Introduction
Metronidazole is a 5-nitroimidazole drug that has become the mainstay in the treatment of anaerobic infections worldwide and ranks amongst the ‘essential medicines’ as defined by the WHO. It was developed in 1959 (Cosar and Julou, Reference Cosar and Julou1959) specifically for the treatment of trichomoniasis, an infection of the genital tract caused by the microaerophilic parasite Trichomonas vaginalis that was notoriously difficult to treat at that time. Although metronidazole is a synthetic drug, its basic structure derives from 2-nitroimidazole, or azomycin, which had been isolated from Streptomyces sp. or other closely related bacteria a few years earlier (Maeda et al. Reference Maeda, Osato and Umezawa1953). Several independent studies quickly confirmed the imposing effectivity of metronidazole, then already being sold under its brand name Flagyl®, against T. vaginalis (Durel et al. Reference Durel, Couture, Collart and Giro1960; Nicol et al. Reference Nicol, Barrow and Redmond1960; Rodin et al. Reference Rodin, King, Nicol and Barrow1960). Soon thereafter, the suitability of metronidazole for the treatment of other microaerophilic parasites, i.e. Giardia lamblia (Schneider, Reference Schneider1961) and Entamoeba histolytica (Powell et al. Reference Powell, MacLeod, Wilmot and Elsdon-Dew1966), was demonstrated. Metronidazole proved to be active against anaerobic and microaerophilic bacteria as well, as shown for Clostridium spp. (Freeman et al. Reference Freeman, McFadzean and Whelan1968; Füzi and Csukás, Reference Füzi and Csukás1969a), Fusobacterium fusiforme (Füzi and Csukás, Reference Füzi and Csukás1969b), Bacteroides fragilis (Nastro and Finegold, Reference Nastro and Finegold1972) and against Helicobacter pylori (Hirschl et al. Reference Hirschl, Hentschel, Schütze, Nemec, Pötzi, Gangl, Weiss, Pletschette, Stanek and Rotter1988). Indeed, metronidazole is active against the vast majority of anaerobic and microaerophilic pathogens, rendering it an indispensable weapon in our antimicrobial arsenal (Table 1).
Table 1. Human infections treated with metronidazole

Despite its frequent use over such a long period of time, metronidazole has remained a reliable drug for the treatment of most anaerobic/microaerophilic infections, thereby setting it apart from most other antimicrobials to which resistance develops much more quickly (Holmes et al. Reference Holmes, Moore, Sundsfjord, Steinbakk, Regmi, Karkey, Guerin and Piddock2016). This is undoubtedly attributable to its pleiotropic mode of action as it targets a large number of molecules in the cell, rather than only a few or even just a single one, as most antimicrobials do. In fact, metronidazole's mode of action is fiendishly simple: it enters the cell without the help of any transporting mechanisms and unfolds its destructive potential after having been reduced to its nitro group, a reaction which occurs only under very low oxygen concentrations.
Nevertheless, metronidazole resistance does occur in some pathogens more frequently than in others; and despite its overall high tolerability, metronidazole can cause unpleasant side-effects. Further, metronidazole and other 5-nitroimidazoles are still under discussion as being potentially carcinogenic. The present review will summarize the most important aspects of metronidazole and gives a comprehensive overview of resistance and safety issues.
Mode of action
Metronidazole uptake occurs without any specific mechanisms such as transporters but depends on metabolic activity ensuring an energized membrane (Müller and Gorrell, Reference Müller and Gorrell1983; Edwards, Reference Edwards1993). It is, as such, a prodrug which is poorly if at all reactive (Edwards, Reference Edwards1993). However, if the nitro group is reduced (Fig. 1) metronidazole is transformed into a reactive intermediate that reacts with multiple targets in the cell (Müller and Gorrell, Reference Müller and Gorrell1983). To date, it is still not fully clear which intermediate, determined by the number of electrons transferred to the nitro group, is the actual toxic form. Several propositions were made, ranging from the nitroradical anion stage (one electron transferred) (Lindmark and Müller, Reference Lindmark and Müller1976; Edwards, Reference Edwards1993; Kulda, Reference Kulda1999) to the nitroso stage (two electrons transferred) or the hydroxylamine stage (four electrons transferred) (Wardman, Reference Wardman1985; Leitsch et al. Reference Leitsch, Kolarich, Wilson, Altmann and Duchêne2007, Reference Leitsch, Kolarich, Binder, Stadlmann, Altmann and Duchêne2009, Reference Leitsch, Drinić and Duchêne2012a, Reference Leitsch, Schlosser, Burgess and Duchêneb). Importantly, metronidazole has a very low midpoint redox potential (−486 mV) (Smith and Edwards, Reference Smith and Edwards1995), thus well below the midpoint redox potential of NADPH and NADH (approximately −320 mV each), resulting in very small amounts of metronidazole being reduced in aerobes. Moreover, oxygen can re-oxidize the metronidazole nitroradical anion in a redox cycling reaction (Mason and Holtzman, Reference Mason and Holtzman1975), leading to the generation of superoxide anions and the re-established prodrug. In microaerophiles and anaerobes, however, intracellular oxygen concentrations are low and factors exist in abundance that are able to reduce metronidazole and, thereby, activate it to its toxic form. In the last three to four decades, several such factors were identified in different microaerophilic or anaerobic organisms. The first enzyme suggested to be relevant for metronidazole reduction was pyruvate:ferredoxin oxidoreductase (PFOR) (Lindmark and Müller, Reference Lindmark and Müller1976), which transfers, via its iron–sulphur clusters, electrons derived from pyruvate to the electron carrier protein ferredoxin, which also contains iron–sulphur clusters. Ferredoxin, in turn, has a very low midpoint redox potential (−430 mV) and can transfer electrons to the nitro group of metronidazole, thereby generating metronidazole nitroradical anions as can be readily measured by electron paramagnetic resonance spectroscopy (Moreno et al. Reference Moreno, Mason, Muniz, Cruz and Docampo1983, Reference Moreno, Mason and Docampo1984; Chapman et al. Reference Chapman, Cammack, Linstead and Lloyd1985; Lloyd and Pedersen, Reference Lloyd and Pedersen1985). Since the PFOR pathway exists in almost all anaerobes susceptible to metronidazole (Narikawa, Reference Narikawa1986), with possibly the exception of bifidobacteria, it was an obvious candidate for metronidazole activation in the living organism. About the same time, however, it was observed that also rat liver microsomes (Pervez-Reyes et al. Reference Pervez-Reyes, Kalyanaraman and Mason1980) or certain flavin enzymes, such as xanthine oxidase (Kedderis et al. Reference Kedderis, Argenbright and Miwa1988), can reduce metronidazole under anaerobic conditions. Indeed, several flavin enzymes have been described in microaerophiles and anaerobes to be involved in metronidazole reduction, including thioredoxin reductase (TrxR) in T. vaginalis (Leitsch et al. Reference Leitsch, Kolarich, Binder, Stadlmann, Altmann and Duchêne2009), E. histolytica (Leitsch et al. Reference Leitsch, Kolarich, Wilson, Altmann and Duchêne2007) and G. lamblia (Leitsch et al. Reference Leitsch, Burgess, Dunn, Krauer, Tan, Duchêne, Upcroft, Eckmann and Upcroft2011) and nitroreductase RdxA in H. pylori (Olekhnovich et al. Reference Olekhnovich, Goodwin and Hoffman2009). Many studies were conducted to identify the main activation pathways in anaerobic and microaerophilic pathogens. Surprisingly, downregulation or deactivation of PFOR in T. vaginalis (Leitsch et al. Reference Leitsch, Kolarich, Binder, Stadlmann, Altmann and Duchêne2009), Tritrichomoas foetus (Sutak et al. Reference Sutak, Dolezal, Fiumera, Hrdy, Dancis, Delgadillo-Correa, Johnson, Müller and Tachezy2004) or B. fragilis (Diniz et al. Reference Diniz, Farias, Carvalho, Rocha and Smith2004) had only a minimal effect, if any, on the susceptibility to metronidazole. An appreciable negative effect on metronidazole susceptibility, however, could be observed when PFOR was downregulated in G. lamblia (Dan et al. Reference Dan, Wang and Wang2000). In turn, overexpression of TrxR rendered G. lamblia somewhat more susceptible to metronidazole (Leitsch et al. Reference Leitsch, Müller and Müller2016). It has, however, proven impossible so far to pinpoint reduction of metronidazole to one single enzymatic pathway. Interestingly, even non-enzymatic reduction of metronidazole under anaerobic conditions by cysteine and ferrous iron was reported (Willson and Searle, Reference Willson and Searle1975). It is, therefore, safe to conclude that reduction of metronidazole in microaerophiles and anaerobes is performed by several factors, arguably some of which are non-enzymatic. This circumstance reduces the likelihood of emergence of metronidazole resistance in most organisms considerably. The only exception might be RdxA in H. pylori, which was identified as the major activating enzyme of metronidazole in several independent studies (Debets-Ossenkopp et al. Reference Debets-Ossenkopp, Pot, van Westerloo, Goddwin, Vandenbroucke-Grauls, Berg, Hoffman and Kusters1999; Jenks et al. Reference Jenks, Ferrero and Labigne1999a, Reference Jenks, Labigne and Ferrerob; Kwon et al. Reference Kwon, Hulten, Kato, Kim, Lee, El-Zaatari, Osato and Graham2001; Latham et al. Reference Latham, Labigne and Jenks2002).
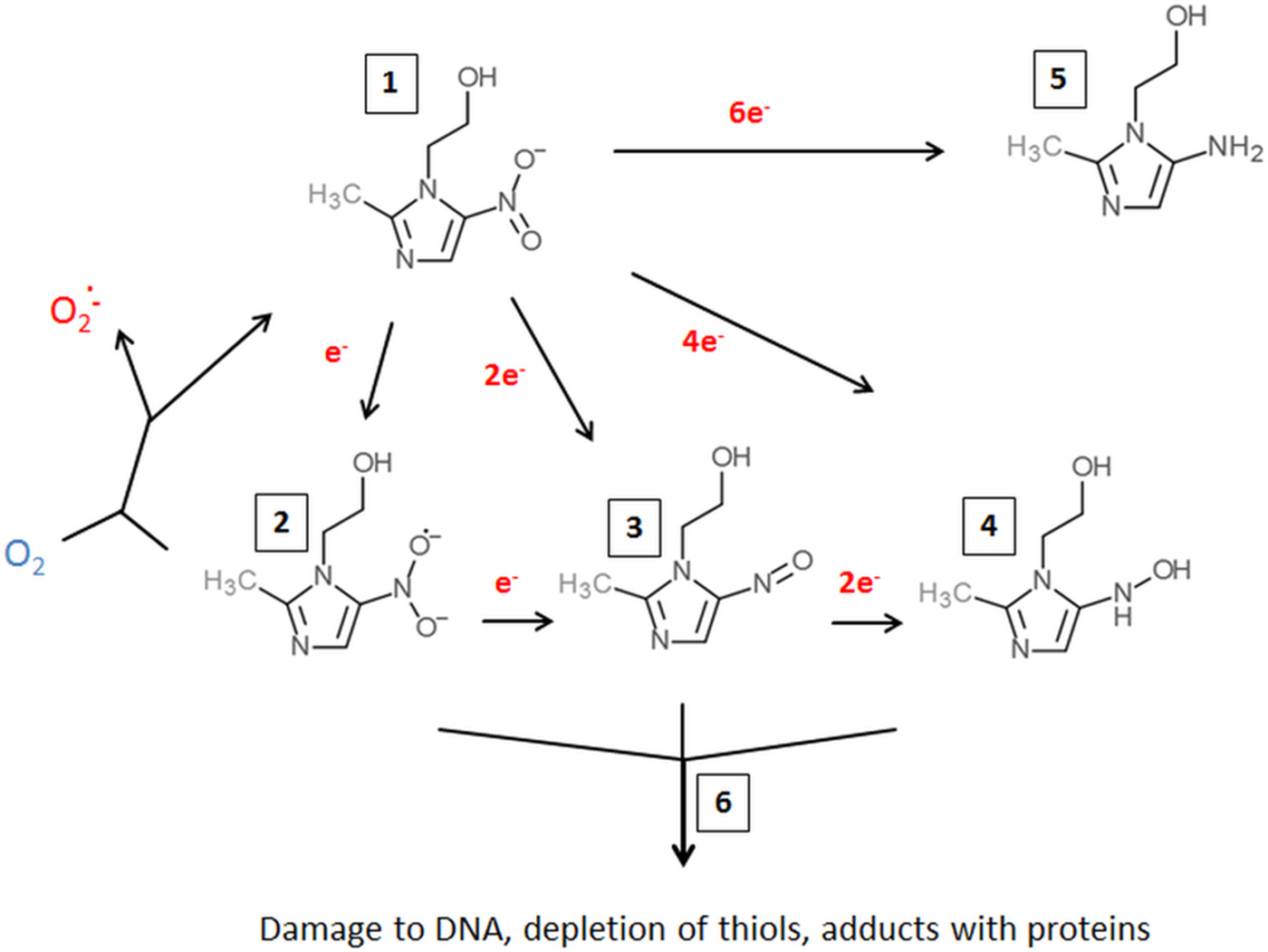
Fig. 1. Metronidazole reduction and toxicity in microaerophiles and anaerobes. Metronidazole enters the cell (1). Depending on the number of electrons transferred to the nitro group, a nitroimidazole radical anion (2), a nitrosoimidazole (3) or a hydroxylaminimidazole (4) is formed. Reduction can be either sequential, (2→3→4) or catalysed in one step. If oxygen is present, the nitroimidazole radical anion (2) is re-oxidized and the original metronidazole prodrug (1) re-established. Some enzymes (e.g. nitroreductase 2 from Giardia lamblia or Nim proteins from Bacteroides spp.) are proposed to detoxify metronidazole by transferring six electrons to the nitro group, thereby generating a non-reactive aminoimidazole (5). Reactive metronidazole intermediates (2–4) damage cell constituents such as DNA and proteins, and deplete thiol pools (6).
A fairly motley picture is also evident regarding the targets of metronidazole in susceptible organisms. Damage to DNA, including strand breaks, was reported from bacteria (Plant and Edwards, Reference Plant and Edwards1976) as well as parasites, e.g. T. vaginalis (Ings et al. Reference Ings, McFadzean and Ormerod1974) and G. lamblia (Uzlikova and Nohynkova, Reference Uzlikova and Nohynkova2014). In addition, 5-nitroimidazoles were shown to form adducts with nucleotides (LaRusso et al. Reference LaRusso, Tomasz, Kaplan and Müller1978; Ludlum et al. Reference Ludlum, Colinas, Kirk and Mehta1988) and cysteine (Wislocki et al. Reference Wislocki, Bagan, Vandenheuvel, Walker, Alvaro, Arison, Lu and Wolf1984; Leitsch et al. Reference Leitsch, Kolarich, Wilson, Altmann and Duchêne2007), an amino acid which is highly abundant in many anaerobes, both as non-protein thiol buffer and as constituent of proteins. Non-protein thiol buffers can be depleted in metronidazole-treated parasites through adduct formation (Leitsch et al. Reference Leitsch, Kolarich, Wilson, Altmann and Duchêne2007, Reference Leitsch, Kolarich, Binder, Stadlmann, Altmann and Duchêne2009, Reference Leitsch, Burgess, Dunn, Krauer, Tan, Duchêne, Upcroft, Eckmann and Upcroft2011; Williams et al. Reference Williams, Lloyd, Kolarich, Alagesan, Duchêne, Cable, Williams and Leitsch2012), thereby causing oxidative stress. Further, metronidazole–cysteine adducts can negatively affect the activity of certain enzymes, such as the disulphide/thioredoxin reductase activity of TrxR (Leitsch et al. Reference Leitsch, Kolarich, Wilson, Altmann and Duchêne2007, Reference Leitsch, Kolarich, Binder, Stadlmann, Altmann and Duchêne2009; Williams et al. Reference Williams, Lloyd, Kolarich, Alagesan, Duchêne, Cable, Williams and Leitsch2012). Thus, TrxR is a special case in this context as it functions, both, as an activator and as a target of metronidazole. Importantly, TrxR was identified as a target of metronidazole in four microaerophilic parasites, i.e. E. histolytica (Leitsch et al. Reference Leitsch, Kolarich, Wilson, Altmann and Duchêne2007), T. vaginalis (Leitsch et al. Reference Leitsch, Kolarich, Binder, Stadlmann, Altmann and Duchêne2009), Spironucleus vortens (Williams et al. Reference Williams, Lloyd, Kolarich, Alagesan, Duchêne, Cable, Williams and Leitsch2012) and G. lamblia (Leitsch et al. Reference Leitsch, Schlosser, Burgess and Duchêne2012b), whereas the other proteins affected by metronidazole treatment varied strongly between the parasites studied. The majority of these, however, were reported to interact with thioredoxin in anaerobes and other organisms, e.g. enolase, malate dehydrogenase and ribonucleotide reductase in T. vaginalis (Leitsch et al. Reference Leitsch, Kolarich, Binder, Stadlmann, Altmann and Duchêne2009), thereby underscoring a correlation between metronidazole action and the thioredoxin system. It is also interesting to note that metronidazole treatment in G. lamblia leads to the degradation of translation elongation factor 1-γ, a factor likely to be essential for cell viability (Leitsch et al. Reference Leitsch, Schlosser, Burgess and Duchêne2012b).
Pharmacokinetics and safety issues
Mostly, metronidazole is administered intravenously or orally, either in large single doses of 2 g or in smaller repeated doses (Ralph et al. Reference Ralph, Clarke, Libke, Luthy and Kirby1974). Treatment regimens vary with the condition treated. After a 2 g oral dose, the peak serum level in a female patient was 40 µg mL−1 and the half-life of elimination approximately 7 h (Wood and Monro, Reference Wood and Monro1975). When smaller doses are administered, peak serum levels are clearly lower, i.e. 11·5 µg mL−1 after oral administration of 500 mg and 6·2 µg mL−1 after oral administration of 250 mg (Ralph et al. Reference Ralph, Clarke, Libke, Luthy and Kirby1974). However, metronidazole can also be applied topically in ointments, e.g. for the treatment of rosacea (Korting and Schöllmann, Reference Korting and Schöllmann2009), a chronic inflammatory skin condition which is treatable with metronidazole for, as yet, unknown reasons.
In most cases, metronidazole is fairly well tolerated, but adverse effects, especially neurological, are not rare. Consumption of alcohol during metronidazole treatment and several days thereafter should be strictly avoided because it can strongly exacerbate side-effects such as nausea or stomach cramps. Indeed, the discomfort resulting from simultaneous intake of metronidazole and alcoholic beverages is so great that metronidazole was used as an adjuvant in the treatment of alcoholism (Semer et al. Reference Semer, Friedland, Vaisberg and Greenberg1966). Due to its reactivity with DNA (LaRusso et al. Reference LaRusso, Tomasz, Kaplan and Müller1978), metronidazole was soon assumed to be carcinogenic and teratogenic (Voogd, Reference Voogd1981). A teratogenic effect of metronidazole could not be established (Koss et al. Reference Koss, Baras, Lane, Aubry, Marcus, Markowitz and Koumans2012), but it was found to be carcinogenic in rodents after extended durations of highly dosed treatment. In man, results were less clear and often conflicting (Dobiás et al. Reference Dobiás, Cerná, Rössner and Srám1994). With regard to short-term treatment with metronidazole, originally no correlation between metronidazole intake and cancer was found (Falagas et al. Reference Falagas, Walker, Jick, Ruthazer, Griffith and Snydman1998), but more recent studies report on a limited correlation (Friedman et al. Reference Friedman, Jiang, Udaltsova, Quesenberry, Cha and Habel2009). As a consequence, metronidazole is officially classified as ‘reasonably anticipated to be a human carcinogen’.
Metronidazole resistance
Due to metronidazole's multifaceted and pleiotropic mode of action and its ability to enter cells without the need for a specific transport mechanism, the emergence of resistance is, on the whole, far less common than seen with other antimicrobials (Holmes et al. Reference Holmes, Moore, Sundsfjord, Steinbakk, Regmi, Karkey, Guerin and Piddock2016). However, metronidazole resistance is observed in the field, with varying frequency and depending on the pathogen concerned. Importantly, treatment failures with metronidazole are not necessarily due to drug resistance as such but can also be attributable to reinfections or caused by poor drug availability in the host (Nash, Reference Nash2001). Other microbes inhabiting the same niches as the pathogens can also modulate the efficacy of metronidazole treatment (Nagy and Földes, Reference Nagy and Földes1991). In laboratory research, resistance to metronidazole can be generated in stocks of most parasites or bacteria. This, however, can give rise to phenotypes which are not viable in the host (Tejman-Yarden et al. Reference Tejman-Yarden, Millman, Lauwaet, Davids, Gillin, Dunn, Upcroft, Miyamoto and Eckmann2011). Interestingly, several features of metronidazole resistance seem to be shared in bacteria and protists, despite the large evolutional distance between these kingdoms of life. Unfortunately, however, there has been little cooperative research between bacteriologists and protistologists on this particular issue, so that our understanding of metronidazole resistance has remained fairly incomplete despite the strong efforts undertaken by a large number of individual research groups. Nevertheless, in several microorganisms underlying mechanisms have been described in detail and, gradually, a more complete picture is evolving (Tables 2 and 3).
Table 2. Overview over established factors involved in metronidazole resistance in protist parasites
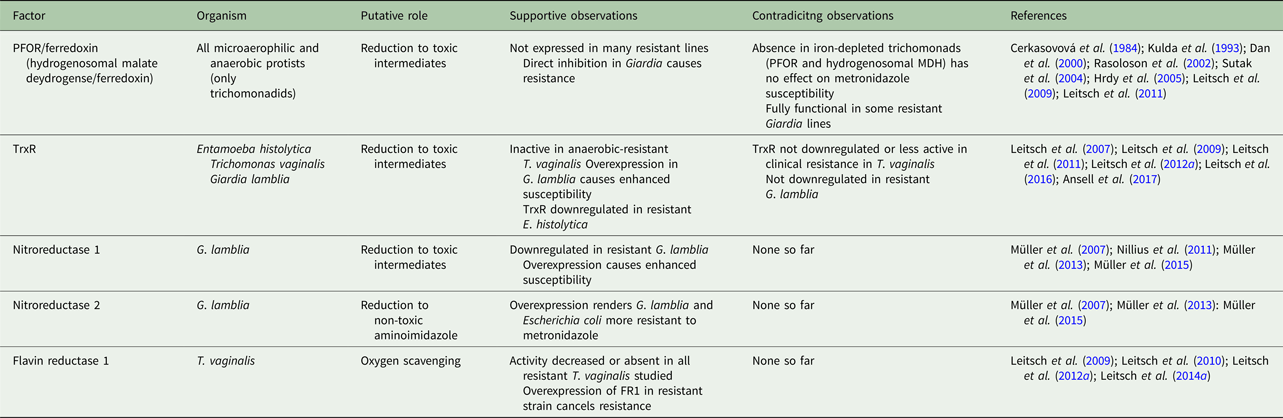
Table 3. Overview over established factors involved in metronidazole resistance in bacteria

Parasites: T. vaginalis
In T. vaginalis, the mechanisms underlying metronidazole resistance are complex. Importantly, two different types of resistance have been established in the literature: ‘aerobic’ or clinical resistance (Meingassner et al. Reference Meingassner, Mieth, Czok, Lindmark and Müller1978; Meingassner and Thurner, Reference Meingassner and Thurner1979), and ‘anaerobic’ or laboratory-induced resistance (Cerkasovová et al. Reference Cerkasovová, Cerkasov and Kulda1984; Kulda, Reference Kulda1999). The former is caused by defective oxygen scavenging mechanisms in the parasite (Yarlett et al. Reference Yarlett, Yarlett and Lloyd1986), leading to higher intracellular oxygen concentrations which counteract metronidazole activation through redox cycling (Mason and Holtzman, Reference Mason and Holtzman1975) and, consequently, increase the tolerance of T. vaginalis to the drug. Under normal growth conditions, as applied in laboratory culture, these strains exhibit no or just minimal resistance (Müller and Gorrell, Reference Müller and Gorrell1983). In the presence of oxygen, however, susceptibilities can be reduced up to several orders of magnitude. This startling effect is hard to observe in the laboratory because stably elevated oxygen levels are hard to achieve in sealed culture flasks filled with commonly used growth media developed for T. vaginalis. In the human host, however, a steady state of decreased oxygen concentrations is readily established in certain body parts. At the mucosal epithelium of the human vagina, the niche of T. vaginalis, oxygen concentrations range from 15 to 56 µ m (Ellis et al. Reference Ellis, Cole and Lloyd1992), well below the approximate 200 µ m as found in oxygen-saturated water but much higher than in growth media. It is certain, however, that this mechanism is not the only one contributing to treatment failures with metronidazole in trichomoniasis patients. Although a correlation between measurable aerobic metronidazole resistance and treatment failure does exist (Müller et al. Reference Müller, Lossick and Gorrell1988), it seems to be rather weak (Schwebke and Barrientes, Reference Schwebke and Barrientes2006), suggesting that the interplay between host and parasite has a decisive role. This interplay has not been studied as yet but likely involves a large number of factors and processes. It is interesting to speculate that one of the factors could be the oxygen concentration in the vagina which varies individually and during different phases of the menstrual cycle. It is important to note, however, that clinical resistance to metronidazole varies strongly between different parts of the world, ranging from the single-digit percentage area (Wendel and Workowski, Reference Wendel and Workowski2007) to almost 20% (Upcroft et al. Reference Upcroft, Dunn, Wal, Tabrizi, Delgadillo-Correa, Johnson, Garland, Siba and Upcroft2009), indicating that there are genetically distinct subpopulations of T. vaginalis with varying metronidazole susceptibility. This is further emphasized by the division of the species into two similarly large, globally occurring populations, of which the second comprises far more metronidazole-resistant isolates than the first (Conrad et al. Reference Conrad, Gorman, Schillinger, Fiori, Arroyo, Malla, Dubey, Gonzalez, Blank, Secor and Carlton2012).
Anaerobic metronidazole resistance can only be induced in the laboratory and has not been observed with clinical isolates, with the possible exception of one strain, i.e. B7268 (Voolmann and Boreham, Reference Voolmann and Boreham1993; Upcroft and Upcroft, Reference Upcroft and Upcroft2001). This form of resistance can be very strongly pronounced and allows growth of T. vaginalis at metronidazole concentrations up to 1000-fold higher than the minimum lethal concentration (MLC) observed with the parent cell line (Kulda et al. Reference Kulda, Tachezy and Cerkasovová1993; Leitsch et al. Reference Leitsch, Kolarich, Binder, Stadlmann, Altmann and Duchêne2009). It is, however, accompanied by fundamental changes in the parasite's physiology. Most importantly, cell lines exhibiting anaerobic resistance lack central hydrogenosomal pathways including PFOR and hydrogenase (Kulda et al. Reference Kulda, Tachezy and Cerkasovová1993; Rasoloson et al. Reference Rasoloson, Vanacova, Tomkova, Razga, Hrdy, Tachezy and Kulda2002). Consequently, they produce no hydrogen, the usual end product of the hydrogenosome. Rather, they produce lactate as the major metabolic end product, formed by cytoplasmic lactate dehydrogenases which are strongly upregulated in expression (Kulda et al. Reference Kulda, Tachezy and Cerkasovová1993). A very similar phenotype can be observed in metronidazole-resistant T. foetus (Cerkasovová et al. Reference Cerkasovová, Cerkasov and Kulda1984), a related parasite of cattle. The main fermentative end product in resistant T. foetus, however, is not lactate but ethanol. Further, highly resistant T. vaginalis cell lines have very low levels of flavins (Leitsch et al. Reference Leitsch, Kolarich, Binder, Stadlmann, Altmann and Duchêne2009), rendering flavin-dependent pathways, including TrxR, inactive. This is accompanied by a marked increase in expression of antioxidant enzymes (Leitsch et al. Reference Leitsch, Kolarich, Binder, Stadlmann, Altmann and Duchêne2009), possibly in an attempt to counterbalance the loss of TrxR activity, which is central to the antioxidant defence. Nevertheless, these cell lines are highly sensitive to oxygen and, therefore, difficult to grow. These physiological changes were interpreted as being in line with the hypothesis that PFOR and ferredoxin are critical for the activation of metronidazole because the absence of this pathway was assumed to abolish metronidazole reduction (Kulda, Reference Kulda1999). Results from several studies, however, suggest that this pathway is unlikely to be decisive for metronidazole reduction in T. vaginalis. First, the deletion of the ferredoxin 1 gene, the main interaction partner of PFOR, did not lead to a decreased susceptibility to metronidazole, although the expression of PFOR was concomitantly decreased by 95% (Land et al. Reference Land, Delgadillo-Correa, Tachezy, Vanacova, Hsieh, Sutak and Johnson2004). Further, withdrawal of intracellular iron with the iron chelator bipyridyl caused a near-to-complete shutdown of PFOR expression but did not increase tolerance to metronidazole (Leitsch et al. Reference Leitsch, Kolarich, Binder, Stadlmann, Altmann and Duchêne2009). A similar observation was made in T. foetus (Sutak et al. Reference Sutak, Dolezal, Fiumera, Hrdy, Dancis, Delgadillo-Correa, Johnson, Müller and Tachezy2004). Possibly, downregulation of PFOR and other hydrogenosomal enzymes is a consequence of low flavin levels. Evidence for this assumption is provided by a study on the effect of diphenyleneiodonium (DPI), a flavin inhibitor which covalently binds to reduced flavins, on metronidazole susceptibility in T. vaginalis (Leitsch et al. Reference Leitsch, Kolarich and Duchêne2010). Strikingly, 10 µ m of DPI rendered T. vaginalis completely insensitive to metronidazole. This was accompanied by a total loss of TrxR and PFOR activities and strongly increased expression of antioxidant enzymes, quite comparable to the situation in cell lines with induced metronidazole resistance. It is important to note, however, that protein levels of PFOR were not decreased upon addition of DPI but, to the contrary, increased, probably as an attempt by the cell to compensate for the sudden loss of PFOR activity. Unfortunately, the continued culture of T. vaginalis in the presence of DPI was not possible due to its anti-proliferative effect on the parasite, so that the long-term effect of DPI on PFOR expression could not be monitored.
Although aerobic resistance and anaerobic resistance have been established as two distinct phenomena, they have several traits in common. Most importantly, the expression of flavin reductase 1 (FR1) is decreased or even abolished in cells exhibiting either form of resistance (Ellis et al. Reference Ellis, Cole and Lloyd1992; Leitsch et al. Reference Leitsch, Drinić and Duchêne2012a). FR1 reduces oxygen to hydrogen peroxide via its FMN and NADPH cofactors and, arguably, constitutes a major pathway for oxygen scavenging in T. vaginalis (Chapman et al. Reference Chapman, Linstead and Lloyd1999; Linstead and Bradley, Reference Linstead and Bradley1988; Leitsch et al. Reference Leitsch, Janssen, Kolarich, Johnson and Duchêne2014a). Accordingly, the introduction of a functional episomal fr1 gene under the control of a strong promoter into a highly resistant clinical strain, B7268, re-established metronidazole susceptibility (Leitsch et al. Reference Leitsch, Janssen, Kolarich, Johnson and Duchêne2014a). It is, therefore, likely that FR1 is a central factor in the emergence of aerobic resistance. Since FR1 was also found to be inactive in an anaerobic-resistant cell line, it is likely that loss of this pathway is also a necessary for the development of anaerobic resistance (Leitsch et al. Reference Leitsch, Kolarich, Binder, Stadlmann, Altmann and Duchêne2009). This hypothesis is supported by the observation that an aerobic resistance-like phenotype constitutes an early intermediate stage in the development of anaerobic resistance (Tachezy et al. Reference Tachezy, Kulda and Tomková1993).
Other factors modulating metronidazole resistance in T. vaginalis also do exist, most notably nitroreductases (Pal et al. Reference Pal, Banerjee, Cui, Schwartz, Ghosh and Samuelson2009). Recently, a clear correlation of stop mutations in two nitroreductase genes, ntr4 and ntr6, and clinical resistance was found (Paulish-Miller et al. Reference Paulish-Miller, Augostini, Schuyler, Smith, Mordechai, Adelson, Gygax, Secor and Hilbert2014). However, since clinical strains do not exhibit resistance in the absence of oxygen, it is questionable if these nitroreductases directly reduce metronidazole. Their importance is, nevertheless, also suggested by a recent large-scale genomic study in which 102 isolates were included (Bradic et al. Reference Bradic, Warring, Tooley, Scheid, Secor, Land, Huang, Chen, Lee, Tang, Sullivan and Carlton2017). Ntr6, amongst other nitroreductases, was found downregulated in metronidazole-resistant strains, as was FR1. In addition, a thioredoxin family protein was upregulated, and three iron–sulphur flavoproteins, two multidrug resistance pumps, four r2r3-Myb transcription factors, and a metal ABC transporter downregulated in metronidazole-resistant T. vaginalis. These results provide good confirmation of previously made observations, but also suggest the existence of hitherto unstudied mechanisms, although it is hard to reconcile reduced drug export due to decreased levels of efflux pumps with resistance. The same study also identified a number of single-nucleotide polymorphisms associated with metronidazole resistance. Interestingly, a large number of these were found in intergenic regions, raising the possibility that they are located in sequences modulating expression of adjacent genes. This is consistent with the observation that the amino acid sequence of FR1 is unchanged even in the most resistant strains studied (Leitsch et al. Reference Leitsch, Janssen, Kolarich, Johnson and Duchêne2014a), suggesting that metronidazole resistance in T. vaginalis is not caused by mutations in genes but by their differential expression. In addition, and to make things even more complicated, different alterations might lead to the same phenotype. For example, metronidazole resistance is also strongly correlated with a decreased activity of alcohol dehydrogenase 1 (ADH1), a zinc-dependent enzyme that oxidizes secondary alcohols and reduces ketones (Leitsch et al. Reference Leitsch, Drinić and Duchêne2012a, Reference Leitsch, Williams, Lloyd and Duchêne2013). In some resistant strains ADH1 expression levels are downregulated, but in others, the decrease of ADH1 activity is caused by low intracellular zinc concentrations (Leitsch et al. Reference Leitsch, Drinić and Duchêne2012a). These issues add to the astounding complexity of metronidazole resistance in T. vaginalis and warrant further research.
Parasites: G. lamblia
Treatment regimens of giardiasis with metronidazole are failing fairly often, with varying rates being reported from different sources (Nash, Reference Nash2001; Mørch et al. Reference Mørch, Hanevik, Robertson, Strand and Langeland2008; Carter et al. Reference Carter, Nabarro, Hedley and Chiodini2017); but in contrast to clinical metronidazole resistance in T. vaginalis, no ‘aerobic’ type of resistance has been observed so far. Rather, G. lamblia isolates from patients who are refractory to metronidazole treatment are normally fully susceptible to metronidazole (Smith et al. Reference Smith, Gillin, Spira and Nash1982). This, however, might also be due to non-optimized conditions applied during drug susceptibility testing. Giardia lamblia displays a lower tolerance to oxygen as compared with T. vaginalis (Mastronicola et al. Reference Mastronicola, Giuffrè, Testa, Mura, Forte, Bordi, Pucillo, Fiori and Sarti2011), rendering metronidazole susceptibility testing in the presence of oxygen hardly feasible if its concentration is not precisely tuned (Gillin and Reiner, Reference Gillin and Reiner1982). Thus, it is presently not possible to rule out the existence of a form of clinical metronidazole resistance in G. lamblia, which resembles aerobic resistance in T. vaginalis. In fact, the results from a study in which G. lamblia isolates from refractory cases were tested in a mouse model suggest that true clinical resistance does indeed exist as the parasites also retained their tolerance to metronidazole in the mice (Lemée et al. Reference Lemée, Zaharia, Nevez, Rabodonirina, Brasseur, Ballet and Favennec2000). In any case, further endeavours are needed in the future to optimize assay conditions and to gain more clarity on whether treatment failure is caused by a resistance mechanism in the parasite or by other factors which could be, at least partly, host-derived.
Induction of metronidazole resistance in laboratory stocks of G. lamblia is easily achievable and has been reported from several laboratories. Different approaches have been applied, including prolonged culture in the presence of sublethal but increasing doses of the drug (Boreham et al. Reference Boreham, Phillips and Shepherd1988; Townson et al. Reference Townson, Laqua, Upcroft, Boreham and Upcroft1992; Müller et al. Reference Müller, Wastling, Sanderson, Müller and Hemphill2007) and mutagenesis with UV-light (Townson et al. Reference Townson, Laqua, Upcroft, Boreham and Upcroft1992). As a rule of thumb, the tolerance to metronidazole in G. lamblia can be enhanced by about 100-fold. Interestingly, strongly decreased susceptibility to metronidazole was also observed after knocking down PFOR levels with hammerhead ribozymes (Dan et al. Reference Dan, Wang and Wang2000). This contrasts with the results of similar studies performed in trichomonadids in which (very) low levels of PFOR activity did not alter metronidazole susceptibility (Land et al. Reference Land, Delgadillo-Correa, Tachezy, Vanacova, Hsieh, Sutak and Johnson2004; Sutak et al. Reference Sutak, Dolezal, Fiumera, Hrdy, Dancis, Delgadillo-Correa, Johnson, Müller and Tachezy2004; Leitsch et al. Reference Leitsch, Kolarich, Binder, Stadlmann, Altmann and Duchêne2009). Importantly, however, the knock-down of PFOR also rendered G. lamblia tolerant to oxygen (Dan et al. Reference Dan, Wang and Wang2000), indicating a large-scale shift in the parasite's physiology due to the methodology applied. Results from other studies are rather conflicting as to the role of PFOR in metronidazole resistance. In one cell line, 106-2ID10, exhibiting metronidazole resistance induced by prolonged exposure of the cells to sublethal doses of the drug, PFOR was found to be strongly downregulated (Leitsch et al. Reference Leitsch, Burgess, Dunn, Krauer, Tan, Duchêne, Upcroft, Eckmann and Upcroft2011). In a cell line with metronidazole resistance induced by mutagenesis with UV-light, however, the PFOR pathway was fully intact (Leitsch et al. Reference Leitsch, Burgess, Dunn, Krauer, Tan, Duchêne, Upcroft, Eckmann and Upcroft2011). Other factors potentially involved in metronidazole resistance were also studied, including nitroreductase 1 (NR1) (GL50803_22677, now annotated as nitroreductase Fd-NR2) which also modulates metronidazole susceptibility (Nillius et al. Reference Nillius, Müller and Müller2011). In a transfectant cell line expressing elevated levels of NR1, metronidazole susceptibility was found to be enhanced twofold to threefold. Recent data from a transcriptomic study, measuring overall mRNA expression in three resistant strains (106-2ID10, 713-M3 and WB-M3) and their respective susceptible parent strains (Ansell et al. Reference Ansell, Baker, Emery, McConville, Svärd, Gasser and Jex2017), further emphasize the role of NR1 in metronidazole resistance. In two resistant strains, expression levels were decreased and in the third line about a third of the NR1 transcripts had a non-sense mutation, effectively reducing the copy number of functional NR1 in the cell. In addition to NR1, also other nitroreductases could have a role in metronidazole resistance. Surprisingly, nitroreductase 2 (NR2) (GL50803_6175; now annotated as nitroreductase family protein fused to ferredoxin domain Fd-NR1), might have exactly the opposite, i.e. protective effect if overexpressed (Müller et al. Reference Müller, Schildknecht and Müller2013). It is possible that NR2 transfers as many as six electrons to the nitro group of metronidazole, thereby forming a non-toxic aminoimidazole. However, further research will be necessary in order to frame a reliable hypothesis regarding NR2 function. A third nitroreductase, GL50803_8377, was found to be downregulated in two of three resistant strains assayed (Ansell et al. Reference Ansell, Baker, Emery, McConville, Svärd, Gasser and Jex2017). Nitroreductase activity, however, is not necessarily only exerted by enzymes designated as nitroreductases. TrxR, for example, can reduce nitro compounds, including nitroimidazoles in several microaerophilic parasites, including G. lamblia (Leitsch et al. Reference Leitsch, Burgess, Dunn, Krauer, Tan, Duchêne, Upcroft, Eckmann and Upcroft2011). A potential role for TrxR in metronidazole activation in G. lamblia was demonstrated recently when a cell line strongly overexpressing TrxR (Leitsch et al. Reference Leitsch, Müller and Müller2016) was found to exhibit moderately increased metronidazole susceptibility. Importantly, TrxR is not downregulated in metronidazole-resistant strains (Leitsch et al. Reference Leitsch, Burgess, Dunn, Krauer, Tan, Duchêne, Upcroft, Eckmann and Upcroft2011; Ansell et al. Reference Ansell, Baker, Emery, McConville, Svärd, Gasser and Jex2017) but it is currently unclear if it is active. Loss of TrxR activity but not expression was observed before in a T. vaginalis strain with ‘anaerobic’ resistance and was caused by the loss of the enzyme's FAD cofactor (Leitsch et al. Reference Leitsch, Kolarich, Binder, Stadlmann, Altmann and Duchêne2009). Measuring TrxR activity in G. lamblia, however, is currently unfeasible because a functional thioredoxin has not yet been identified in this parasite.
In accordance with metronidazole-resistant T. vaginalis, reduction of flavins was also found to be decreased in cell extracts of metronidazole-resistant G. lamblia cell lines as compared with their parent cell lines (Ellis et al. Reference Ellis, Wingfield, Cole, Boreham and Lloyd1993; Leitsch et al. Reference Leitsch, Burgess, Dunn, Krauer, Tan, Duchêne, Upcroft, Eckmann and Upcroft2011), mirroring the observations made in T. vaginalis (Leitsch et al. Reference Leitsch, Kolarich, Binder, Stadlmann, Altmann and Duchêne2009, Reference Leitsch, Drinić and Duchêne2012a, Reference Leitsch, Janssen, Kolarich, Johnson and Duchêne2014a). A homologue of T. vaginalis FR1 does not exist in the G. lamblia genome but potential candidate enzymes which could exert this activity, three FMN-dependent oxidoreductases (GL50803_9719; GL50803_17150; GL50803_17151), were downregulated in metronidazole-resistant strains (Ansell et al. Reference Ansell, Baker, Emery, McConville, Svärd, Gasser and Jex2017). Quite confusingly, however, a closely related enzyme (GL50803_15004), termed diaphorase (Sánchez et al. Reference Sánchez, Elmendorf, Nash and Müller2001), was upregulated in two of the three strains. It was hypothesized that diaphorase exerts a different activity, i.e. detoxification of metronidazole (Ansell et al. Reference Ansell, Baker, Emery, McConville, Svärd, Gasser and Jex2017), but experimental data with the purified enzyme are needed to support this claim.
Taken together, metronidazole resistance in G. lamblia is currently not as well understood as in T. vaginalis, mainly due to the lack of clinical metronidazole-resistant strains available to the research community. There is strong evidence for an involvement of NR1, but further research on a larger number of resistant isolates is warranted.
Parasites: E. histolytica
Clinical metronidazole resistance in E. histolytica has not been reported in the field and, therefore, poses no problem for the treatment of amoebic liver abscess. Intriguingly, it is also very difficult to induce metronidazole resistance in the laboratory with only a few successful attempts documented (Samarawickrema et al. Reference Samarawickrema, Brown, Upcroft, Thammapalerd and Upcroft1997; Wassmann et al. Reference Wassmann, Hellberg, Tannich and Bruchhaus1999; Penuliar et al. Reference Penuliar, Nakada-Tsukui and Nozaki2015). Moreover, the extent of the resistance induced is far smaller than observed in T. vaginalis and G. lamblia, and ranges from twofold (Samarawickrema et al. Reference Samarawickrema, Brown, Upcroft, Thammapalerd and Upcroft1997; Penuliar et al. Reference Penuliar, Nakada-Tsukui and Nozaki2015) to about 10-fold (Wassmann et al. Reference Wassmann, Hellberg, Tannich and Bruchhaus1999) of the normal MLC. This low-level metronidazole resistance is associated with increased expression of superoxide dismutase (Samarawickrema et al. Reference Samarawickrema, Brown, Upcroft, Thammapalerd and Upcroft1997; Wassmann et al. Reference Wassmann, Hellberg, Tannich and Bruchhaus1999) and peroxiredoxin (Wassmann et al. Reference Wassmann, Hellberg, Tannich and Bruchhaus1999), and decreased expression of ferredoxin 1 and TrxR (Wassmann et al. Reference Wassmann, Hellberg, Tannich and Bruchhaus1999), which is strongly reminiscent of the changes reported for metronidazole-resistant T. vaginalis (Leitsch et al. Reference Leitsch, Kolarich, Binder, Stadlmann, Altmann and Duchêne2009). However, levels of PFOR were reported to be unchanged in another cell line with reduced susceptibility to metronidazole, whereas 88 genes in total were reported to be differentially regulated at the mRNA level (Penuliar et al. Reference Penuliar, Nakada-Tsukui and Nozaki2015). This set of genes also did not include TrxR or two NADPH-dependent oxidoreductases which had been previously discovered by the same investigators to render E. histolytica slightly more susceptible to metronidazole if overexpressed (Jeelani et al. Reference Jeelani, Husain, Sato, Ali, Suematsu, Soga and Nozaki2010). Instead, DNA polymerase, several other factors involved in DNA metabolism, and several iron–sulphur flavoproteins were upregulated, whereas several leucine-reach repeat proteins and cysteine proteases were downregulated. The significance of these observations, however, is presently unclear.
Bacteria: H. pylori
By a large margin, metronidazole resistance occurs most often in H. pylori infections, for which metronidazole is often used in combination with other antimicrobials such as clarithromycin (De Francesco et al. Reference De Francesco, Bellesia, Ridola, Manta and Zullo2017). Indeed, metronidazole resistance in H. pylori has become so widespread in some parts of the world, mainly in South Asia and Africa (De Francesco et al. Reference De Francesco, Giorgio, Hassan, Manes, Vannella, Panella, Ierardi and Zullo2010), that metronidazole has been practically rendered useless in the treatment of peptic ulcer. Resistance is, almost invariably, caused by mutations in the rdxA gene (Debets-Ossenkopp et al. Reference Debets-Ossenkopp, Pot, van Westerloo, Goddwin, Vandenbroucke-Grauls, Berg, Hoffman and Kusters1999; Jenks et al. Reference Jenks, Ferrero and Labigne1999a; Reference Jenks, Labigne and Ferrerob; Kwon et al. Reference Kwon, Hulten, Kato, Kim, Lee, El-Zaatari, Osato and Graham2001; Latham et al. Reference Latham, Labigne and Jenks2002), encoding a nitroreductase harnessing FMN and NADH as cofactors (Goodwin et al. Reference Goodwin, Kersulyte, Sisson, Veldhuyzen van Zanten, Berg and Hoffman1998; Olekhnovich et al. Reference Olekhnovich, Goodwin and Hoffman2009). In several independent studies on metronidazole-resistant clinical isolates as well as on laboratory stocks with induced resistance, the rdxA gene contained non-sense and missense mutations (Kwon et al. Reference Kwon, Hulten, Kato, Kim, Lee, El-Zaatari, Osato and Graham2001; Latham et al. Reference Latham, Labigne and Jenks2002). According to observations in some studies, metronidazole resistance can be further enhanced through mutations in the frxA gene, encoding another nitroreductase (Kwon et al. Reference Kwon, Kato, El-Zaatari, Osato and Graham2000, Reference Kwon, Hulten, Kato, Kim, Lee, El-Zaatari, Osato and Graham2001; Justino et al. Reference Justino, Parente, Boneca and Saraiva2014). This notion was further supported by a careful genomic study (Binh et al. Reference Binh, Suzuki, Trang, Kwon and Yamaoka2015) in which mutations were found in the rdxA and frxA genes in a laboratory strain with induced resistance but not its susceptible parent. Thus, at a first glance, a very clear correlation seems to exist between abolished reduction of metronidazole and resistance. At a second glance, however, the picture becomes less clear because RdxA- and FrxA-deficient clinical strains are only resistant in the presence of oxygen but not under anaerobic conditions (Gerrits et al. Reference Gerrits, van der Wouden, Bax, van Zwet, van Vliet, de Jong, Kusters, Thijs and Kuipers2004). This resembles ‘aerobic’ resistance in T. vaginalis and is incompatible with the notion that RdxA and FrxA are the only factors capable of reducing metronidazole in H. pylori. Possibly, RdxA and FrxA do not reduce metronidazole in vivo at all because metronidazole reduction by RdxA was only observed under anaerobic but not aerobic conditions in assays with the purified enzyme (Olekhnovich et al. Reference Olekhnovich, Goodwin and Hoffman2009). It is interesting to note that in strains with laboratory-induced metronidazole resistance, several enzyme activities, including disulfide reduction (possibly catalysed by a TrxR), NADH oxidation and nitroreduction were strongly decreased in metronidazole-resistant cell lines as compared with the sensitive parent cell lines (Trend et al. Reference Trend, Jorgensen, Hazell and Mendz2001). Unfortunately, these enzymes have not been further characterized but the involvement of these activities resembles metronidazole resistance in parasites (Kaakoush et al. Reference Kaakoush, Asencio, Mégraud and Mendz2009). To conclude, it is well established that clinical metronidazole resistance in H. pylori is mostly caused by mutations in the rdxA and frxA genes, at least in most cases (Marais et al. Reference Marais, Bilardi, Cantet, Mendz and Mégraud2003), but the exact mechanism of resistance remains unresolved.
Bacteria: B. fragilis and other Bacteroides spp.
Bacteroides fragilis, together with H. pylori, is the prokaryote in which metronidazole resistance has been most extensively studied. This is somewhat surprising considering resistance rates are very low (about 1%) (Urbán et al. Reference Urbán, Sóki, Brazier, Nagy and Duerden2002; Aldridge et al. Reference Aldridge, Ashcraft, O'Brien and Sanders2003; Hedberg and Nord, Reference Hedberg and Nord2003; Sóki et al. Reference Sóki, Eitel, Urbán and Nagy2013; Snydman et al. Reference Snydman, Jacobus, McDermott, Goldstein, Harrell, Jenkins, Newton, Patel and Hecht2017), although alarmingly high metronidazole resistance rates (between 5 and 10%) have been reported in the UK (Brazier et al. Reference Brazier, Stubbs and Duerden1999), Brazil (Vieira et al. Reference Vieira, Boente, Miranda, Avelar, Domingues and Ferreira2006), Lebanon (Yehya et al. Reference Yehya, Hamze, Mallat and Dabbousi2014) and Pakistan (Sheikh et al. Reference Sheikh, Jabeen, Qaiser, Ahsan, Khan and Zafar2015). Of great interest, however, is a metronidazole resistance mechanism, possibly specific for B. fragilis and still incompletely understood: Nim protein-mediated resistance. Nim proteins were discovered in 1989 as transmissible, mainly plasmid-borne metronidazole resistance determinants (Breuil et al. Reference Breuil, Dublanchet, Truffaut and Sebald1989; Haggoud et al. Reference Haggoud, Reysset, Azeddoug and Sebald1994; Sebald, Reference Sebald1994), which are normally preceded by an insertion element to enable transcription (Sóki et al. Reference Sóki, Gal, Brazier, Rotimi, Urbán, Nagy and Duerden2006). They are assumed to be the major cause of metronidazole resistance in the field and predicted to contain a FMN-binding domain and a pyridoxamine 5′-phosphate oxidase domain. Currently, nine homologues of Nim proteins have been described in Bacteroides spp. (NimA to NimJ, with NimI occurring in Prevotella, a closely related genus.) Interestingly, proteins with the same designation also exist in other organisms but the nomenclature is confusing because the different Nim homologues of Bacteroides are more closely related to each other than to homologues with the same designation in other genera, e.g. NimB in B. fragilis and Clostridium difficile. Interestingly, Nim proteins also exist in T. vaginalis and E. histolytica (Pal et al. Reference Pal, Banerjee, Cui, Schwartz, Ghosh and Samuelson2009). These homologues are only distantly related to the Nim proteins in B. fragilis but seem to have a similar function because they render Escherichia coli more insensitive to metronidazole when introduced on a plasmid (Pal et al. Reference Pal, Banerjee, Cui, Schwartz, Ghosh and Samuelson2009).
It has been proposed that Nim proteins act as nitroreductases which reduce metronidazole to non-toxic aminoimidazoles (Carlier et al. Reference Carlier, Sellier, Rager and Reysset1997) by transferring six electrons to the drug's nitro group. However, direct proof of this activity with purified Nim is lacking and data from more recent studies are hard to reconcile with this hypothesis. Expression levels of Nim proteins are not increased in nim-positive strains after the induction of high-level metronidazole resistance and, thus, are independent of the degree of metronidazole resistance (Leitsch et al. Reference Leitsch, Sóki, Kolarich, Urbán and Nagy2014b). This is at odds with the notion that Nim proteins are nitroreductases because higher concentrations of metronidazole would require larger amounts of the reducing enzyme in order to detoxify all metronidazole. Further, nim genes only confer very modest levels of resistance if transferred from highly resistant nim-positive to nim-negative recipient strains (Husain et al. Reference Husain, Veeranagouda, His, Meggersee, Abratt and Wexler2013). It is also interesting to note that the occurrence of nim genes in B. fragilis by far exceeds the proportion of metronidazole-resistant isolates (Gal and Brazier, Reference Gal and Brazier2004; Löfmark et al. Reference Löfmark, Fang, Hedberg and Edlund2005). Thus, most isolates carrying a nim gene are not metronidazole resistant. By contrast, it was repeatedly shown that high-level metronidazole resistance can be much more easily induced in nim-positive strains (Gal and Brazier, Reference Gal and Brazier2004; Löfmark et al. Reference Löfmark, Fang, Hedberg and Edlund2005; Leitsch et al. Reference Leitsch, Sóki, Kolarich, Urbán and Nagy2014b) than in nim-negative strains, although the latter is still possible (Schaumann et al. Reference Schaumann, Petzold and Rodloff2005). It is, therefore, certain that Nim proteins are correlated with metronidazole resistance but the underlying mechanism remains to be discovered.
In addition to Nim proteins, other factors potentially involved in metronidazole resistance were studied. Importantly, a knock-out of PFOR (Diniz et al. Reference Diniz, Farias, Carvalho, Rocha and Smith2004) had little or no effect at all on metronidazole susceptibility. However, it was demonstrated that the deletion of the iron transporter gene feoAB leads to reduced susceptibility of B. fragilis to metronidazole (Veeranagouda et al. Reference Veeranagouda, Husain, Boente, Moore, Smith, Rocha, Patrick and Wexler2014), but it is presently unclear if the iron import is also reduced in metronidazole-resistant B. fragilis clinical isolates. A forced efflux of metronidazole through efflux pumps of the RND family (Pumbwe et al. Reference Pumbwe, Glass and Wexler2006, Reference Pumbwe, Chang, Smith and Wexler2007) could also have a certain role in metronidazole resistance although their role in clinical metronidazole resistance remains to be established.
Bacteria: Clostridia
Fairly little is known about metronidazole resistance in clostridia despite their great medical importance. Metronidazole, together with vancomycin, has remained the treatment option of choice for C. difficile infections (Peng et al. Reference Peng, Jin, Kim, Stratton, Wu, Tang and Sun2017) but treatment failures seem to occur more frequently lately (Leffler and Lamont, Reference Leffler and Lamont2015). It is important, however, to emphasize that treatment failures are not necessarily caused by resistance as such, as discussed previously. Nevertheless, some of the refractory strains are definitely metronidazole-resistant, as determined in appropriate susceptibility assays. In a careful proteomic study (Chong et al. Reference Chong, Lynch, McCorrister, Kibsey, Miller, Gravel, Westmacott and Mulvey2014), overall protein expression in one such isolate was compared to a normally metronidazole susceptible isolate, revealing numerous changes in the expression profile. Interestingly, several thioredoxin reductases and thioredoxins were differentially expressed and ferredoxin was downregulated approximately 2·5-fold. In contrast, a Nim homologue, NimB, was expressed more strongly (upto threefold). The significance of these changes remains unclear, but the same candidate factors emerge in C. difficile with respect to metronidazole resistance as seen in other microbes. Metronidazole resistance in clostridia can also be induced in the laboratory. In a study on Clostridium perfringens, metronidazole resistance was induced by mutagenesis using N-methyl-N′-nitro-N-nitrosoguanidine lactate (Sindar et al. Reference Sindar, Britz and Wilkinson1982). Quite in accordance with the observations in T. vaginalis, resistance was accompanied by a total loss of PFOR activity and a shift of metabolic end products from acetate to pyruvate and lactate.
Other 5-nitroimidazoles and outlook
Research on alternative 5-nitroimidazoles began soon after the introduction of metronidazole in order to develop alternatives with similar potential but improved characteristics such as patient compliance, serum half-life and safety. Tinidazole (Fig. 2) has emerged as the most successful of these alternative 5-nitroimidazoles and is superior to metronidazole in several aspects. It has the same spectrum as metronidazole (Fung and Doan, Reference Fung and Doan2005) but a longer half-life, i.e. 12·5 vs 7·3 h (Wood and Monro, Reference Wood and Monro1975), and is better tolerated (Fung and Doan, Reference Fung and Doan2005). Most importantly, tinidazole can be used to overcome metronidazole resistance in many cases. In metronidazole refractory trichomoniasis patients, for example, cure rates with tinidazole were as high as 92% (Sobel et al. Reference Sobel, Nyiresy and Brown2001). Despite these advantages, tinidazole was not approved in the USA before 2004 (Nailor and Sobel, Reference Nailor and Sobel2007), and in many countries metronidazole has even yet remained the only approved 5-nitroimidazole for the treatment of anaerobic infections in man. Nevertheless, other 5-nitroimidazoles are in use, such as ornidazole, nimorazole, ronidazole and dimetridazole. Ronidazole and dimetridazole were originally widely used in food-producing animals but were banned in the USA and the EU due to their suspected carcinogenic potential. The use of 5-nitroimidazoles, however, is still legal for the treatment of anaerobic infections in companion animals, such as ronidazole for the treatment of trichomoniasis in cats (Gookin et al. Reference Gookin, Hanrahan and Levy2017). The mode of action of the various 5-nitroimidazoles seems to be very similar. Along with DNA (Zahoor et al. Reference Zahoor, Lafleur, Knight, Loman and Edwards1987), proteins and thiols seem to be affected by all 5-nitroimidazoles studied so far. Tinidazole, for example, was found to bind the same proteins as metronidazole in the parasites E. histolytica (Leitsch et al. Reference Leitsch, Kolarich, Wilson, Altmann and Duchêne2007), T. vaginalis (Leitsch et al. Reference Leitsch, Kolarich, Binder, Stadlmann, Altmann and Duchêne2009) and G. lamblia (Leitsch et al. Reference Leitsch, Schlosser, Burgess and Duchêne2012b) and to inhibit TrxR to a similar extent as metronidazole (Leitsch et al. Reference Leitsch, Kolarich, Wilson, Altmann and Duchêne2007, Reference Leitsch, Kolarich, Binder, Stadlmann, Altmann and Duchêne2009). Moreover, tinidazole, ornidazole and ronidazole also decrease non-protein thiol levels, with ronidazole exhibiting the strongest effect (Leitsch et al. Reference Leitsch, Kolarich, Wilson, Altmann and Duchêne2007, Reference Leitsch, Kolarich, Binder, Stadlmann, Altmann and Duchêne2009, Reference Leitsch, Schlosser, Burgess and Duchêne2012b).
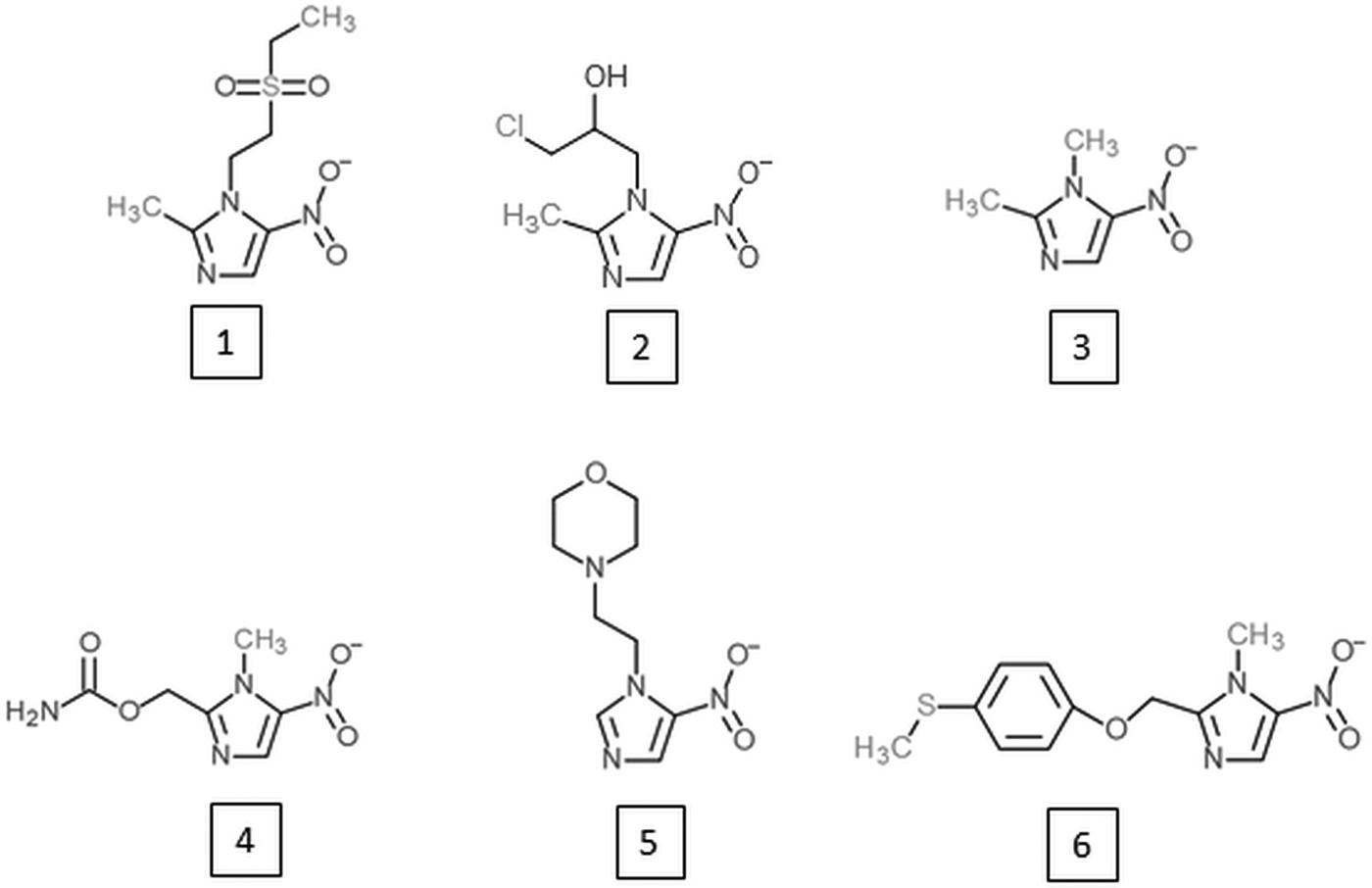
Fig. 2. 5-nitroimidazoles developed as alternatives to metronidazole or as novel treatment option against African trypanosomiasis. (1) Tinidazole; (2) ornidazole; (3) dimetridazole; (4) ronidazole; (5) nimorazole; (6) fexinidazole.
Despite the reluctance of the authorities to approve alternative 5-nitroimidazoles, obviously due to the deficient safety profile of this drug class, research on novel 5-nitroimidazoles has never stopped. There are many promising candidates amongst newly developed 5-nitroimidazoles which could enable more effective treatments with reduced mutagenicity and an improved management of metronidazole resistance in the future (Crozet et al. Reference Crozet, Botta, Gasquet, Curti, Rémusat, Hutter, Chapelle, Azas, De Méo and Vanelle2009; Dunn et al. Reference Dunn, Burgess, Krauer, Eckmann, Vanelle, Crozet, Gillin, Upcroft and Upcroft2010; Jarrad et al. Reference Jarrad, Debnath, Miyamoto, Hansford, Pelingon, Butler, Bains, Karoli, Blaskovich, Eckmann and Cooper2016). Interestingly, another 5-nitroimidazole which was developed in 1983, fexinidazole (Jennings and Urquhart, Reference Jennings and Urquhart1983; Raether and Seidenath, Reference Raether and Seidenath1983), might revolutionize the notoriously difficult treatment of African trypanosomiasis or sleeping sickness in the near future (https://www.dndi.org/diseases-projects/portfolio/fexinidazole/). Probably, fexinidazole has a different mode of action than other 5-nitroimidazoles because trypanosomatids are not microaerophilic. This example shows that the well-studied drug class of 5-nitroimidazoles might still have some surprises in store for us.
Acknowledgements
The author thanks Norbert Müller, Joachim Müller and Michael Duchêne for careful reading of the manuscript.
Financial Support
This research received no specific grant from any funding agency, commercial or not-for-profit sectors.