Introduction
The ‘sunshine hormone’, vitamin D, is a pro-steroid hormone that is reported to be the earliest hormone to arise on earth(Reference Bikle1). In the last 20 years, the number of scientific studies reporting the importance of vitamin D dietary intake and supplementation for cell and tissue function has increased dramatically. However, clinical studies have indicated that more than 1 billion individuals present with vitamin D insufficiency or deficiency(Reference Wacker and Holick2–Reference Daly, Gagnon and Lu5). Vitamin D receptor (VDR) expression in human muscle declines with age and a reduced capacity for UV-mediated vitamin D synthesis in the elderly’s skin may partly explain why the muscles of these individuals tend to be more susceptible to low vitamin D levels(Reference Girgis, Clifton-Bligh and Turner6). Hence, low vitamin D status has been considered a worldwide public health problem and is associated with the development of many diseases, such as osteoporosis, cancer, infertility, type 2 diabetes mellitus, coronary artery disease, and also has a significant impact on the immune system(Reference Chowdhury, Kunutsor and Vitezova7–Reference Calton, Keane and Newsholme10).
It has long been accepted that vitamin D plays a critical role in the regulation of Ca2+ and phosphate homeostasis and therefore is critical for impact on bone function. However, the discovery of a VDR in skeletal muscle cells provided further evidence of the important role of this hormone in skeletal muscle function and metabolism(Reference Hassan-Smith, Jenkinson and Smith11–Reference Pojednic, Ceglia and Olsson14). Low levels of vitamin D are associated with skeletal muscle fibre atrophy, muscle pain, weakness, and increased risk of sarcopenia and associated falls, in active and non-active individuals(Reference Pfeifer, Begerow and Minne15–Reference Lappe, Cullen and Haynatzki18). In athletes, low vitamin D levels are associated with poor bone health, and can impair muscle and immune functions, resulting in low muscle regenerative capacity after exercise sessions(Reference Dahlquist, Dieter and Koehle19) and high risk of upper respiratory tract infections(Reference Bergman, Lindh and Bjorkhem-Bergman20), respectively. The importance of vitamin D for skeletal muscle function and ultimately for whole body health is also supported by several randomised controlled trials (RCT) where vitamin D supplementation resulted in an increase in muscle strength in physically active and non-active individuals(Reference Chiang, Ismaeel and Griffis21,Reference Salminen, Saaristo and Salonoja22) .
Several molecular mechanisms have been proposed to mediate the effects of vitamin D in muscle strength, function and metabolism, including changes in protein synthesis, myogenesis, mitochondrial activity, muscle regeneration and glucose metabolism(Reference Salminen, Saaristo and Salonoja22–Reference Owens, Sharples and Polydorou25). However, the exact underlying mechanisms of vitamin D-related pathways, as well as their regulation and action in skeletal muscle, or how these pathways can be translated into clinical improvements, are unclear. Hence, the main purpose of the present review is to discuss the evidence for vitamin D action in skeletal muscle function and metabolism, at the molecular level, citing results from studies in vitro, in vivo and clinical research.
Vitamin D physiology and synthesis
Vitamin D is a liposoluble pro-hormone mostly found in two forms (vitamin D2 and vitamin D3) and is obtained by a self-regulated process, as described below. Sources of dietary vitamin D include oily fish, eggs and dairy products; however, the consumption and intestinal absorption of vitamin D represent only a minor part of the total vitamin D requirement for the whole body(Reference Pfeifer, Begerow and Minne15). Exposure of the skin to UV radiation (wavelength 290–315 mm) is associated with 80–90 % of total vitamin D synthesis and allows 7-dehydrocholesterol to be converted to cholecalciferol (pre-vitamin D3)(Reference Pfeifer, Begerow and Minne15,Reference Calton, Pathak and Soares26,Reference Chen, Chimeh and Lu27) . Both endogenous synthesis and exogenous dietary intake raise serum concentrations of vitamin D, which undergoes two hydroxylation steps to the final product(Reference Armas, Hollis and Heaney28). Firstly, pre-vitamin D3 is thermal isomerised to vitamin D3, binds to vitamin D binding protein and is subsequently transported into the liver where it is hydroxylated by the enzyme vitamin D 25-hydroxylase to 25-hydroxyvitamin D (25(OH)D). This molecule is the predominant circulating form of vitamin D that is traditionally measured to identify vitamin D status in humans as it has a relatively long half-life in the circulation as it is established as a reliable marker of vitamin D status(Reference Heaney and Holick29). 25(OH)D is then metabolised primarily in the proximal tubule of the kidney, or other tissues such as the skeletal muscle, by the enzyme 25-hydroxyvitamin D-1α-hydroxylase (encoded by the CYP27B1 mitochondrial gene) to its biologically active form 1,25-hydroxyvitamin D3 (1,25(OH)2D3), also known as calcitriol(Reference Chen, Chimeh and Lu27,Reference Girgis, Clifton-Bligh and Hamrick30) . Subsequently, the resultant seco-steroid is carried by vitamin D binding protein via plasma from the kidneys to target tissues where it is able to bind to the potent transcription factor VDR. Receptor binding leads to conformational changes within the receptor that allow the VDR to interact with its heterodimeric partner, the retinoid X receptor, in the nucleus. VDR also forms homodimers that bind specific regions of DNA and activate or inhibit gene transcription(Reference Norman, Nemere and Zhou31). The biologically active form 1,25(OH)2D3 is responsible for maintaining Ca2+ and PO42– homeostasis(Reference Heaney and Holick29). Interestingly, it has been proposed that vitamin D biological activity involves unbound or free fractions of the vitamin(Reference Chun, Peercy and Orwoll32,Reference Tsuprykov, Chen and Hocher33) . The ‘free-hormone hypothesis’ is considered a different pathway for cellular uptake of steroid hormones, as these molecules are highly lipophilic and therefore have the potential to quickly and passively diffuse across cell membranes(Reference Chun, Peercy and Orwoll32). It seems to be important to measure and differentiate total vitamin D (measured as 25(OH)D) and bioavailable vitamin D (i.e. free vitamin D) as important evidence suggests that vitamin D binding protein inhibits certain actions of vitamin D since the bound fraction is unavailable to act on target cells(Reference Powe, Evans and Wenger34,Reference Bikle, Gee and Halloran35) . Powe et al. (Reference Powe, Ricciardi and Berg36) have demonstrated in healthy adults that bone mineral density was more strongly associated with the bioavailable fraction of circulating 25(OH)D than the total levels(Reference Powe, Ricciardi and Berg36). In accordance with this result, other studies suggest that free vitamin D is a better predictor of bone mineral density especially in an ethnically diverse athletic population and also correlates better with parathyroid hormone (the marker of Ca balance associated with bone health) than total serum 25(OH)D concentration(Reference Shieh, Chun and Ma37,Reference Allison, Farooq and Cherif38) .
Role of vitamin D in skeletal muscle
Proliferation and differentiation
The development of muscular tissue (myogenesis) has several phases starting from stem cells located in somites, followed by the development of the first progenitor cells named myoblasts, and finally their differentiation into mature myotubes(Reference Tajbakhsh39). Regulation of myogenesis depends mainly on two transcription factor systems: paired-box transcription factors, Pax3 and Pax7, and a family of basic Helix–Loop–Helix transcription factors known as myogenic regulatory factors (MRF)(Reference Sambasivan and Tajbakhsh40). Myoblasts then proliferate extensively until they reach a myofibrillar protein synthesis peak and then differentiate into mature myotubes, due to activation of MRF. To maintain tissue homeostasis, there is a subpopulation of cells that resides in the quiescent state, also known as satellite cells. These cells also have the potential to differentiate into new muscle fibres (i.e. myogenesis) and maintain protein turnover(Reference Braun and Gautel41,Reference Bentzinger, Wang and Rudnicki42) . Briefly, the fate and differentiation of muscle cells are controlled by four MRF: myogenic factor 5 (Myf5), muscle-specific regulatory factor 4 (MRF4), myoblast determination protein (MyoD) and myogenin (MYOG). Myf5 and MyoD are specifically degraded at mitosis and G1/S, respectively, and this is mediated by phosphorylation via cyclin-dependent kinases. Myf5 and MyoD determine skeletal muscle cell identity, and are consequently considered to be the gatekeepers for entry into the terminal specification of myogenic lineage(Reference Pownall, Gustafsson and Emerson43), whereas MYOG is essential for the differentiation of myoblasts into myotubes(Reference Berkes and Tapscott44). MYOG acts genetically downstream of MyoD and Myf5 to switch on muscle differentiation genes. Although MRF4 is classified as a differentiation gene, it is also believed to act as a determinant gene when it is expressed by undifferentiated cells(Reference Valdez, Richardson and Klein45). MRF signalling molecules induce the activation of skeletal muscle cell receptors and regulate transcription of specific target genes in order to develop the adult skeletal muscle tissue, such as SIX1–SIX6 and myocyte enhancer factor (MEF)2 proteins (MEF2A, MEF2C and MEF2D)(Reference Bentzinger, Wang and Rudnicki42).
Several animal studies have demonstrated that a vitamin D-deficient diet decreases markers of proliferation, such as bone morphogenetic protein family, fibroblast growth factor 2 and/or proliferating cell nuclear antigen, while increasing markers of myogenic differentiation such as Myf5, myostatin, atrophy marker E3-ubiqutin ligases and muscle ring-finger protein-1 (Table 1)(Reference Domingues-Faria, Chanet and Salles46). Interestingly, in many studies the expression of VDR in skeletal muscle increases in animals receiving a vitamin D-sufficient diet and/or vitamin D-rich diet, which suggests that the effects of vitamin D are dependent on its receptor(Reference Hutton, Vaughn and Litta47,Reference Girgis, Clifton-Bligh and Mokbel48) . VDR was also detected in the nucleus of muscle fibres from middle-aged and older female patients with osteoarthritis/osteoporosis and the expression seems to decrease with age(Reference Bischoff, Borchers and Gudat49,Reference Bischoff-Ferrari, Borchers and Gudat50) . In accordance with these studies, Ceglia et al. (Reference Ceglia, da Silva Morais and Park51) also confirmed the VDR expression in skeletal muscles from healthy postmenopausal women. Conversely, a recent study has reported that VDR protein is readily detected in human myoblasts and myotubes, while non-detectable in adult human skeletal muscle tissue(Reference Olsson, Saini and Stromberg23). It is important to highlight that the detection of VDR expressed in skeletal muscle cells and tissue varies according with the technique used for protein extraction and the type of primary antibody; consequently many authors have found difficulty in detecting this receptor(Reference Girgis, Mokbel and Cha52).
Table 1. Overview of the biomolecular role of vitamin D (VitD) in skeletal muscle (six animal studies)

↑, Increase; ↓, decrease; 25(OH)D, 25-hydroxyvitamin D; AIN, American Institution of Nutrition; BMP4, bone morphogenetic protein family; FGF2, fibroblast growth factor 2; IU, international units; MyoD, family of myogenic regulatory factors; MuRF1, muscle ring-finger protein-1; VDR-KO, vitamin D receptor knockout mouse; PCNA, proliferating cell nuclear antigen; VDR, vitamin D receptor.
The majority of animal studies have focused on the physiological effects of vitamin D on muscle mass and strength(Reference Oku, Tanabe and Nakaoka53–Reference Girgis, Cha and Houweling55) and most have not investigated the precise pathways regulating the reported outcomes. At the cellular level, some studies have investigated the effects of vitamin D in murine cell lines, such as C2C12 (Table 2), and demonstrated that treatment with vitamin D inhibits cell proliferation(Reference Olsson, Saini and Stromberg23,Reference Girgis, Cha and Houweling55–Reference Van der Meijden, Bravenboer and Dirks59) and stimulates cell differentiation(Reference Owens, Sharples and Polydorou25,Reference Garcia, Ferrini and Norris58,Reference Irazoqui, Boland and Buitrago60,Reference Braga, Simmons and Norris61) . More recently, Braga et al. (Reference Braga, Simmons and Norris61) have shown that treatment with 1,25(OH)2D3 in primary cultures of satellite cells enhances myogenic differentiation through an increase in the expression of myogenic markers, such as MyoD and MYOG, myotube formation, and the modulation of pro- and anti-myogenic factors(Reference Braga, Simmons and Norris61). These results are in agreement with previous studies that identified an increase in myogenic factors such as MYOG, Myf5 and MYC2 (transcription factor) in response to vitamin D treatment(Reference Girgis, Clifton-Bligh and Mokbel48,Reference Van der Meijden, Bravenboer and Dirks59,Reference Irazoqui, Boland and Buitrago60) . In addition, Ryan et al. (Reference Ryan, Daniel and Craggs62) were the first group to prove a dose–response effect of the active form of vitamin D to modulate the capacity of C2C12 cells to transdifferentiate into adipocytes. Low concentrations of vitamin D (simulating a deficient status) induced adipogenesis and up-regulation of key adipogenic marker genes (PPARγ2 and fatty acid binding protein 4 (FABP4)), whereas higher concentrations attenuated the differentiation into adipocytes. Consequently, an increase in triacylglycerol synthesis and levels within skeletal muscle is associated with a decrease in functional strength and impairment of glucose tolerance, leading to a higher risk of developing metabolic diseases, insulin resistance, obesity and type 2 diabetes(Reference Goodpaster, Krishnaswami and Resnick63–Reference Zoico, Rossi and Di Francesco65). However, in primary human skeletal muscle cells, the effects of vitamin D on proliferation and differentiation are conflicting(Reference Olsson, Saini and Stromberg23,Reference Owens, Sharples and Polydorou25) . Owens et al. (Reference Owens, Sharples and Polydorou25) have investigated the effects of vitamin D during differentiation in human primary muscle cells collected by biopsies from active adults and found an increase in myotube fusion and differentiation(Reference Owens, Sharples and Polydorou25). On the other hand, Olsson et al.(Reference Olsson, Saini and Stromberg23) reported opposite effects regarding differentiation, such as reduction of expression of cell cycle regulators and myogenic regulatory factors (MyoD, MYOG, MEF2C and sarcomeric proteins), with associated activation in forkhead box O3 and Notch signalling pathways(Reference Olsson, Saini and Stromberg23).
Table 2. Overview of the biomolecular role of vitamin D (VitD) in skeletal muscle cells (eleven in vitro studies)
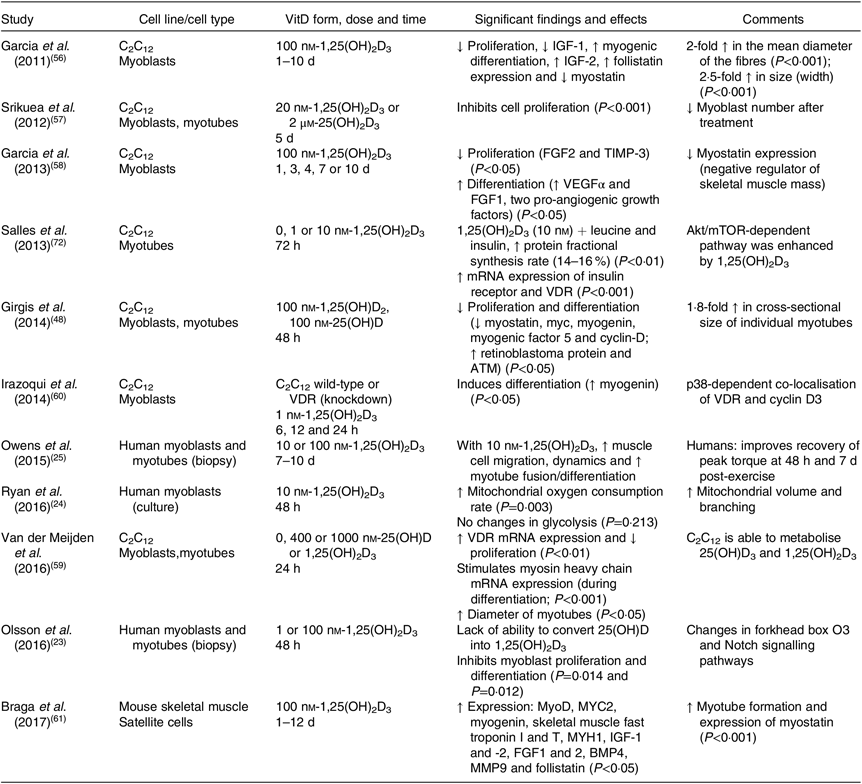
↑, Increase; ↓, decrease; 1,25(OH)2D3, 1,25-hydroxyvitamin D3; 25(OH)D, 25-hydroxyvitamin D; Akt, protein kinase B; ATM, ataxia telangiectasia mutated; BMP4, bone morphogenetic protein family; FGF, fibroblast growth factor; IGF, insulin-like growth factor; MMP9, matrix metallopeptidase 9; mTOR, mammalian target of rapamycin; MYC2, transcription factor; MYH1, heavy polypeptide 1; MyoD, family of myogenic regulatory factors; TIMP-3, metalloproteinase inhibitor 3; VDR, vitamin D receptor; VEGFα, vascular endothelial growth factor.
An important factor to consider regarding skeletal muscle cell lines is that the conversion of 25(OH)D to its active form (1,25(OH)2D3) has not been confirmed to occur locally in primary skeletal muscle cells, whereas it does occur in the skeletal muscle murine cell line C2C12 and in vivo in mice(Reference Olsson, Saini and Stromberg23,Reference Van der Meijden, Bravenboer and Dirks59) . In addition, discrepancies exist among studies regarding the cell line used, and vitamin D form, dose and duration of the treatment, consequently leading to different outcomes. Overall, vitamin D influences myogenesis through regulation of myogenic factors and proteins involved in this process. The main findings suggest that vitamin D promotes differentiation while reducing proliferation in murine cells; however, is still difficult to define how vitamin D affects proliferation and differentiation in primary human muscle cells. Further studies are needed to elucidate the cellular development stages and/or subpopulations of cells that undergo regulation by vitamin D and thus affect the process of muscular development.
Protein synthesis and myotube size
Cellular protein content is controlled by anabolic and catabolic mechanisms that regulate synthesis and degradation of muscle proteins, resulting in changes in muscle mass(Reference Braun and Gautel41). The main mechanisms that regulate protein synthesis in skeletal muscle are via the insulin signalling cascade, insulin-like growth factors (IGF, such as IGF-1 and IGF-2) and amino acids(Reference Matsakas and Patel66). These molecules, upon receptor binding, induce phosphorylation and activation of sequential targets, including the insulin receptor substrate, phosphatidylinositol-3 kinase (PI3K), phosphoinositide-dependent kinase-1, protein kinase B serine/threonine kinase family (Akt/PKB), mammalian target of rapamycin (mTOR) and 70-kDa S6 protein kinase (p70S6k)(Reference Trendelenburg, Meyer and Rohner67). mTOR is the major regulator of cell growth and proliferation by controlling the initiation phase of protein translation and synthesis (Fig. 1)(Reference Hay and Sonenberg68). Furthermore, it has been suggested that regulatory proteins, for example transforming growth factor β (TGF-β) family and myostatin, also play an essential role in protein synthesis and myotube size by regulating Akt/PKB, mitogen-activated protein kinase (MAPK) and mTOR pathways(Reference Winbanks, Weeks and Thomson69,Reference Artaza and Norris70) . The proposed model of signalling is via activation of the TGF-β signalling pathway that initially is inhibited by follistatin (activin-binding protein essential for muscle fibre formation and growth)(Reference Winbanks, Weeks and Thomson69,Reference Artaza and Norris70) . Follistatin subsequently binds to multiple extracellular ligands including myostatin which modifies the transcription of Smad target genes, consequently stimulating Akt/mTOR signalling that potentiates protein synthesis(Reference Winbanks, Weeks and Thomson69).

Fig. 1. Proposed mechanisms of action of vitamin D (VitD) in mammalian skeletal muscle cells. 4E-BP1, eukaryotic translation initiation factor 4E-binding protein 1; AKT, serine–threonine kinase; Ca2+, calcium ions; CREB, cellular transcription factor; c-Src, proto-oncogene c-Src; DAG, diacylglycerol; ELK1, ETS domain-containing protein; ERK1/2, extracellular signal-regulated kinases; HSP27, heat shock protein 27; IP3, inositol triphosphate; MAPK, mitogen-activated protein kinase; mTOR, mammalian target of rapamycin; OCR, oxygen consumption rate; P38, P38 mitogen-activated protein kinases; P70S6K, ribosomal protein S6 kinase β-1; PI3K, phosphoinositide-3 kinase; PIP2, phosphatidylinositol biphosphate; PKC, protein kinase C; PLCγ, phospholipase Cγ; Raf-1, proto-oncogene serine/threonine-protein kinase (also known as c-RAF); RNA poly, RNA polymerase; RXR, retinoid X receptor; SOCE, store-operated calcium entry; SR, sarcoplasmic reticulum; VDCC, L-type voltage-dependent calcium channel; VDR, vitamin D receptor; VDRE, vitamin D response elements.
The first in vivo study to suggest an association between vitamin D and improvements in net muscle protein synthesis was published in 1975(Reference Birge and Haddad71). This first observation indicated that 25(OH)D supplementation increased [3H]leucine incorporation into proteins in rat skeletal muscle and specified that the vitamin D effect was, at least in part, independent of the action of the intestinal transport of Ca and phosphate(Reference Birge and Haddad71). However, Ca2+ and phosphate (PO42–) could be directly involved in the mechanism of action of vitamin D in muscle cells. Subsequent studies with VDR knockout (VDR-KO) mice, and with also mice subjected to a vitamin D-deficient diet (Table 1), have reported significant muscular atrophy, weakened strength, decreased muscle fibre size, lower bone mineral density and dysregulation of myogenic regulatory factors when compared with control groups, i.e. normal mice and mice receiving a vitamin D-sufficient diet(Reference Domingues-Faria, Chanet and Salles46,Reference Oku, Tanabe and Nakaoka53–Reference Girgis, Cha and Houweling55) . Moreover, Oku et al. (Reference Oku, Tanabe and Nakaoka53) have demonstrated the negative impact of vitamin D deficiency on the levels of mRNA expression of MyoD in skeletal muscle tissue, which corroborates the importance of vitamin D for the process of myogenesis, muscle maintenance and hypertrophy. Salles et al.(Reference Salles, Chanet and Giraudet72) later confirmed that an in vitro treatment with 10 nm-1,25(OH)2D3 potentiated the effects of leucine and insulin; and increased the protein synthesis rate by 14–16 % through Akt/PKB and mTOR pathways. In the later study vitamin D has also been shown to enhance the phosphorylation of Akt/PKB and glycogen synthase kinase 3β (GSK3β), and consequently improve insulin signalling by up-regulating the insulin receptor. It is proposed that Akt inhibits GSK3 and, as a consequence, activates the eukaryotic translation initiation factor 2B (eIF2B), resulting in the formation of the 43S preinitiation complex. Furthermore, mTOR phosphorylates eukaryotic translation initiation factor 4E-binding protein 1 (4E-BP1) and p70S6K allowing assembly of the 43S pre-initiation complex(Reference Bolster, Jefferson and Kimball73,Reference Proud and Denton74) . Further research is important to identify if vitamin D has a biological effect on amino acid transporters, as a potential alternative pathway to improve protein synthesis and myotube size. Studies also have reported the action of vitamin D on muscle proteolytic activity and regulation via proteases, specifically the ubiquitin–proteasome pathway(Reference Bhat, Kalam and Qadri75,Reference Mitch and Goldberg76) . In a rat muscle model, vitamin D deficiency led to an increase in activities of the glutathione-dependent enzymes and decrease in superoxide dismutase and catalase enzymes, resulting in oxidative stress and proteolysis. Rehabilitation with vitamin D could reverse the alterations in oxidative stress parameters, increase total protein degradation and muscle atrophy gene markers post-vitamin D treatment in C2C12 muscle cells(Reference Bhat and Ismail77). The same authors have also demonstrated an increase in protein degradation and decreased protein synthesis after a vitamin D-deficient diet in male rats(Reference Bhat, Kalam and Qadri75).
Some of the strongest evidence linking vitamin D to protein synthesis is the stimulation of fibre hypertrophy(Reference Bentzinger, Wang and Rudnicki42). In one study, fibre hypertrophy was observed after 10 d of treatment with 1,25(OH)2D3, with a significant 2-fold increase in the mean diameter of C2C12 fibres, and an increase of 2·5-fold in length(Reference Garcia, King and Ferrini56). These results were attributed to an increase in protein synthesis; however, the authors did not measure this parameter directly. Similar results were obtained by van der Meijden et al. (Reference Van der Meijden, Bravenboer and Dirks59) where the treatment with 25(OH)D for 3 d resulted in a 19 % increase in C2C12 fibre diameter. Despite previous evidence of the impact of vitamin D on metabolic signalling pathways and phenotype in skeletal muscle cell lines, there is currently no evidence regarding the effect of vitamin D on protein synthesis in primary human skeletal muscle cells. Finally, in clinical studies, vitamin D supplementation in healthy human subjects with low serum levels activates the VDR in skeletal muscle tissue, which appears to stimulate protein synthesis and improve muscle strength following an increase in the size and number of type II muscle fibres(Reference Daly, Gagnon and Lu5,Reference Bruyere, Cavalier and Souberbielle78) .
Mitochondrial metabolism
Adequate mitochondrial function is essential for cellular homeostasis, especially in high energy-demanding skeletal muscle cells, as mitochondria are the main organelles responsible for generating energy in the form of ATP(Reference Deepa, Bhaskaran and Ranjit79). Typically, the effects of vitamin D are intermediated by its interaction with a nuclear VDR and is part of the nuclear receptor superfamily of ligand-activated transcription factors. VDR can also be translocated into the mitochondria of certain cell types, including the skeletal muscle cells, and potentially act directly on cellular bioenergetics(Reference Girgis, Clifton-Bligh and Hamrick30). This evidence led researchers to consider that mitochondrial activity could potentially be modified by a diet containing sufficient levels of vitamin D and/or supplementation, which is thought to increase cellular metabolism. Interestingly, a few studies have indicated that vitamin D supplementation modulates mitochondrial activity and enhances ATP production at rest and during exercise(Reference Sinha, Hollingsworth and Ball80,Reference Rana, Marwaha and Kumar81) . More recently, a positive association between vitamin D and RMR was observed in obese adults(Reference Calton, Pathak and Soares26). The latter study demonstrated that for every 10 nmol/l increase in serum 25(OH)D, the RMR increased by 56·5 kJ/d(Reference Campbell and Allain16). However, another study with a very short (1 week) period of vitamin D supplementation reported no influence on energy or substrate utilisation(Reference Boon, Hul and Sicard82). Additional randomised clinical trials examining the influence of vitamin D on energy expenditure are therefore urgently needed.
The mechanism of vitamin D action in mitochondrial metabolism currently proposed is an increase in the expression of electron transport chain proteins and the tricarboxylic acid cycle enzymes via both genomic and non-genomic pathways(Reference Silvagno and Pescarmona83). This theory was tested by Muñoz Garcia et al.(Reference Muñoz Garcia, Kutmon and Eijssen84) who investigated pathways related to the tricarboxylic acid cycle, oxidative phosphorylation and ATP synthesis in existing gene expression databases from multiple related monocyte models(Reference Muñoz Garcia, Kutmon and Eijssen84). In this study, genes associated with the electron transport chain activity and in the conversion of acetyl-CoA to CO2 were up-regulated by vitamin D in three immune cell types, the THP-1 monocytic cell line, monocyte derived dendritic cells and monocytes(Reference Muñoz Garcia, Kutmon and Eijssen84). The same pattern was observed in human peripheral blood mononuclear cells linking serum vitamin D status with specific markers of bioenergetic pathways(Reference Calton, Keane and Soares85).
With respect to skeletal muscle tissue, the precise function of vitamin D in relation to mitochondrial metabolism remains highly elusive. Ryan et al. (Reference Ryan, Craig and Folmes24) has investigated the effects of vitamin D on cellular bioenergetics in primary human muscle cells (undifferentiated myoblasts). The authors have demonstrated that mitochondrial oxygen consumption rate increased when cells were treated with vitamin D for 48 h and this response was dependent of the VDR(Reference Ryan, Craig and Folmes24). A possible mechanism for this, is an elevation in mitochondrial volume fraction and branching, which may result in mitochondrial fusion and biogenesis. Vitamin D treatment increased expression levels of MYC, MAPK13 and endothelial PAS domain-containing protein 1 mRNA (which encodes for a protein that regulates mitochondrial biogenesis)(Reference Ryan, Craig and Folmes24). In addition, mediators of mitochondrial fusion were altered. Specifically, OPA1 expression increased following vitamin D treatment, while mediators of mitochondrial fission (Fis1 and Drp1) were decreased(Reference Ryan, Craig and Folmes24). Furthermore, current evidence has illustrated that treatment with 0·1 nm-1,25(OH)2D3 in human primary muscle improved mitochondrial morphology (volume and structure) and altered mRNA expression of pyruvate dehydrogenase kinase 4 and carnitine palmitoyltransferase 1 (CPT1), important genes that control muscle glucose and lipid metabolism(Reference Pramono, Jocken and Blaak86). Vitamin D deficiency is known to impair muscle function and metabolism, where, in this case, skeletal muscle fibres are most likely to VDR ablation and to uptake cytoplasmic Ca2+ released from the sarcoplasmic reticulum during twitch responses(Reference Rudolf, Mongillo and Magalhaes87). Experiments with chick muscles deficient in vitamin D observed changes in oxidative phosphorylation and a failure of muscle mitochondria to maintain Ca2+, leading to impairment of cellular metabolic homeostasis, increased reactive oxygen species and cytotoxicity due to mitochondrial dysfunction(Reference Mukherjee, Zerwekh and Nicar88). Despite previous reports, there are still no studies that have reported whether the effects of vitamin D up-regulate or down-regulate genes and proteins associated with the tricarboxylic acid cycle and electron transport chain in primary skeletal muscle cells.
Actions and targets of vitamin D
The outcomes of vitamin D action appear to be dependent on interaction with a nuclear VDR and with the retinoid X receptor. This complex is able to up-regulate and/or down-regulate target genes by binding to regulatory sequences named vitamin D3 response elements (Fig. 1)(Reference Hii and Ferrante89). It is estimated that approximately 3 % of the human genome is regulated, directly and/or indirectly, by the vitamin D–endocrine system(Reference Bouillon, Gielen and Vanderschueren90). Genomic mechanisms may explain how supplementation of vitamin D influences, for instance, muscle hypertrophy in adults. Gene expression was evaluated using muscle biopsies in a retrospective cohort of healthy volunteers(Reference Hassan-Smith, Jenkinson and Smith11). In this study, a positive correlation between serum active vitamin D and genes encoding for transcriptional activators or co-repressors of TGF-β and myostatin were observed, resulting in anti-proliferative effects(Reference Hassan-Smith, Jenkinson and Smith11). Associations between vitamin D levels and up-regulation of gene expression were also found with molecules involved in protein synthesis pathways, such as IGF-1 receptor and eukaryotic translation initiation factor 4E-binding protein 1 (EIF4BP1) and eukaryotic translation initiation factor 2B subunit α (EIF2B1), which are members of a family of translation repressor proteins(Reference Hassan-Smith, Jenkinson and Smith11). Additionally, it has been established that 1,25(OH)2D3 regulates proteins that effect contractility and cell regeneration in a rat model of muscle crush injury(Reference Stratos, Li and Herlyn91). In the present study, vitamin D treatment increased cell proliferation of non-satellite cells, including fibroblasts, endothelial cells, Pax7-negative local stem cells and prolyl-4-hydroxylase-β expression (P4HB), which have a direct impact on collagen synthesis. Treatment with vitamin D also increased the production of extracellular matrix components that can similarly affect muscle contractility and force(Reference Stratos, Li and Herlyn91).
Another possible genomic mechanism of vitamin D involves the stimulation of IGF-1(Reference Matar, Al-Shaar and Maalouf92). A study assessing rickets patients detected that circulating concentrations of IGF-1 increased significantly after vitamin D treatment and this may regulate the pituitary gland and growth hormone production(Reference Sollman, Al Khalaf and AlHemaidi93). More recently, a post hoc analysis of the Styrian Vitamin D Hypertension Trial did not find any significant effects of vitamin D supplementation on IGF-1 concentrations in hypertensive patients with low 25(OH)D levels at baseline; however, a significant effect was detected in a cross-sectional correlation between the active form of 1,25(OH)2D3 and IGF-1(Reference Trummer, Schwetz and Pandis94). Therefore, further studies are necessary to clarify the relationship between vitamin D and IGF-1/growth hormone. It is also known that vitamin D influences pathways of apoptosis in a variety of cells, including osteoblasts, osteocytes and tumour cells, resulting in anti-apoptotic effects(Reference Stratos, Li and Herlyn91). These findings suggest that vitamin D acts via VDR/inositol triphosphate (IP3) and Akt, consequently reducing caspase activity and increasing cell survival.
The typical steroid hormone (genomic pathway) is characterised by direct regulation of gene expression, which mediates the responses to the hormone some hours after hormone-receptor binding. In contrast, the non-genomic pathway is characterised by activation of a rapid second messenger which results in acute (mostly seconds) cellular responses and it is not dependent upon the immediate regulation of gene transcription(Reference Norman, Nemere and Zhou31). 1,25(OH)2D3 stimulates fast transcriptional-independent effects (seconds to minutes range) that are not feasibly explained by alterations in gene expression(Reference Ceglia17). Although there is no consensus about the non-genomic pathways of vitamin D, studies have suggested that the actions of vitamin D start at the plasma membrane(Reference Huhtakangas, Olivera and Bishop95). Initially, vitamin D binds to a surface or caveolae VDR, which activates c-Src and phosphoinositide-3 kinase and leads to the fast recruitment of Ca2+ from the sarcoplasmic reticulum into the cytosol. This results in the activation of phospholipase Cy and release of IP3 and diacylglycerol from the membrane. Subsequently, IP3 facilitates the release of Ca2+ from the sarcoplasmic reticulum into the cytosol(Reference Buitrago, Arango and Boland96,Reference Morelli, de Boland and Boland97) .
Another non-genomic mechanism of vitamin D action is promotion of the translocation of PKC-α from the cytosol to the cell membrane, which has been previously studied using chick and rat myoblasts with in vitro 1,25(OH)2D treatment (Fig. 1)(Reference Capiati, Vazquez and Tellez Inon98,Reference Vazquez and de Boland99) . PKC also activates the L-type voltage-dependent Ca2+ channel and store-operated Ca2+ entry (SOCE) channel and may have a role in the activation of extracellular signal-regulated kinases (ERK1/2). Briefly, studies have found a dose-dependent increase in intracellular muscle Ca2+ uptake after treatment with the active form of vitamin D, which may have an impact on muscle contraction(Reference Capiati, Vazquez and Tellez Inon98,Reference Vazquez, Boland and de Boland100,Reference Boland101) . In addition, the activation of c-Src results in Raf-1 stimulation(Reference Morelli, Buitrago and Boland102,Reference Buitrago, Pardo and de Boland103) , which leads to the activation of the MAPK pathway. Subsequently, ERK1/2 activation and phosphorylation of ETS domain-containing protein (Elk-1) and cAMP response element-binding protein increases the expression of c-myc and c-fos, which are key regulators of proliferation and differentiation(Reference Ronda, Buitrago and Colicheo104). Finally, vitamin D also activates p38 MAPK and consequently phosphorylates heat-shock protein 27 which has a significant role in remodelling muscle cells through the actin microfilament system (Fig. 1)(Reference Buitrago, Ronda and de Boland105,Reference An, Fabry and Mellema106) . Even though this seems like a non-genomic effect of vitamin D through direct and acute stimulation of the AKT pathway, it is also known that both genomic and non-genomic effects are interdependent. For instance, extra nuclear-initiated actions may regulate gene expression indirectly through their effects in pathways that themselves control transcription(Reference Ordonez-Moran and Munoz107). New studies focusing on genomic and especially non-genomic mechanisms of vitamin D are required to better distinguish the precise contribution of each mechanism of action and optimal dose–effect for skeletal muscle tissue.
Clinical studies and perspectives
The clinical outcomes of vitamin D supplementation for skeletal muscle physiology have recently received increased attention (the last decade) and could result in a better understanding of the mechanisms of vitamin D effects when analysed from the perspective of the genomic pathways induced by the hormone. A vitamin D inadequacy or deficiency induces muscle fibre atrophy, slow twitch peak, may promote long periods of muscle relaxation and increased risk of chronic musculoskeletal pain(Reference Pfeifer, Begerow and Minne15). Muscle biopsies from vitamin D-deficient adults demonstrate impairment in skeletal muscle tissue, such as fibrosis, loss of type II fibre complement and enlarged interfibrillar spaces(Reference Ceglia17,Reference Yoshikawa, Nakamura and Tanabe108) . Type II fibres are responsible for fast muscle contraction and are used predominantly to prevent falls and in power exercises and anaerobic activities(Reference Ceglia, Niramitmahapanya and da Silva Morais109).
Many studies investigated healthy patients with baseline low serum vitamin D levels followed by supplementation with vitamin D. In these studies, vitamin D supplementation led to the activation of the VDR in skeletal muscle and consequently improved protein synthesis and muscle strength, as well as increased size and number of type II muscle fibres(Reference Bhat, Kalam and Qadri75,Reference Sorensen, Lund and Saltin110–Reference Sato, Iwamoto and Kanoko112) . A systematic review reported that vitamin D supplementation increased muscle strength (1·4 to 18·8 %) in vitamin D-insufficient athletes(Reference Chiang, Ismaeel and Griffis21). In this review, the quality of controlled trials was assessed using the PEDro scale, and five RCT were identified as excellent quality and one controlled trial as good quality. Furthermore, a reduced injury incidence was reported in a group of elite ballet dancers following 4 months of 2000 IU/d of vitamin D supplementation(Reference Wyon, Koutedakis and Wolman113). In a study with elite ballet dancers, significantly fewer injuries were observed after 4 months of vitamin D supplementation(Reference Wyon, Koutedakis and Wolman113). Interestingly, the majority of studies investigating the effects of vitamin D in exercise performance have reported positive results in vitamin D-deficient athletes and not with adequate or supraphysiological concentrations of vitamin D. Hence, further studies are required to elucidate if supraphysiological doses of vitamin D have an ergogenic effect in vitamin D-replete athletes in different sport disciplines. Chronic long-term modifications observed in clinical studies suggest that such consequences are primarily a result of genomic actions. In disparity, genomic secondary changes in gene expression can also arise from alterations in sustained non-genomic/acute signalling.
Recent findings validated the involvement of vitamin D in the regulation of numerous skeletal and extra-skeletal cellular processes that have a direct impact on muscle function and metabolism; however, the pathways involved are incompletely understood. Vitamin D has been reported to positively influence protein synthesis, as well as increase the size of adult muscle cells and muscle mass (in animal and human studies), which could consequently result in increased muscle strength, and/or performance. Moreover, increments in skeletal muscle mass can raise the RMR and consequently the daily energy expenditure, which may benefit in the reduction of body weight in obese individuals. Three meta-analyses of RCT(Reference Muir and Montero-Odasso114–Reference Beaudart, Buckinx and Rabenda116) reported a positive effect of vitamin D supplementation on muscle function and strength in healthy adults, while five cohort studies identified a link between serum 25(OH)D concentration and muscle strength and function(Reference Houston, Tooze and Davis117–Reference Houston, Tooze and Neiberg121). In older adults, a recent meta-analysis found that vitamin D supplementation significantly improved muscle strength of the lower limb and femoral neck bone mineral density(Reference Antoniak and Greig122). Moreover, Chanet et al. (Reference Chanet, Verlaan and Salles123) have proved in healthy older men that supplementing breakfast with vitamin D and leucine enhances the postprandial muscle protein synthesis and muscle mass(Reference Chanet, Verlaan and Salles123). Higher muscle mass is associated with higher muscle strength, which is an important variable to prevent sarcopenia, falls and muscle disorders, as well as being associated with enhancement of performance in athletes. Valuable nutritional interventions combining vitamin D and amino acid supplementation could positively support muscle fibre protein synthesis in specific conditions, for example sarcopenia, where vitamin D and amino acid responses are deficient(Reference Wagatsuma and Sakuma124). Vitamin D status is also a regulator of intestinal Ca absorption and, in turn, bone mineralisation(Reference Sadat-Ali, Al Elq and Al-Turki125). In this context, several reviews have concluded that there is a positive outcome for supplementation of Ca plus vitamin D for fracture risk reduction, osteoporosis and/or falls(Reference Girgis, Clifton-Bligh and Turner6,Reference Neal, Sykes and Rigby126–Reference Ross, Manson and Abrams128) . In contrast, recent meta-analyses have not been consistent with these outcomes, though many variables need to be considered when comparing studies, for example, the parameters analysed, age of the population, if participants live in residential institutions, dose and cut points of vitamin D, ethnicity, period of the intervention, instruments to assess Ca and vitamin D intake and sunlight exposure, and many others(Reference Zhao, Zeng and Wang129). Another important variable to consider in supplementation studies is if the participant is classified as sufficient or deficient in vitamin D since the baseline period, as many of the biological effects of vitamin D are evident when status of vitamin D is corrected from deficient to normal levels(Reference Wyon, Koutedakis and Wolman113,Reference Antoniak and Greig122,Reference Barker, Schneider and Dixon130–Reference Farrokhyar, Sivakumar and Savage137) .
Nutrition strategies to complement the adaptive response to exercise have been well investigated. The effects of supplementation of vitamin D on exercise and muscle health have recently been reported in soccer and rugby players, elite ballet dancers, and in active adult males that were vitamin D insufficient at the baseline(Reference Wyon, Koutedakis and Wolman113,Reference Barker, Schneider and Dixon130–Reference Jastrzebska, Kaczmarczyk and Jastrzebski136) . Four studies(Reference Wyon, Koutedakis and Wolman113,Reference Barker, Schneider and Dixon130,Reference Close, Leckey and Patterson131,Reference Jastrzebska, Kaczmarczyk and Jastrzebski136) have found that supplementation of vitamin D significantly increased strength, power and exercise performance even with a small sample size, while three studies reported no significant effect of vitamin D on any physical parameter(Reference Close, Russell and Cobley132–Reference Todd, McSorley and Pourshahidi134). Further, a reduced injury incidence was reported in a group of elite ballet dancers following 4 months of 2000 IU/d of vitamin D supplementation(Reference Wyon, Koutedakis and Wolman113). Additionally, in a RCT, Owens et al. (Reference Owens, Sharples and Polydorou25) showed that elevating serum 25(OH)D concentrations to >75 nmol/l of 25(OH)D after supplementation with vitamin D3 at 4000 IU/d had a positive effect on the recovery of force following a protocol of damaging eccentric exercise(Reference Owens, Sharples and Polydorou25). In summary, evidence supports a role for vitamin D in muscle remodelling and function; however, further work is needed to clarify under what specific conditions vitamin D can be beneficial.
An important limitation related to the effect of vitamin D in muscle strength is the lack of agreement on the optimal range in blood. While plasma values of >50 nmol/l of 25(OH)D have been demonstrated to improve physiological function and assist with disease prevention, it is believed that different tissues have distinct responses to the levels of vitamin D. Hence, it is possible that the optimal 25(OH)D concentration for skeletal muscle metabolism is different from the optimal concentration for other organs/tissues(Reference Aloia and Li-Ng138,Reference Giovannucci139–Reference Biesalski, Aggett and Anton145) . For example, Heaney & Holick(Reference Heaney and Holick146) proposed that a higher (120–225 nmol/l) serum total 25(OH)D concentration may be required for optimal skeletal muscle physiology in adults, whilst Bischoff-Ferrari et al. (Reference Bischoff-Ferrari, Giovannucci and Willett147) recommended a 25(OH)D concentration of 90–100 nmol/l to enhance bone mineral density and prevent fractures in young adults. Conversely, the metabolic effects of vitamin D supplementation in non-deficient athletes remain to be clarified. In accordance with previous research, it is possible that higher serum levels of vitamin D benefit sports performance(Reference Wyon, Koutedakis and Wolman113,Reference Barker, Schneider and Dixon130,Reference Close, Leckey and Patterson131,Reference Jastrzebska, Kaczmarczyk and Jastrzebski136) . For example, in vitro treatment with high vitamin D levels (400, 1000 nm) stimulates differentiation of skeletal muscle cells and results in the maturation of myoblasts, leading to the developing of an increase in myotube fibre diameter(Reference Van der Meijden, Bravenboer and Dirks59).
Vitamin D can also affect mitochondrial metabolism, increasing O2 consumption, ATP production and modulating RMR and energy production. Vitamin D supplementation could result in significantly better efficiency regarding energy metabolism, perhaps leading to better health outcomes, for example, preventing/reducing obesity and/or weight gain. However, insufficient data exist to confirm the effects of vitamin D on mitochondrial muscle metabolism. Observational research seems promising; however, more RCT are important to assess the effects of vitamin D supplementation in the context of muscle metabolism and function.
Conclusion
Vitamin D is associated with enhanced muscle structure and function, although the mechanisms are not yet fully understood. There is a wide range of muscle disorders related to vitamin D deficiency, and supplementation with vitamin D has mostly shown beneficial effects by counteracting the progression of diseases such as myopathy, sarcopenia, rickets and muscular dystrophy. The effects of vitamin D on proliferation and differentiation of skeletal muscle tissue in human and animal studies remain partially conflicting and need to be clarified. Finally, substantial evidence suggests that vitamin D may have the potential to modulate protein synthesis, mitochondrial metabolism as well as energy production, which is likely to make an impact on muscle strength, function and performance. Further research is required to describe the underlying mechanisms of vitamin D action on human muscle tissue, to clarify how these changes are translated into clinical effects and to define the optimal dose–effect conditions for vitamin D to obtain improvements in skeletal muscle function.
Acknowledgements
We thank the School of Pharmacy and Biomedical Sciences and the Curtin Health Innovation Research Institute for financial research support and excellent research facilities, respectively. This research received no specific grant from any funding agency, commercial or not-for-profit sectors. K. R. M., V. C., R. C. and P. N. conceived the idea, and organised and designed the content of the manuscript. K. R. M. wrote the first draft, which was reviewed by V. C., R. C. and P. N. Fig. 1 was designed and prepared by V. C. and K. R. M. and tables by K. R. M. All authors contributed to manuscript revision and agreed with the final submitted version. The authors declare no conflicts of interest.