used for experimental immunization against human malaria (Roestenberg et al., Reference Roestenberg, McCall, Hopman, Wiersma, Luty, van Gemert, van de Vegte-Bolmer, van Schaijk, Teelen, Arens, Spaarman, de Mast, Roeffen, Snounou, Renia, van der Ven, Hermsen and Sauerwein2009; Table 5).
East coast fever of cattle
This is an economically important disease in eastern, central and southern regions of Africa. The causative organism is Theileria parva. Regionally varied species, subspecies, or variants of T. parva exist for which cross-protection may be poor (reviewed by Uilenberg, Reference Uilenberg1999). The parasite is transmitted by Rhipicephalus ticks and causes high mortality in Bos taurus cattle, less mortality in Bos indicus cattle, while native species of buffalo appear to be well-adapted natural reservoirs. Organisms transmitted by ticks invade, replicate within, and transform bovine lymphocytes, prior to invading erythrocytes from whence they are ingested by ticks; sexual recombination occurs in the tick prior to forming sporozoites within salivary glands.
These organisms are difficult to cultivate in vitro and reliable attenuation has not been achieved. Virulent seed stock organisms are used to infect cattle in the vaccine production process, which are then used to infect ticks. Organisms are harvested from tick salivary glands and are semiquantified, may be cryopreserved, and are used to immunize cattle in the field. Cattle are protected from disease by simultaneous injection of a long-acting formulation of oxytetracycline (Radley et al. Reference Radley, Brown, Cunningham, Kimber, Musisi, Payne, Purnell, Stagg and Young1975). Efficacy of this infection-and-treatment protocol is high and protection endures at least 3 years, but sterile immunity is not achieved. Immunized animals develop a carrier state with potential to transmit virulent organisms to ticks.
A multivalent vaccine (the Mugaga cocktail) (Patel et al. Reference Patel, Lubembe, Gachanja, Mwaura, Spooner and Toye2011), originally developed by the East African Veterinary Research Organization (now the Kenya Agriculture Research Institute), is licensed and being produced by a commercial enterprise for distribution in Kenya, Tanzania and Malawi (GALVmed, 2010). Different vaccine seed stocks are used in other regions. It is possible to immunize cattle in Zimbabwe using low-titration doses of the Boleni stock of T. parva without concurrent administration of antibiotics, attributed to its lower virulence (Latif and Hove, Reference Latif and Hove2011).
The life cycle of the parasites of bovine theilerioses shares certain generalized features with that of malaria parasites, including sexual reproduction within a haematophagous arthropod vector, initial invasion within nucleated cells of the vertebrate host, and subsequent invasion of erythrocytes (Marquardt et al. Reference Marquardt, Demaree and Grieve2000). Furthermore, infection-and-treatment protocols using the corresponding sporozoites induce protection against each of these diseases (Radley et al. Reference Radley, Brown, Cunningham, Kimber, Musisi, Payne, Purnell, Stagg and Young1975; Roestenberg et al. Reference Roestenberg, McCall, Hopman, Wiersma, Luty, van Gemert, van de Vegte-Bolmer, van Schaijk, Teelen, Arens, Spaarman, de Mast, Roeffen, Snounou, Renia, van der Ven, Hermsen and Sauerwein2009).
Poultry coccidiosis
Eimeria spp. are coccidia of major economic importance to poultry industries around the world. These fecal-oral pathogens have a direct life cycle. Oocysts are excreted in feces and sporulate upon exposure to air. Ingested oocysts excyst in the gastrointestinal tract to release sporozoites, which invade host enterocytes. Each species undergoes a set number of asexual replication cycles within the intestinal tract, ultimately producing gametocytes that combine sexually and become oocysts (McDougald, Reference McDougald1998).
Live oocysts of various Eimeria spp. are purified from the feces of birds used for vaccine production. On farms, oral inoculation of hatchlings with mixed species of coccidial oocysts is used to induce protective immunity. Today, most if not all live coccidiosis vaccines are recommended to be applied without use of anticoccidial drugs, and thus they rely solely upon the birds’ ability to mount an effective immune response before serious superinfections can result from re-exposure to organisms in litter. Nevertheless, organism strains used in live vaccines are selected for their continuing sensitivity to anticoccidial drugs. Although now falling out of favour, a common practice was to vaccinate birds and then add low levels of coccidiostatic drugs to feed or water (i.e. infection-and-treatment) (reviewed by Williams, Reference Williams2002).
Vaccinal protection from disease results from a combination of vaccine-induced immunity, immunological boosting from environmental re-exposure to vaccinal and wild type organisms, and displacement or dilution of coccidiostat-resistant strains in litter that otherwise may be prevalent (presumably this is in case anticoccidial treatment should become necessary). Because meat-producing birds have such short lifespans, induction of short-term immunity may be all that is required of a vaccine for broilers.
Live attenuated vaccines (see Table 2)
A superior concept to controlled-exposure or infection-and-treatment strategies, when possible, is to develop vaccines that contain live but attenuated organisms that are unlikely to cause disease. The best performing protozoal vaccines have this design. Vaccinal organisms are sometimes manufactured in vivo, including many babesiosis vaccines and all attenuated coccidiosis vaccines; clearly, those production strategies are unsuitable for adaptation to purely human conditions. However, several attenuated vaccines are produced in vitro, have been attenuated using repeatable methods, and show little evidence of vaccine-associated adverse events; similar designs merit strong consideration for development of protozoal vaccines for human diseases.
Bovine tropical theileriosis
The causative organism, Theileria annulata, is a blood-borne apicomplexan parasite that is transmitted by an arthropod vector (Hyalomma ticks). Disease commonly occurs in highly susceptible B. taurus cattle in countries bordering the Mediterranean Sea and extending southward into Sudan and eastward into China and India.
Organisms are transmitted to cattle by ticks to invade, replicate within, and cause clonal expansion of macrophages and lymphocytes, prior to invading erythrocytes from whence they are ingested by other ticks. This species of Theileria is adaptable to in vitro culture within bovine macrophages or lymphoid cells. Many strains have become attenuated simply by prolonged passage in cell culture (Boulter and Hall, Reference Boulter and Hall1999; Gubbels et al. Reference Gubbels, Viseras, Habela and Jongejan2000). Attenuation has been associated with loss of expression of parasite-induced matrix metalloproteinases, which hinders systemic dissemination of organisms (Hall et al. Reference Hall, Ilhan, Kirvar, Wilkie, Preston, Darghouth, Somerville and Adamson1999). Although vaccine strains may cause cryptic infections, latent organisms are incapable of transmission to ticks (Gubbels et al. Reference Gubbels, Viseras, Habela and Jongejan2000).
High rates of vaccine efficacy (>90%) and safety (100%) have been claimed (Zhang, Reference Zhang1997; Boulter and Hall, Reference Boulter and Hall1999) with little if any evidence of reversion to virulence. Immunity is cross-protective among strains (Boulter and Hall, Reference Boulter and Hall1999). Vaccinal protection endures at least 19 months in the field (Yin et al. Reference Yin, Luo and Lu2008), although there is room to question whether the duration of vaccine efficacy might depend upon repeated exposure to infected ticks.
Similar vaccines for Theileria lestoquardi (Theileria hirci) are used in Iran, Iraq and Bulgaria to protect sheep from malignant ovine theileriosis (Hooshmand-Rad, Reference Hooshmand-Rad1985; Lawrence, Reference Lawrence1997; Ali et al. Reference Ali, Beyer, Bakheit, Kullmann, Salih, Ahmed and Seitzer2008).
Toxoplasmosis abortion
An attenuated vaccine prevents Toxoplasma gondii-induced abortion in sheep (Buxton, Reference Buxton1993). This vaccine has been in continuous production since 1988 and is currently marketed in New Zealand and parts of Europe. It contains the S48 strain of T. gondii, which lost the ability to transform into the bradyzoite stage during years of twice-weekly intraperitoneal passage of tachyzoites in mice – such rapid passage did not allow time for stage conversion to form bradyzoites, and the strain lost this ability (Buxton and Innes, Reference Buxton and Innes1995). For the commercial production of the vaccine, the S48 strain is now maintained in tissue culture.
Ewes are vaccinated once, at least 3 weeks before breeding. Vaccination causes a transient fever and then the infection is cleared, unlike natural infections in which bradyzoites form latent intracellular cysts within brain and muscle. The inability to encyst makes the vaccine acceptable to use in a food-producing animal; otherwise, vaccine organisms in raw tissues could transmit infections to people and cats.
Vaccinal protection from toxoplasmosis abortion is strong against heterologous challenge with ingested oocysts (Wilkins et al. Reference Wilkins, O'Connell and Te Punga1988), which has been tested out to 18 months post vaccination (Buxton et al. Reference Buxton, Thomson, Maley, Wright and Bos1993). The manufacturer recommends revaccination in 2 years.
There are product warnings against vaccination of ewes during pregnancy, and because toxoplasmosis is a zoonotic disease there are further warnings about accidental human inoculation, particularly of pregnant women or immunosuppressed individuals. The author has been unable to find information to suggest that an adverse event may have occurred in people or that abortions in ewes may have been caused by inadvertent vaccination during pregnancy; this absence of published adverse events, after 25 years of commercial use, suggests (but does not prove) that despite the hypothetical concerns, any risks associated with the vaccine may in fact be minimal.
Bovine babesiosis
Babesia spp. are apicomplexan parasites of erythrocytes that are transmitted by tick vectors. Infection causes a febrile haemolytic disease. Morbidity and mortality can be particularly severe in B. taurus cattle.
Many bovine babesiosis vaccines are currently produced by governmental organizations around the world. Most are produced in vivo within splenectomized calves and contain Babesia bovis, Babesia bigemina, or both species. Many babesiosis vaccines may induce clinical disease; for example, a Cuban vaccine (Alonso et al. Reference Alonso, Blandino, Mendoza, Savon and Camacho1994) was reported to have a 2% rate of post-vaccination incidents. Vaccine risks are greater in adults (mirroring natural disease risks), so vaccinations are typically restricted to juveniles. Despite these limitations, babesiosis vaccines have been widely used in tropical and subtropical regions and have greatly reduced the incidence of disease losses (Callow et al. Reference Callow, Dalgliesh and de Vos1997).
A babesiosis vaccine currently in use in Argentina merits special attention because it is produced in vitro within bovine erythrocytes (Mangold et al. Reference Mangold, Vanzini, Echaide, de Echaide, Volpogni and Guglielmone1996), although it is not the only babesiosis vaccine to be cultured in this way (reviewed by Shkap and Pipano, Reference Shkap and Pipano2000). Millions of doses of the combined B. bovis – B. bigemina vaccine have been used in Argentina, with failure of protection documented in 0·09% of vaccinated cattle (Echaide, Reference Echaide2008). The B. bovis fraction is known to have lost the ability to be transmitted to tick vectors.
In Europe, bovine babesiosis is caused by Babesia divergens, and vaccines have been produced for that pathogen (reviewed by Bock et al. Reference Bock, Jorgensen and Molloy2008; Zintl et al. Reference Zintl, Mulcahy, Skerrett, Taylor and Gray2003). Many methods of attenuation and production were reported including in vivo within splenectomized calves and also within Mongolian gerbils (Meriones unguiculatas), or in vitro within erythrocytes. The use of gerbils for vaccine production was thought to have biosecurity and safety advantages over use of calves, however no more than 200 vaccine doses could be generated from a single gerbil. The apparent paucity of B. divergens vaccines available at present may have been influenced in part by the bovine spongiform encephalopathy epidemic in Europe and heightened concerns about disease transmission risks of bovine biological products.
An attenuated vaccine for Babesia ovis has been used to protect sheep in Bulgaria (Lawrence, Reference Lawrence1997).
Precocious coccidiosis vaccines
An alternative to infection-and-treatment coccidiosis immunization protocols is the use of attenuated vaccines. The elegant ‘precocious’ method of attenuation was first described for Eimeria tenella (Jeffers, Reference Jeffers1975), and the same method has been effectively applied to multiple species of poultry coccidia. Precocious strains of Eimeria spp. are selected by collecting the earliest forming oocysts from each infection, using those oocysts to infect the next subject, collecting the earliest oocysts again, and so on for multiple generations. Prepatent periods become shortened; this effect can be the result of the loss of one or more asexual cycles in the intestinal tract prior to gametogony (McDougald and Jeffers, Reference McDougald and Jeffers1976), or by a shortening of the time that each schizont takes to develop, and/or by a reduction in the size of schizonts and number of progeny within them (McDonald and Shirley, Reference McDonald and Shirley2009).
Reduced asexual reproduction of precocious strains in the intestinal tract leads to less mucosal damage, fewer oocysts produced, and a corresponding reduction in the number of oocysts that accumulate in the environment (McDonald and Shirley, Reference McDonald and Shirley2009). A manufacturing drawback is that because fewer precocious oocysts are produced in comparison with wild-type infections, a greater number of birds must be used for in vivo production of vaccinal oocysts and this increases production costs.
Precocious attenuation is a repeatable achievement and many stable strains have been developed. The prototype Wis-F strain of E. tenella could not be induced to revert to a longer prepatent period or virulence even when it was subjected to a relaxed selection protocol for 25 generations (Jeffers, Reference Jeffers1975).
The precocious selection concept has recently been adapted to create Eimeria spp. vaccine strains for rabbits (Pakandl, Reference Pakandl2005; Akpo et al. Reference Akpo, Kpodekon, Djago, Licois and Youssao2012), although it is unclear whether any have progressed to commercial use or regional distribution.
Vaccines containing whole killed protozoa (see Table 3)
Only four examples were found of commercially marketed protozoal vaccines containing whole killed organisms. All of these vaccines incorporate adjuvants and require two initial immunizations. Although these products are safe, they induce at best only partial protection for a brief period of time. However, even brief protection may have useful niche applications, such as vaccination prior to the breeding season to reduce a sexually transmitted disease of cattle (trichomoniasis). Two of these products have been withdrawn from the market.
Vaccines containing subunit antigens (see Table 4)
Only a few examples were found of commercially marketed protozoal vaccines based on defined or extracted antigens. All of the products in this class can be referred to as subunit vaccines; however, most of them are not recombinant antigen vaccines, because the vaccinal antigens are not derived from artificial synthesis or from transfection of other organisms such as bacteria, yeast, insect cells or viral vectors. All subunit protozoal vaccines incorporate adjuvants and require two or three initial immunizations.
Evidence of field efficacy has been published for two subunit protozoal vaccines (Leishmune and Coxabic). Unfortunately, the single example of a true recombinant antigen vaccine (Leish-Tec) has no documentation of field efficacy (from PubMed and Google searches or from the manufacturer's website).
Canine visceral leishmaniasis
Dogs and other canids are important reservoir hosts of Leishmania infantum, one of the pathogens of visceral leishmaniasis (canine and human). The pathogen is transmitted by sandflies when obtaining blood meals. In Brazil, a commercial vaccine for dogs (Leishmune) contains Fucose-Mannose-Ligand glycoprotein antigen derived from axenic culture of L. donovani (not L. infantum), administered with saponin adjuvant. This antigen complex plays a role in the entry of parasites into host phagocytes, a prerequisite for parasite replication and trafficking.
In field trials, vaccination with Leishmune reduced the incidence of disease in dogs over several years (Borja-Cabrera et al. Reference Borja-Cabrera, Correia Pontes, da Silva, Paraguai de Souza, Santos, Gomes, Luz, Palatnik and Palatnik de Sousa2002; Nogueira et al. Reference Nogueira, Moreira, Borja-Cabrera, Santos, Menz, Parra, Xu, Chu, Palatnik-de-Sousa and Luvizotto2005). Importantly, experimental evidence indicates that the vaccine helps to block transmission of L. infantum from dogs to sandfly vectors (Saraiva et al. Reference Saraiva, de Figueiredo Barbosa, Santos, Borja-Cabrera, Nico, Souza, de Oliveira Mendes-Aguiar, de Souza, Fampa, Parra, Menz, Dias, de Oliveira and Palatnik-de-Sousa2006). These properties are consistent with epidemiological evidence associating vaccination of dogs with statistically significant reductions of human visceral leishmaniasis (Palatnik-de-Sousa et al. Reference Palatnik-de-Sousa, Silva-Antunes, Morgado Ade, Menz, Palatnik and Lavor2009).
In addition to vaccination of dogs, public health programmes in Brazil include identification and removal of Leishmania-infected dogs. This complicates epidemiological analysis, so further, public-sponsored investigations are merited to more clearly distinguish between the degree of human protection that is attributable to vaccination of dogs and to culling of infected dogs, respectively.
A similar Leishmania vaccine for dogs (CaniLeish) has been provisionally licensed in parts of Europe (EMA, 2011). The vaccine contains Excreted Secreted Proteins (ESP) of L. infantum. Pre-licensing experiments, using ESP in muramyl dipeptide as adjuvant, demonstrated protection from infectious challenge for at least 8 months (Lemesre et al. Reference Lemesre, Holzmuller, Cavaleyra, Goncalves, Hottin and Papierok2005), and a double-blind field trial of approximately 400 dogs showed 92% efficacy in preventing infections over a 2-year period (Lemesre et al. Reference Lemesre, Holzmuller, Goncalves, Bourdoiseau, Hugnet, Cavaleyra and Papierok2007). The provisionally licensed formulation of CaniLeish contains saponin adjuvant instead of muramyl dipeptide.
A third vaccine for canine leishmaniasis, licensed in Brazil since 2007 (Leish-Tec), is perhaps the only marketed recombinant antigen vaccine for any protozoal disease. It contains a Leishmania amastigote antigen (A2) purified from transfected Escherichia coli. A small trial showed partial short-term protection of vaccinated dogs from a severe infectious challenge (Fernandes et al. Reference Fernandes, Costa, Coelho, Michalick, de Freitas, Melo, Luiz Tafuri, Resende Dde, Hermont, Abrantes Cde and Gazzinelli2008). There appear to be no further published investigations of efficacy.
Coccidiosis of chickens
A subunit vaccine containing two native antigens extracted from Eimeria maxima macrogametocytes is marketed for vaccination of hens to provide passive immunization of chicks (Coxabic) (reviewed by Sharman et al. Reference Sharman, Smith, Wallach and Katrib2010). Organisms used in vaccine production are produced in vivo in chickens and are extracted from the intestinal tract. Maternal antibody is passed via the egg to hatchlings.
Passive immunity engendered by Coxabic is sufficient to enable offspring to perform well and to transition towards active immunity against mixed Eimeria spp. as a result of ubiquitous natural exposure. Vaccine efficacy has also been inferred from production data of large-scale field trials in which growth and mortality were similar between the offspring of vaccinated hens and the offspring of unvaccinated hens in which coccidiosis had been managed using traditional industry practices (i.e. prophylactic coccidiostatic drugs in feed, and/or use of live vaccines) (Wallach et al. Reference Wallach, Ashash, Michael and Smith2008). Because of its manner of production and application, Coxabic is more expensive per dose than are other types of coccidiosis vaccines, however each vaccinated hen passes immunity to numerous offspring.
Canine babesiosis
A vaccine for canine babesiosis, containing soluble protein antigens derived from in vitro cultures of Babesia canis and Babesia rossi, was demonstrated to ameliorate the severity of infectious challenge with heterologous B. canis for up to 6 months after vaccination (Schetters et al. Reference Schetters, Kleuskens, Scholtes, Gorenflot, Moubri and Vermeulen2001, Reference Schetters, Kleuskens, Scholtes, van de Crommert, Krijnen, Moubri, Gorenflot and Vermeulen2006). The infectious challenge in these experiments was severe, having been induced by intravenous inoculation of infected erythrocytes rather than by the bite of infected ticks; infectious challenge experiments that are overly harsh have the potential to mask the protective effect of a vaccine. In these experiments, haematocrit values dropped 54% in vaccinated dogs compared with a 65% drop in unvaccinated controls, and clinical scores also showed modest but statistically significant improvements (Schetters et al. Reference Schetters, Kleuskens, Scholtes, van de Crommert, Krijnen, Moubri, Gorenflot and Vermeulen2006).
The vaccine was marketed in parts of Europe, but unfortunately there was no published epidemiological study and the product is no longer in production. Therefore, it is unclear whether the vaccine may have prevented or reduced transmission from ticks, or if the effects were limited to a reduction in the severity of disease as in the challenge experiments.
Comparisons across vaccine classes
Propagation of organisms
Perhaps the single most obvious conclusion is that the production of all effective protozoal vaccines is dependent upon growth of protozoal organisms, either in vitro or in vivo; this includes production of organisms within arthropod vectors. Effective vaccines don't always contain whole organisms, but even native subunit vaccines require culture of organisms for extraction of antigens. The author has found only one commercially available recombinant antigen vaccine, but no evidence has been published to support the efficacy of that vaccine in the field. There are no licensed protozoal vaccines based on DNA or viral vectors.
This conclusion has a profound implication: for any protozoal disease to be controlled by a vaccine, methods must be developed to cultivate the pathogen. Although breaking this paradigm is a legitimate goal of protozoal vaccine science, vaccine designs with this goal carry a high risk of failure.
The value of living organisms
A single inoculation of living organisms, whether virulent or attenuated, tends to create strong, long-lasting protection against most protozoal diseases. The advantage of great efficacy is tempered by legitimate safety concerns, even though many attenuated vaccines have excellent safety records. A major rationale for research of novel protozoal vaccine designs for human diseases has been that live vaccines carry unacceptable risks; however, while many novel vaccines have been safe they have not been effective. Moving forward, new emphasis should be placed upon the development of novel methods to improve the safety of live vaccines, which can reasonably be expected to be effective.
Uses for short-term protection
Killed and subunit vaccines require adjuvants, booster inoculations, and annual or semiannual revaccination. The relatively short duration of protection is a disadvantage. However, residents of highly endemic regions may only need vaccinal immunity in the short term, and then naturally acquired immunity could take over as a result of frequent natural exposure. An example of this is vaccination of hens to provide passive protection of chicks from coccidiosis (Sharman et al. Reference Sharman, Smith, Wallach and Katrib2010).
Mucosal vaccines
In relation to mucosal pathogens, the endurance of effective immunity that may be attributed solely to live vaccination is unclear and is worthy of further research. For example, the true duration of effective immunity resulting solely from application of a poultry coccidiosis vaccine is obscured by the daily natural exposure of production birds to coccidia in litter (Williams et al. Reference Williams, Johnson and Andrews2000). Although this distinction may be unimportant for the control of coccidiosis in poultry, the duration of protection of mucosal vaccines in the absence of frequent endemic exposure is an important consideration for development of future veterinary or human vaccines against diseases such as giardiasis, cryptosporidiosis, amoebiasis, microsporidiosis and trichomoniasis.
Parenteral vaccination with killed mucosal pathogens appears to provide modest, short-lived protection at most, and has been inadequately investigated (Lehmann and Lehmann, Reference Lehmann and Lehmann2004; Baltzell et al. Reference Baltzell, Newton and O'Connor2013).
EXPERIMENTAL PROTOZOAL VACCINES
Experimental vaccines containing organisms
Table 5 gives details of several noteworthy vaccination experiments that, although often highly effective, have not (or have not yet) resulted in a marketed vaccine. All of these experiments were based on live, attenuated, or whole-killed protozoal organisms. The results of these studies carry great predictive value because each was performed using a natural host–parasite relationship.
Two human malaria trials show protective efficacy for infection-and-treatment (Roestenberg et al. Reference Roestenberg, McCall, Hopman, Wiersma, Luty, van Gemert, van de Vegte-Bolmer, van Schaijk, Teelen, Arens, Spaarman, de Mast, Roeffen, Snounou, Renia, van der Ven, Hermsen and Sauerwein2009) and whole-organism protocols (Seder et al. Reference Seder, Chang, Enama, Zephir, Sarwar, Gordon, Holman, James, Billingsley, Gunasekera, Richman, Chakravarty, Manoj, Velmurugan, Li, Ruben, Li, Eappen, Stafford, Plummer, Hendel, Novik, Costner, Mendoza, Saunders, Nason, Richardson, Murphy, Davidson, Richie, Sedegah, Sutamihardja, Fahle, Lyke, Laurens, Roederer, Tewari, Epstein, Sim, Ledgerwood, Graham and Hoffman2013); these experimental immunization protocols have counterparts among veterinary vaccines, and closely analogous experiments using avian malaria essentially predicted these results as much as a century earlier (Sergent and Sergent, Reference Sergent and Sergent1910; Richards, Reference Richards1966). Although an intravenously administered irradiated vaccine, recently examined by Seder et al. (Reference Seder, Chang, Enama, Zephir, Sarwar, Gordon, Holman, James, Billingsley, Gunasekera, Richman, Chakravarty, Manoj, Velmurugan, Li, Ruben, Li, Eappen, Stafford, Plummer, Hendel, Novik, Costner, Mendoza, Saunders, Nason, Richardson, Murphy, Davidson, Richie, Sedegah, Sutamihardja, Fahle, Lyke, Laurens, Roederer, Tewari, Epstein, Sim, Ledgerwood, Graham and Hoffman2013), is described as being metabolically active, the experimental results compare closely with killed vaccines investigated in chickens (Richards, Reference Richards1966). These investigations need to be progressed further, to include heterologous infectious challenges at meaningfully long intervals after immunization.
An attenuated vaccine to prevent cats from disseminating T. gondii oocysts was a remarkable scientific success but was not commercially viable. The vaccine was designed to reduce transmission from cats to other animals and humans. The T-263 strain of T. gondii was developed by chemical mutagenesis and selection for the inability to undergo sexual recombination (Frenkel et al. Reference Frenkel, Pfefferkorn, Smith and Fishback1991). The orally administered vaccine demonstrated high efficacy in infectious challenge experiments of cats (Frenkel et al. Reference Frenkel, Pfefferkorn, Smith and Fishback1991; Freyre et al. Reference Freyre, Choromanski, Fishback and Popiel1993). Additionally, in an ambitious 3-year field trial, cats were trapped and vaccinated on swine farms. The number of cats observed to be shedding oocysts and the T. gondii seroprevalence of pigs and rodents on farms decreased significantly (Mateus-Pinilla et al. Reference Mateus-Pinilla, Dubey, Choromanski and Weigel1999), consistent with decreased oocyst contamination of farms. Although effective, this vaccine would have been expensive to manufacture and distribute, and difficult to market.
Field evidence was obtained for efficacy of a vaccine to prevent human cutaneous leishmaniasis in Ecuador, which contained three local isolates of Leishmania spp. promastigotes that were phenol-killed and administered with BCG adjuvant. Vaccinated and control subjects were monitored for a year, and cutaneous leishmaniasis occurred in 2% and 8%, respectively (Armijos et al. Reference Armijos, Weigel, Aviles, Maldonado and Racines1998). The vaccine required a booster and annual revaccination (Armijos et al. Reference Armijos, Weigel, Romero, Garcia and Salazar2003). Injection site swelling and mild fever were frequently observed especially following the second application; this could have been caused by reaction to Leishmania spp. antigens or to the BCG adjuvant. The vaccine does not appear to have been brought forward for commercial or governmental use. Another killed vaccine for human cutaneous leishmaniasis was recently trialled in Brazil and positive results were reported (Mayrink et al. Reference Mayrink, Mendonca-Mendes, de Paula, Siqueira, Marrocos Sde, Dias, de Andrade and Machado-Coelho2013).
An attenuated vaccine for histomoniasis of turkeys and chickens has been developed by researchers in Austria. The vaccinal strain became attenuated when organisms were passaged every 2–3 days in axenic broth culture for 295 times. The vaccine is effective when administered into the crop or into the cloaca, and provides strong protection in challenge experiments, at least against low passage virulent organisms of the parent strain (Liebhart et al. Reference Liebhart, Windisch and Hess2010, Reference Liebhart, Sulejmanovic, Grafl, Tichy and Hess2013). Histomoniasis is re-emerging as a cause of poultry losses, in part because the prophylactic use of effective antimicrobials has been restricted or banned. Practical issues to overcome in order to commercialize this type of product will probably include the economics of transportation and administration of cryopreserved vaccines, which must be inexpensive to be used in poultry.
Experimental molecular malaria vaccines
Table 6 compares results of animal models and human trials for several prominent types of experimental malaria vaccines. Malaria has been the dominant category of protozoal vaccine research in recent history. All of the vaccines in Table 6 were based on novel molecular designs.
In each comparison in Table 6, the positive results obtained in animal models exceeded the results obtained in humans. The following factors help explain the poor predictive value of those experiments: (1) Reliance upon artificially adapted host–parasite models of malaria; (2) Investigation of unreasonably short intervals between vaccination and infectious challenge experiments; (3) Use of homologous organisms in infectious challenge experiments; (4) Vaccine designs that disregard the history of veterinary protozoal vaccines. These four factors are discussed in greater detail in the following sections.
The importance of animal model selection
All of the animal malaria models in Table 6 are artificial combinations of host and parasite. Natural malarias have a period of acute illness, during which mortality may occur in a minority of individuals, typically followed by recovery with prolonged splenomegaly, frequent development of latent infections, and the possibility of disease relapse (Garnham, Reference Garnham1966; Miller et al. Reference Miller, Good and Milon1994). Metaphorically, there is a standoff between host and parasite rather than absolute victory for either one. In contrast, artificially adapted malaria models tend to behave in a ‘winner-takes-all’ manner that does not resemble naturally evolved malaria relationships.
Laboratory rodent-adapted Plasmodium spp. are natural parasites of Thamnomys and Grammomys spp. of thicket rats (Vincke and Lips, Reference Vincke and Lips1948; Cox, Reference Cox, Wernsdorfer and McGregor1988), native to montane forests of central Africa. The ancestral lineage of these rodents diverged from that of the house mouse over 10 million years ago (Lecompte et al. Reference Lecompte, Aplin, Denys, Catzeflis, Chades and Chevret2008). The natural hosts are well adapted and do not suffer high mortality (Garnham, Reference Garnham and Kreier1980). In contrast, experimental malaria in laboratory mice has high mortality, with many mouse–parasite combinations being completely lethal. Rescue of infected mice tends to cause sterile recovery (i.e. without chronic or latent infections) (Ishih et al. Reference Ishih, Kawakami, Todoroki, Hirai, Ohori and Kobayashi2013). This is a winner-takes-all scenario.
Natural hosts of Plasmodium knowlesi include long-tailed macaques (Macaca fascicularis) and pig-tailed macaques (Macaca nemestrina), in which the results of infection are variable and have low mortality (Butcher et al. Reference Butcher, Mitchell and Cohen2010). Rhesus monkeys (Macaca mulatta) are not natural hosts of P. knowlesi, and infection in them induces fulminating parasitaemia usually resulting in death (Collins, Reference Collins, Wernsdorfer and McGregor1988), similar to Plasmodium berghei infection of mice. This is another winner-takes-all scenario.
Infection of owl monkeys (Aotus spp.) with the human pathogen Plasmodium falciparum creates another winner-takes-all scenario (Sadun et al. Reference Sadun, Wellde and Hickman1969).
In artificial winner-takes-all relationships, relatively minor treatments may cause a radical shift between the only two major outcomes, death or sterile recovery. Researchers may mistakenly believe that lethal models of malaria are severe tests for any vaccine, and that survival after vaccination is therefore evidence of great efficacy. In practice, a vaccine that reduces infection or moderates disease in any naturally evolved host–parasite relationship is more predictive of what may be expected in other animals with similar parasites, presumably including humans. A vaccine that ameliorates the course of naturally evolved malarias in Thamnomys rodents, pig-tailed macaques, canaries, or chickens, should be expected to have greater predictive value for human malaria than do vaccines that block mortality in mice, owl monkeys, or in other artificially adapted animal models.
Short testing intervals
Data for the protective efficacy of most vaccines should be assessed over a range of intervals that extend into a useful duration. Short-lived immunity may be adequate for poultry coccidiosis or seasonal breeding of cattle; however, vaccines for systemic illnesses such as malaria should be tested at least to 6 months, and preferably to a year or more.
Homologous vs heterologous infectious challenge
To be effective in the field, vaccines need to protect against a variety of organism isolates. When possible, vaccines should be tested by challenge with heterologous organisms, rather than with the same strain from which the vaccine was manufactured. Research efforts should include acquisition of varied isolates for any pathogen under investigation. Ultimately, efficacy must be assessed in field trials within endemic regions.
Vaccine designs that are useful in animals
There is a reason why veterinary medicine hasn't created a bevy of recombinant antigen vaccines for the prevention of protozoal diseases of animals – they haven't been sufficiently effective.
This is not to imply that such vaccine designs could never work, or that transformational new knowledge will never open a floodgate of effective vaccines based on novel technological advancements. Potentially transformative but technologically difficult and unproven vaccine designs should be investigated first in naturally occurring diseases of animals, not in artificial models. Translation of a novel design principle to human vaccines should be attempted after it has been shown to be effective against a natural animal–parasite relationship. If science cannot create an effective recombinant antigen or plasmid vaccine to prevent infection of (for example) rats with T. gondii, deer mice with Babesia microti, or sparrows with Plasmodium relictum, then it seems rather naive to expect such designs to be effective against human protozoal diseases.
Funding for veterinary medical research is paltry in comparison to human disease research. Human medical research agencies should fund research of protozoal diseases of animals in order to develop improved vaccine methods and investigate new paradigms. At the same time, protozoal vaccines for people should be developed by adapting currently effective techniques from veterinary medicine.
ONE MEDICINE
The concept of ‘One Medicine’ includes the beneficial flow of knowledge and techniques from human medicine to veterinary medicine, and from veterinary medicine to human medicine. However, because of the dominance of human medical research funding, the flow of information moves predominantly from human medicine to veterinary medicine. Human medicine is missing significant benefits that could be had by paying greater attention to veterinary knowledge and by supporting opportunities to investigate naturally occurring diseases of animals. This missed opportunity is vividly illustrated by the discordance between development of veterinary protozoal vaccines, of which there are many, and human protozoal vaccines, of which there are none.