Introduction
It is now widely accepted that obesity is associated with low-grade chronic inflammation, which contributes to an increased risk of insulin resistance and type 2 diabetes mellitus, as well as other detrimental health consequences linked to obesity( Reference Masoodi, Kuda and Rossmeisl 1 ). Most prior studies have focused on adipocytes as the source of inflammatory mediators in this pathology( Reference Weisberg, McCann and Desai 2 – Reference Xu, Barnes and Yang 5 ). Recently, the gastrointestinal tract has been described as another potential source of inflammation that is associated with diet- and/or obesity-related pathologies( Reference Ding, Chi and Scull 6 ). The intestine is essential for the digestion and extraction of nutrients, such as lipids, carbohydrates and proteins, but its role in metabolic diseases has been poorly investigated over the years. Increased attention has been paid to the link between the gut microbial composition and obesity. The gut microbiota is a source of endotoxins, whose increase in plasma is related to obesity and insulin resistance through increased intestinal permeability in animal models; however, this relationship still needs to be confirmed in humans( Reference Teixeira, Collado and Ferreira 7 ). Furthermore, different studies suggest that normal non-pathogenic enteric bacteria play a key role in diet-induced adiposity because germ-free mice have been reported to have less body fat( Reference Bäckhed, Ding and Wang 8 ) and do not become obese or insulin resistant when subjected to a high-fat diet( Reference Bäckhed, Manchester and Semenkovich 9 ). In addition, strong evidence supports a direct link between metabolic diseases encompassing obesity and intestinal dysbiosis, namely, alterations of the gut microbial composition( Reference Ley, Bäckhed and Turnbaugh 10 ). These findings have driven research interest to converge on making clearer the relationships between the gut microbiota, diet, host metabolism and the immune system( Reference Power, O’Toole and Stanton 11 ).
Defining the sources, causes and mechanisms underlying the development of inflammation during progressive increases in body weight and adiposity is a powerful tool for developing strategies and therapies that prevent or limit the adverse effects of obesity on health. A growing body of evidence suggests that some bioactive compounds present in food, particularly flavonoids, could play a role in obesity through their effects on inflammatory mediators and pathways( Reference Terra, Montagut and Bustos 12 , Reference Terra, Pallarés and Ardèvol 13 ), barrier integrity( Reference Azuma, Shigeshiro and Kodama 14 , Reference Amasheh, Luettig and Amasheh 15 ) and/or gut microbiota composition( Reference Etxeberria, Arias and Boqué 16 – Reference Parkar, Stevenson and Skinner 19 ). Dietary flavonoids are not completely absorbed from the gastrointestinal tract and are metabolised by the gut microbiota, which reaffirms that they and their metabolites may play a key role in the maintenance of intestinal health.
In view of the direct contact and the dual interaction existing between these natural bioactive compounds and the gut microbial ecosystem coupled with their involvement in modulating obesity and inflammation, it can be hypothesised that the effects of flavonoids on obesity-associated pathologies can be partially explained through the flavonoid-mediated beneficial effects on intestinal alterations. It is worth mentioning that most of the studies performed to date on the effects of flavonoids, both in vitro and in vivo, address the issue using models of cytokine-, endotoxin- or chemically induced intestinal inflammation to address severe, acute or chronic inflammation, but few studies have addressed the specific effect of flavonoids on obesity-associated intestinal alterations. However, although various forms of severe intestinal inflammation, including inflammatory bowel disease and obesity-associated intestinal inflammation, show different degrees of severity, these pathologies share common pathways and mechanisms. Taking into account all these factors, the present review will describe the in vivo and in vitro evidence on the effects of flavonoids in modulating the intestinal inflammatory response, barrier integrity and changes in gut microbiota.
Overview of dietary flavonoids: classification, metabolism, absorption and bioavailability
The term flavonoid refers to certain plant-derived bioactive substances, which exert biological responses in mammalian systems. Flavonoids are classified into flavones, flavonols, flavan-3-ols, flavanones, anthocyanidins and isoflavones( Reference Crozier, Jaganath and Clifford 20 ), depending on the level of oxidation of the C-ring. These bioactive compounds are particularly abundant in certain vegetables, fruits, fruit juices, green and black tea, red wine, chocolate and coffee( Reference Manach, Scalbert and Morand 21 ).
The biological properties of flavonoids are closely linked to their bioavailability, intestinal absorption and metabolism in the gastrointestinal tract, which, in turn, depend on their chemical structure and the degree of polymerisation( Reference Thilakarathna and Vasantha Rupasinghe 22 ). Flavonoid metabolism occurs via a common pathway that results in an aglycone form, which can be absorbed from the small intestine. However, most flavonoids are present in food as esters, glycosides or polymers that cannot be absorbed in their original form( Reference Manach, Scalbert and Morand 21 ). To be absorbed, these molecules must be previously hydrolysed by intestinal enzymes, such as lactase phloridzin hydrolase or cytosolic β-glucosidase, or by the colonic microflora. Before they pass into the bloodstream, the aglycones are subjected to some degree of phase II metabolism to obtain methylated, sulfated and/or glucuronidated metabolites( Reference Del Rio, Rodriguez-Mateos and Spencer 23 ). Then, the metabolites reach the liver via the portal bloodstream, where they can also undergo further phase II metabolism. The resulting metabolites can enter into the systemic circulation or can return to the small intestine by means of enterohepatic recirculation( Reference Thilakarathna and Vasantha Rupasinghe 22 ). Flavonoids that are resistant to the action of hydrolytic enzymes are not absorbed in the small intestine and reach the colon. The colonic microbiota hydrolyses glycosides into aglycones and metabolises them into different aromatic acids. Finally, the flavonoids and their metabolic derivatives are mainly excreted through both biliary and urinary pathways( Reference Manach, Scalbert and Morand 21 ). Despite all of this literature, the bioavailability of these compounds remains a controversial point. Most of them are detected in several tissues inside the organism( Reference Manach, Scalbert and Morand 21 ). However, the enormous diversity of chemical structures that can arise after their metabolisation makes it difficult, most of the time, to identify the compound(s) that are responsible for the described effect. In contrast, it is very clear that flavonoids reach the gastrointestinal tract, where they can directly interact with the intestinal cells, which control several digestive and metabolic processes. Consequently, flavonoids and their aromatic bacterial metabolites are postulated to have significant effects on the intestinal environment, which may lead to an important influence on the physiology and biochemistry of the gut, mainly in situations of metabolic disruption such as obesity.
Intestinal alterations in obesity
Obesity-associated intestinal inflammation: the link between high-fat diet, changes in the gut microbiota and metabolic endotoxaemia
The gut microbiota represents an ensemble of micro-organisms that resides in the intestine, where it plays important roles in ensuring proper digestive functioning, in the immune system and in performing a barrier effect. With respect to the major role that the gut microbiota plays in the normal functioning of the body and the different functions it accomplishes, experts currently consider it an ‘organ’. In addition, certain bacteria tend to adhere to the surface of the intestinal mucosa, while others inhabit the lumen. Whereas the bacteria of the mucosal surface interact with the host immune system, the micro-organisms residing in the lumen may be more relevant to metabolic interactions with food or other digestion derivatives( Reference Quigley 24 ). In fact, compelling evidence supports the role of the intestinal microbiota in the regulation of adiposity and body weight, and it has received increased attention from researchers worldwide( Reference Bäckhed, Ding and Wang 8 , Reference Ley, Turnbaugh and Klein 25 – Reference Remely, Tesar and Hippe 28 ).
The highest microbiota density in the human body is found in the colon. This compartment is primarily composed of anaerobic bacteria, such as Bacteroides, Porphyromonas, Bifidobacterium, Lactobacillus and Clostridium, that belong to the most abundant phyla: Bacteroidetes, Actinobacteria and Firmicutes( Reference Balzola, Bernstein and Ho 29 – Reference Arumugam, Raes and Pelletier 31 ). The proportion of each phylum varies between individuals and depends on age, stress, geographical location, as well as on diet ( Reference Morgan, Segata and Huttenhower 32 – Reference Dethlefsen, Huse and Sogin 37 ). Data in human subjects and rodents revealed that 90 % of the normal gut microbiota consists of the Bacteroidetes and Firmicutes phyla, while obesity is linked to changes in their proportions( Reference Ley, Bäckhed and Turnbaugh 10 , Reference Furet, Kong and Tap 38 – Reference Ley, Turnbaugh and Klein 40 ), which can lead to dysbiosis. In this sense, transfer of the colonic microbiota from ob/ob mice to germ-free animals led to increased fat gain, equivalent to an extra 2 % energy retention of the energy consumed, compared with transfer from control mice. These changes were associated with a dysbiosis in obese mice characterised by an enhanced representation of Firmicutes and a reduced representation of Bacteroidetes( Reference Ley, Bäckhed and Turnbaugh 10 ). In obese patients the same results were obtained: the relative proportion of Bacteroidetes was decreased by comparison with lean individuals. Interestingly, this proportion increased with weight loss on two types of low-energy diet( Reference Ley, Turnbaugh and Klein 40 ). However, this modification of the Firmicutes:Bacteroidetes ratio observed in obese individuals was not observed in other studies( Reference Duncan, Lobley and Holtrop 41 ), requiring further studies in this area. In addition, it has been suggested that changes in the composition of the gut microbiota and epithelial functions may play a role in obesity-associated inflammation( Reference Brandsma, Houben and Fu 42 ). However, due to the high micro-organism diversity found between subjects, it has been difficult to obtain clear conclusions about the predominant phyla present in metabolic diseases( Reference Aguirre, Jonkers and Troost 43 ).
Components that originated from gut microbiota, such as lipopolysaccharides (LPS), lipoteichoic acid, peptidoglycan, flagellin and bacterial DNA, can cause immune system activation. Among them, LPS is thought to be a major inducer of the inflammatory response( Reference Cani, Amar and Iglesias 44 , Reference Zhou, Han and Xu 45 ). LPS are large glycolipids that consist of lipid and polysaccharide fractions joined by a covalent bond. They are found in the outer membrane of Gram-negative bacteria, act as endotoxins, and can elicit strong immune responses. The ability of LPS to promote low-grade inflammation and metabolic disturbances may differ, primarily, because of the chemistry of its components, due to the existing variations between strains( Reference Manco, Putignani and Bottazzo 46 ). Under normal conditions, the presence of this endotoxin in the intestinal lumen does not cause negative health effects. However, some factors can favour the transfer of LPS into the circulatory system and cause metabolic endotoxaemia( Reference Geurts, Neyrinck and Delzenne 47 ). It is suggested that the consumption of a high-fat diet induces changes in the gut microbiota, which leads to excessive energy harvesting and storage, and increases intestinal permeability, leading to metabolic endotoxaemia( Reference Caricilli and Saad 48 ).
Animal research has indicated that germ-free mice fed a high-fat diet do not gain weight, exhibit adiposity, or display other metabolic effects, such as insulin resistance. When the microbiota was transplanted from lean mice or genetically or diet-induced obese mice into germ-free mice, the recapitulation of the original phenotype was observed, showing an increase in body fat( Reference Bäckhed, Manchester and Semenkovich 9 ). In genetically obese mice and obese patients, there is a significant change in the composition of the gut microbiota compared with lean controls( Reference Ley, Bäckhed and Turnbaugh 10 , Reference Ley, Turnbaugh and Klein 40 ), and in rodents, these modifications can be induced by the ingestion of a high-fat diet( Reference Ravussin, Koren and Spor 49 ). It has been hypothesised that the body-weight gain is associated with an increase in the capacity of the microbiota to extract nutrients from the diet( Reference Blaut 50 ). However, other mechanisms, such as changes in gut function, have not been fully explored( Reference de La Serre, Ellis and Lee 51 ). It has been suggested that the type of diet consumed, particularly high-fat diets, can contribute to metabolic endotoxaemia( Reference Serino, Luche and Gres 52 ).
In this respect, it has been observed that a high-fat meal promotes the translocation of intestinal endotoxins into the circulation in human subjects( Reference Ley, Bäckhed and Turnbaugh 10 , Reference Power, O’Toole and Stanton 11 ) and in mice( Reference Terra, Montagut and Bustos 12 , Reference Terra, Pallarés and Ardèvol 13 ). Plasma levels of LPS were also shown to increase in response to a 4-week high-fat diet, by genetically induced hyperphagia( Reference Terra, Pallarés and Ardèvol 13 ), or in the blood of mice orally administered with LPS( Reference Azuma, Shigeshiro and Kodama 14 ). Moreover, a link has been revealed between high-fat diet, inflammation and the occurrence of pro-inflammatory products from gut microbiota Gram-negative bacteria in plasma( Reference Teixeira, Collado and Ferreira 7 , Reference Raybould 53 ).
Therefore, an important question is how dietary fat promotes intestinal LPS absorption. One possibility is that dietary fat promotes paracellular leakage of LPS across the intestinal epithelium. This possibility is supported by observations that intestinal–epithelial tight-junction (TJ) integrity is compromised in obese mice( Reference Hamilton, Boudry and Lemay 26 ) and by studies demonstrating that experimental exposure of the intestinal lumen to some fatty acids can cause small-intestinal epithelial damage( Reference Laugerette, Furet and Debard 54 ). An alternative possibility could be that LPS enters the bloodstream by transcellular transport through intestinal epithelial cells. This process could occur through the so-called intestinal-epithelial microfold cells (M-cells), which are permeable to bacteria and macromolecules and facilitate sampling of gut antigens by the underlying lymphoid tissue( Reference Ghoshal, Witta and Zhong 55 ).
Once in circulation, LPS initiates the activation of Toll-like receptor (TLR) 2 and 4, and LPS receptor CD14, leading to increased activation of inflammatory pathways through cytokine release( Reference Cani, Bibiloni and Knauf 56 – Reference Kim, Gu and Lee 58 ), all generally contributing to a state of systemic inflammation associated with obesity and other metabolic disorders (Fig. 1).

Fig. 1 Hypothesis for gut inflammation after a high-fat diet challenge. Changes in gut microbiota after a high-fat diet (HFD) induce an increase in intestinal permeability and activation of immune cells. Consequently, endotoxaemia increases and triggers systemic inflammation and metabolic disorders. TLR4, Toll-like receptor 4; MLCK, myosin light chain kinase; LPS, lipopolysaccharide.
Altered networks in intestinal inflammation: barrier integrity and inflammatory response
The paracellular and transcellular pathways are the two major pathways mediating transmembrane transfer of intestinal bacterial substances. Both mechanisms may be involved in intestinal mucosal barrier damage and bacterial translocation. The paracellular pathway is integrated by TJ, consisting of zonulin/zonula occludens (ZO)-1, occludin, claudins and actin–myosin cytoskeletal proteins. Previous studies have shown that inflammatory cytokines and bacterial antigens can affect the expression level and assembly of these elements, thereby exerting an influence on TJ functions( Reference Luo, Guo and Zhou 59 ). Immune cells, including neutrophils, dendritic cells and monocytes, have also been directly implicated in inducing disturbances in TJ barrier function. It has been postulated that pro-inflammatory cytokine-induced opening of the intestinal TJ barrier is an important mechanism contributing to the TJ barrier defects present in various inflammatory conditions of the gut( Reference Suzuki 60 ). Previous studies( Reference Suzuki, Nagaishi and Yamazaki 61 – Reference Al-Sadi, Guo and Ye 64 ) have shown that myosin light-chain kinase (MLCK) plays a central role in the regulation of intestinal TJ permeability. The activation of MLCK catalyses the phosphorylation of myosin light chain, inducing contraction of the peri-junctional actin–myosin filaments and the opening of the TJ barrier. In contrast, inhibition of MLCK activation prevents this effect( Reference Al-Sadi, Ye and Dokladny 63 ). It has been suggested that the cytokine-mediated barrier dysfunction could be mediated by an increase in NF-κB, which, in turn, activates MLCK gene and protein expression( Reference Ye and Ma 65 ).
Once intestinal bacteria and endotoxins enter the portal vein and/or lymphatic system, they can reach other tissues and organs, leading to a cascade response modulated by inflammatory mediators. This situation can induce a systemic inflammatory response, which further damages the function of the intestinal barrier( Reference Luo, Guo and Zhou 59 ). The endotoxin-signalling pathway includes the binding of LPS to LPS-binding protein (LBP) and its subsequent transfer to the CD14 receptor. LBP-bound LPS initiates inflammation via TLR associated with membrane-anchored CD14( Reference Laugerette, Furet and Debard 54 ). TLR are a family of pattern-recognition receptors that play a key role in the innate immune system. Among all, the TLR4 is expressed at high levels in the intestinal tract, and given that LPS is its specific ligand, TLR4 could be considered the first barrier for recognition of bacterial presence in the gastrointestinal tract. NF-κB is the final effector transcription factor of the TLR4 signalling pathway. It promotes the development of many intestinal diseases and also plays a pivotal role in the translation and transcription of inflammatory mediators( Reference Luo, Guo and Zhou 59 ).
In mammals, the NF-κB family comprises five proteins, including p65 (RelA), RelB, c-Rel, p105/p50 (NF-κB1) and p100/p52 (NF-κB2), which associate with each other to form transcriptionally distinct homo- and heterodimeric complexes; the p65:p50 heterodimer is the most abundant and the most relevant for inflammation( Reference Li and Verma 66 ). In resting cells, the p65:p50 NF-κB heterodimer is sequestered in the cytoplasm by binding to its inhibitory protein, IκB. In response to an inflammatory stimulus, such as LPS, the classical NF-κB activation pathway leads to the activation of the IκB kinase (IkkB), a member of the IKK complex, triggering IκBa phosphorylation (pIκBa). Then, pIκBa is recognised by the ubiquitin ligase machinery, resulting in its polyubiquitination and subsequent proteasomal degradation. After pIκBa degradation, the p65:p50 heterodimers are able to translocate to the nucleus, where they bind to the κB motif found in the promoter or enhancer regions of numerous pro-inflammatory genes to induce their expression( Reference Hayden, West and Ghosh 67 ).
NF-κB target genes include cytokines (for example, TNF-α and IL), adhesion molecules, acute-phase proteins and inducible enzymes (inducible NO synthase (iNOS) and cyclo-oxygenase-2 (COX-2)) among others( Reference Terra, Valls and Vitrac 68 ). All of these genes contain verified NF-κB binding sites in their sequences, providing strong experimental evidence for their direct control by NF-κB( Reference Pahl 69 ). Among all of these genes, the expression of iNOS and COX-2 has been widely studied in relation to intestinal inflammation. In this regard, sustained high NO production by iNOS plays a role in the pathology of chronic inflammatory bowel disease( Reference Toumi, Soufli and Rafa 70 , Reference Wang, Xia and Yu 71 ). During the last decade, it has become increasingly clear that NO overproduction by iNOS is deleterious to intestinal function( Reference Kolios, Valatas and Ward 72 ), thus contributing significantly to gastrointestinal immunopathology. Cyclo-oxygenases are enzymes that are responsible for the metabolism of arachidonic acid, converting it into PG. These products influence a wide variety of biological processes, ranging from homeostasis to inflammation( Reference Martinez-Micaelo, Gonzalez-Abuin and Terra 73 ). There are two cyclo-oxygenase isoforms: the constitutive COX-1 isoform and the inducible COX-2 isoform( Reference Martinez-Micaelo, Gonzalez-Abuin and Terra 73 , Reference Du, Choi and Dong 74 ). As a result of COX-2 induction, PGE2 levels increase at the site of inflammation and can also be detected systemically.
Taken together, these data suggest that high-fat diet-induced changes in the intestinal microbiota could be responsible for metabolic endotoxaemia and for the onset of the corresponding diseases. The causative link between changes in intestinal bacteria populations, endotoxaemia and metabolic disease needs further assessment( Reference Cani, Bibiloni and Knauf 56 ), but the mechanisms probably include altered epithelial permeability, translocation of bacterial products and up-regulation of pro-inflammatory cytokines and hormones produced by gut endocrine cells, mechanisms which might be modulated by flavonoids.
Flavonoid modulation of intestinal inflammation, barrier integrity and gut microbiota
Flavonoid effects on inflammatory pathways
NF-κB plays a key role in the intestinal inflammatory response( Reference Atreya, Atreya and Neurath 75 ); therefore, the compounds that could modulate this inflammatory pathway are an interesting field of investigation. Flavonoid-mediated modulation of the inflammatory response has been extensively studied in several in vivo and in vitro models( Reference Yoshida, Watanabe and Ishida 76 – Reference Vazquez-Prieto, Bettaieb and Haj 80 ); however, there are fewer studies regarding its effects on intestinal inflammation (Table 1).
Table 1 Summary of flavonoid effects on intestinal inflammatory response and barrier function in vivo and in vitro
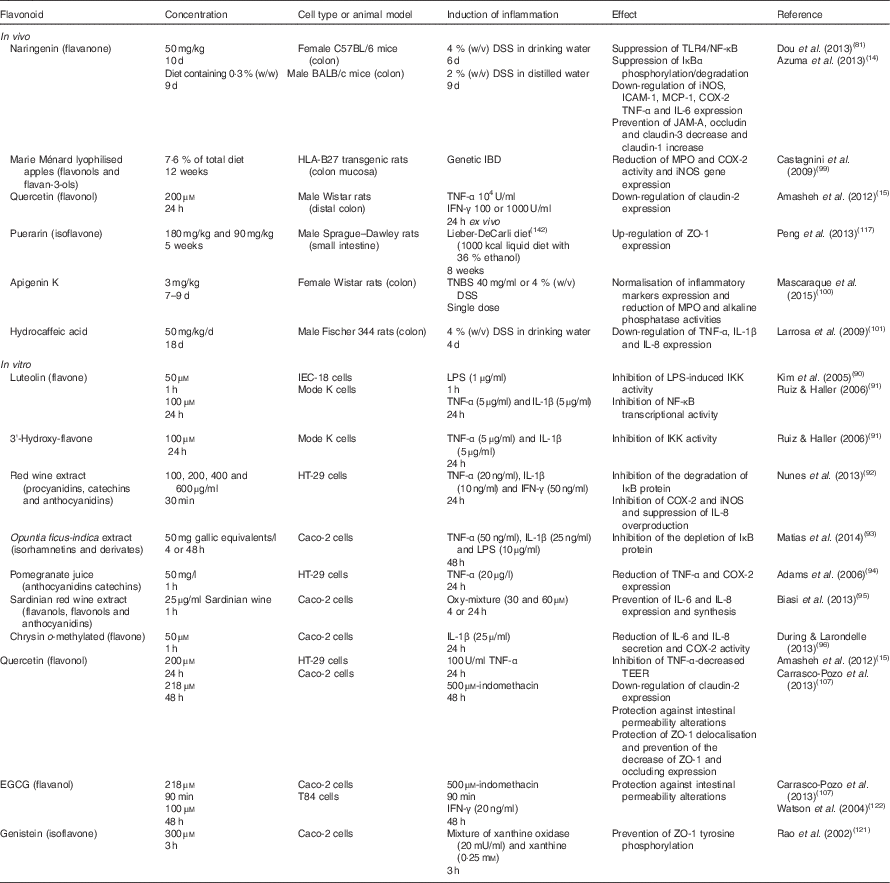
TLR4, Toll-like receptor 4; DSS, dextran sulfate sodium; IκB, inhibitory protein κB; iNOS, inducible NO synthase; ICAM-1, intercellular adhesion molecule-1; MCP-1, monocyte chemotactic protein-1; COX-2, cyclo-oxygenase-2; JAM-A, junction adhesion molecule; MPO, myeloperoxidase; IBD, inflammatory bowel disease; IFN-γ, interferon-γ; ZO-1, zonula occludens-1; TNBS, trinitrobenzenesulfonic acid; LPS, lipopolysaccharide; IKK, IκB kinase; TEER, transepithelial electrical resistance; EGCG, epigallocatechin gallate.
The initial step in the activation of the NF-κB pathway by endotoxins is LPS binding to its receptor TLR4. Dou et al. ( Reference Dou, Zhang and Sun 81 ) studied the effect of naringenin on flavonoid modulation of TLR4 expression in colonic inflammation using female C57BL/6 mice. Naringenin is a flavanone present in citrus fruits that plays an important role as an anti-inflammatory and antioxidant agent( Reference Azuma, Shigeshiro and Kodama 14 , Reference Esmaeili and Alilou 82 ). In this report, colonic inflammation was produced using dextran sulfate sodium (DSS), one of the most widely utilised chemical compounds for inducing an intestinal inflammatory model( Reference Aubry, Michon and Chain 83 – Reference Nighot, Al-Sadi and Rawat 85 ). After a 6 d DSS treatment, both the mRNA and protein expression of TLR4 were significantly increased; however, naringenin treatment inhibited its expression. In the presence of intestinal inflammation, a situation of dysbiosis is observed accompanied by a dysregulation of pattern-recognition receptors that recognise pathogen-associated molecular patterns. One of this pattern-recognition receptor is TLR4, a key receptor for commensal recognition in gut innate immunity and the initial modulator in the activation of the NF-κB pathway. Given that many therapeutic targets that abrogate intestinal inflammation might transect with the TLR4 signalling pathway( Reference Ungaro, Fukata and Hsu 86 , Reference Fiorotto, Scirpo and Trauner 87 ), the results observed by Dou et al. ( Reference Dou, Zhang and Sun 81 ) may give insight into further evaluation of naringenin as a food supplement in the treatment of intestinal inflammation by suppressing the TLR4/NF-κB signalling pathway.
Luteolin is another flavonoid that has been related to NF-κB pathway inhibition. It is a flavone that is abundant in carrots, peppers, celery, olive oil, peppermint, thyme, rosemary and oregano. Luteolin has been shown to produce various beneficial health effects, including antioxidant, anti-inflammatory, antimicrobial and anti-cancer activities( Reference Nepali, Son and Poudel 88 , Reference Lin, Tian and Yi 89 ). Once the membrane receptor is activated by, for example, LPS, the classical pathway of NF-κB activation leads to the phosphorylation of IKK. Kim & Jobin( Reference Kim and Jobin 90 ) observed that IKK activity was suppressed by pretreating IEC-18 cells (a rat non-transformed small intestinal cell line) with luteolin followed by LPS stimulation. The luteolin effect resulted in an inhibition of NF-κB signalling and the consequent pro-inflammatory gene expression in these intestinal epithelial cells. Ruiz & Haller( Reference Ruiz and Haller 91 ) found that treatment with functionally diverse flavonoids, such as 3'-hydroxy-flavone and also luteolin, followed by TNF-α stimulation, inhibited NF-κB signalling by targeting different points of the pathway. They observed that 3'-hydroxy-flavone was able to inhibit IKK activity and that luteolin inhibited NF-κB RelA transcriptional activity in Mode-K cells, a murine intestinal epithelial cell line.
Some authors have demonstrated that flavonoids are able to inhibit the NF-κB translocation to the nucleus, preventing pro-inflammatory gene transcription. This effect can be explained by the protective role that some flavonoids exert over IκB degradation. Nunes et al. ( Reference Nunes, Ferreira and Freitas 92 ) found that a treatment with a red wine extract rich in procyanidins and anthocyanidins significantly inhibited IκB degradation. These results were observed in HT-29 cells (human epithelial colorectal adenocarcinoma cells) stimulated with TNF-α, IL-1β and interferon-γ (IFN-γ). Some in vitro and in vivo studies have proven the effect of flavonoids on IκB degradation. An in vitro study showed that Opuntia ficus-indica juice, also known as cactus pear juice, acted as an antioxidant and anti-inflammatory agent in Caco-2 cells( Reference Matias, Nunes and Poejo 93 ). The extract constituents were flavonoids, such as isorhamnetin and some of its derivates. Pre-treatment with Opuntia extract followed by stimulation with TNF-α, IL-1β and LPS slightly prevented IκB depletion. Moreover, the co-incubation of the extract with these inflammatory inducers led to a more significant effect, showing higher levels of IκB. Other authors( Reference Dou, Zhang and Sun 81 ) also showed similar effects of flavonoids on NF-κB translocation. Specifically, naringenin significantly blocked the NF-κB signalling pathway in DSS-induced colitis by suppressing IκBα phosphorylation/degradation, blocking NF-κB p65 nuclear translocation and inhibiting NF-κB-mediated transcriptional activity.
Upon activation, NF-κB regulates the transcriptional activation of many genes involved in the immune and inflammatory responses, such as pro-inflammatory cytokines (TNF-α, IL-1β and IL-6) and enzymes( Reference Terra, Valls and Vitrac 68 ). The beneficial effect of flavonoids on intestinal inflammation has directly been related to the suppression of pro-inflammatory enzyme expression, such as COX-2 and iNOS. Nunes et al. ( Reference Nunes, Ferreira and Freitas 92 ) observed that pre-treatment with a red wine extract rich in catechins, oligomeric procyanidins and anthocyanidins inhibited COX-2 and iNOS cytokine-induced expression and it also suppressed IL-8 overproduction in HT-29 cells. In another study, also in HT-29 cells, pre-treatment with pomegranate juice, which is rich in anthocyanidins and catechins, reduced TNF-α-induced COX-2 expression( Reference Adams, Seeram and Aggarwal 94 ). This finding may be related to the inhibition of phosphatidylinositide 3-kinase (PI3K) and protein kinase B, preventing the translocation of NF-κB to the nucleus, inhibiting the transcription of genes encoding these inflammatory enzymes. Another hypothesis might be that flavonoids are acting at the same level of the NF-κB pathway but modulating the mitogen-activated protein kinase activity. Either way, the selective inhibition of COX-2 by flavonoids could be an interesting strategy to reduce inflammation without altering the protective role of PG synthesised by COX-1.
Other authors found that the pre-treatment of Caco-2 cells with a Sardinian red wine extract, rich in flavanols, flavolons and anthocyanidins, prevents IL-6 and IL-8 expression and synthesis after being challenged with an oxysterol mixture( Reference Biasi, Guina and Maina 95 ). In addition, During & Larondelle( Reference During and Larondelle 96 ) studied the effects of chrysin, a flavone found in some plants, such as passionflowers or chamomile. They concluded that o-methylated chrysin was able to modulate intestinal inflammation in Caco-2 cells. The cells were pre-treated with both the o-methylated and the non-methylated forms of chrysin, and then stimulated with IL-1β. The results indicated that the o-methylated form was able to reduce IL-6 and IL-8 secretion and COX-2 activity more effectively than the non-methylated form, indicating a structure-related effect. These results are in agreement with other studies that have demonstrated that the o-methylation of flavones improves their intestinal absorption and metabolic stability( Reference Wen and Walle 97 , Reference Walle, Ta and Kawamori 98 ). Due to their increased lifespan in our body, o-methylated flavones are more able to induce potential health effects as compared with their parent unmethylated analogues, according to the observations of During & Larondelle( Reference During and Larondelle 96 ).
It has also been reported that naringenin is able to down-regulate the expression of adhesion molecules (intercellular adhesion molecule-1; ICAM-1), chemokines (monocyte chemotactic protein-1; MCP-1), iNOS, COX-2, TNF-α and IL-6( Reference Dou, Zhang and Sun 81 ) in a model of DSS-induced colitis using female C57BL/6 mice. Furthermore, in a rat model of spontaneous inflammatory bowel disease, Castagnini et al. ( Reference Castagnini, Luceri and Toti 99 ) found that Marie Ménard lyophilised apples, which are rich in flavonols and flavan-3-ols, reduced myeloperoxidase (MPO) activity and COX-2 and iNOS gene expression. MPO is a key component of the oxygen-dependent microbial activity of phagocytes but it has been also linked to tissue damage in acute or chronic inflammation. Beyond its oxidative effects, MPO affects various processes involved in cell signalling and cell–cell interactions and are, as such, capable of modulating inflammatory responses. MPO is considered a marker of disease activity in patients with intestinal inflammation, further highlighting the modulatory effect of flavonoids on this enzyme.
Very recently, Mascaraque et al. ( Reference Mascaraque, González and Suárez 100 ) tested the intestinal anti-inflammatory activity of apigenin K, a soluble form of apigenin, in two models of rat colitis, namely, the trinitrobenzenesulfonic acid model and the DSS model. Apigenin K pre-treatment ameliorated the morphological signs and biochemical markers in both models. Specifically, apigenin K pre-treatment tended to normalise the expression of a number of colonic inflammatory markers (for example, TNF-α, transforming growth factor-β, IL-6, intercellular adhesion molecule 1 or chemokine ligand 2) and to reduce colonic MPO and alkaline phosphatase activities.
It should be noted that flavonoids are metabolised by intestinal cells and gut bacteria, and it is possible that some of the anti-inflammatory properties of flavonoids in the gut might be mediated by their metabolites in addition to or in place of the original compound present in food; however, it has been studied much less. In this sense, Larrosa et al. ( Reference Larrosa, Luceri and Vivoli 101 ) concluded that some polyphenol-derived metabolites from the colon microbiota inhibit DSS-induced colitis lipid peroxidation and DNA damage in the colon mucosa and down-regulate the fundamental cytokines involved in the inflammatory process (TNF-α, IL-1β and IL-8).
In summary, the literature suggests that flavonoids reduce intestinal inflammatory processes driven by NF-κB activation by inhibiting cytokine expression and synthesis and down-regulating the TLR-4/NF-κB pathway in intestinal cell models (Fig. 2). If these observations are confirmed in clinical trials, flavonoid-rich foods or flavonoid supplements may have potential therapeutic and/or preventive applications in the management of intestinal inflammation.

Fig. 2 Schematic view of the anti-inflammatory mechanisms of flavonoids on intestinal inflammation. The mechanisms underlying the anti-inflammatory effects of flavonoids involve, among others, the production and secretion of inflammatory mediators, protection of tight junction cytokine-induced damage and the modulation of the mitogen-activated protein kinase (MAPK) and NF-κB pathways. LPS, lipopolysaccharide; LBP, LPS-binding protein; TLR-4, Toll-like receptor 4; ZO, zonulin/zonula occludens; PI3K, phosphatidylinositide 3-kinase; AA, arachidonic acid; COX-2 cyclo-oxygenase-2; IKK, IκB kinase; IκB, inhibitory protein κB; iNOS, inducible NO synthase.
Flavonoid effects on intestinal mucosal barrier integrity
Because the integrity of the intestinal barrier has been compromised in several intestinal pathologies( Reference de La Serre, Ellis and Lee 51 , Reference Brun, Castagliuolo and Leo 102 , Reference Chen, Zhao and Fu 103 ), the potential protective effects of naturally occurring bioactive compounds have been evaluated in some in vitro and in vivo models (Table 1).
Quercetin is a flavonoid that has been proposed to exert beneficial effects over the intestinal barrier function( Reference Suzuki and Hara 104 ). It is the most common flavonoid in nature and can be found in fruits and vegetables, including onions, kale and apples( Reference Hertog, Hollman and Katan 105 ). Amasheh et al. tested the effect of quercetin on cytokine-induced intestinal barrier damage both in HT-29 cells and in the distal colon from male Wistar rats ex vivo ( Reference Amasheh, Luettig and Amasheh 15 ). In vitro, quercetin was added on both sides of the culture insert and TNF-α was added only to the basolateral side, which produced a decrease in transepithelial electrical resistance (TEER). Interestingly, quercetin treatment partially inhibited this effect. In this study, the expression of claudin-2 was also evaluated. Claudin-2 forms cation-selective channels, and consequently, its up-regulation could contribute to the altered barrier function by allowing the massive transit of cations and water to the lumen( Reference Amasheh, Grotjohann and Amasheh 106 ). In this context, the authors found that quercetin exerts a protective effect on the intestinal barrier by down-regulating claudin-2. The analysis of intestinal permeability in rat colon ex vivo revealed that the application of TNF-α and IFN-γ reduced the total resistance of the intestinal barrier, which was partially inhibited by quercetin.
Carrasco-Pozo et al. ( Reference Carrasco-Pozo, Morales and Gotteland 107 ) tested the effect of quercetin and epigallocatechin gallate (EGCG) against the indomethacin-induced disruption of epithelial barrier integrity in Caco-2 cells. Indomethacin is a non-steroidal anti-inflammatory drug that causes mitochondrial dysfunction, oxidative stress and apoptosis in chronic administration( Reference Carrasco-Pozo, Gotteland and Speisky 108 , Reference Carrasco-Pozo, Speisky and Brunser 109 ). The results showed that quercetin and EGCG completely protected against the indomethacin-induced decrease in TEER. The same results were obtained when the permeability was assessed by measuring fluorescein isothiocyanate-labelled dextran (FD-4) transport across the Caco-2 cell monolayer( Reference Carrasco-Pozo, Morales and Gotteland 107 ). Finally, they evaluated the protective effect of quercetin on ZO-1 and occludin in Caco-2 cells treated with indomethacin and rotenone (an environmental toxin). Immunofluorescence analysis revealed that either indomethacin or rotenone, both inhibitors of mitochondrial complex I, caused TJ disruption through ZO-1 delocalisation. Treatment with quercetin protected ZO-1 delocalisation and also prevented the decrease in ZO-1 and occludin expression. The authors hypothesised that quercetin’s effects may be due to its mitochondrial-protecting property. However, it could also be the result of a modulatory effect of quercetin on the activity of various intracellular signalling molecules that regulate the integrity of TJ. In fact, quercetin has been reported to inhibit isoform-mixed protein kinase C (PKC)( Reference Sim, Lee and Cho 110 ) and PI3K( Reference Agullo, Gamet-Payrastre and Manenti 111 ). The PKC family has been shown to be involved in the barrier function in an isoform-specific manner( Reference Eckert, McCallum and Mears 112 , Reference Helfrich, Schmitz and Zigrino 113 ). Atypical PKCς and -λ are necessary for the maintenance of TJ( Reference Suzuki, Yamanaka and Hirose 114 ), whereas a novel PKCδ is activated by H2O2 and induces TJ disruption( Reference Banan, Farhadi and Fields 115 ). PI3K, also, negatively modulates the intestinal barrier function. Activation of PI3K by oxidative stress dissociates occludin and ZO-1 from the actin cytoskeleton and disrupts barrier function in epithelial cells( Reference Sheth, Basuroy and Li 116 ). Nevertheless, the mechanisms underlying these flavonoid-mediated biological effects have not been fully clarified yet.
The effect of naringenin was evaluated in a murine model of chronic intestinal inflammation( Reference Azuma, Shigeshiro and Kodama 14 ). To induce intestinal damage, male BALB/c mice were fed with 2 % (w/v) DSS. The colonic permeability was studied by measuring fluorescein isothiocyanate-labelled dextran (FD-4) paracellular transport. The authors found that the animals fed with DSS exhibited higher permeability than the control group. In contrast, the DSS + naringenin group did not differ from the control group. Furthermore, the expression of the occludin, junctional adhesion molecule-A, claudin-3 and claudin-1 proteins was decreased in the DSS group. However, the level of these proteins was equivalent to the control group after treatment with naringenin. Taken together, all of these findings suggested that naringenin was able to protect TJ by suppressing DSS-induced damage in the intestinal epithelial cells.
Puerarin (daidzein-8-C-glucoside), an isoflavone extracted from a Chinese medicinal herb, can modulate TJ expression in the altered intestinal barrier in vivo ( Reference Peng, Cui and Huang 117 ). Male Sprague–Dawley rats were fed an ethanol liquid diet producing intestinal barrier dysfunction. In this study, ZO-1 protein expression was significantly down-regulated by ethanol intake, whereas the groups treated with puerarin exhibited an up-regulation of this protein. The authors concluded that the expression of ZO-1 in the ethanol diet rats was indicative of injury to the intestinal barrier function and that puerarin mitigated such intestinal alterations. The negative effect that ethanol exercises at the intestine level is not only due to the alteration of the gastrointestinal epithelial barrier function, and the increment of intestinal permeability, but also includes diminished phagocytosis mediated by Kupffer cells( Reference Shiratori, Teraoka and Matano 118 ) and bacterial overgrowth, among others( Reference Bode and Christian Bode 119 ). Then puerarin may be acting against ethanol-induced injury at different levels to modulate intestinal health, but this hypothesis needs further assessment.
The molecular mechanisms of genistein, quercetin, myricetin and EGCG in protecting the intestinal barrier have been extensively reviewed by Suzuki & Hara( Reference Suzuki and Hara 120 ). These molecules exerted protective and promoting effects on intestinal TJ barrier function. In particular, genistein and quercetin interact with intracellular signalling molecules, such as tyrosine kinases and PKCδ, resulting in the regulation of TJ protein expression and assembly. More specifically, it has been demonstrated that oxidative stress-induced TJ dysfunction is related to the tyrosine phosphorylation of occludin, ZO-1 and E-cadherin in Caco-2 cells( Reference Rao, Basuroy and Rao 121 ). It has been hypothesised that genistein acts against the oxidative stress in the intestinal barrier by suppressing c-Src kinase (a tyrosine kinase) activation, which inactivates tyrosine phosphorylation of the TJ. Furthermore, EGCG’s effects on IFN-γ-induced intestinal barrier dysfunction were evaluated in T84 human colonic cells( Reference Watson, Ansari and Cameron 122 ). The results showed that EGCG restored the decreased TEER values caused by IFN-γ. The authors suggested that the ability of EGCG to limit the IFN-γ-induced increases in epithelial permeability is probably a component of the anti-inflammatory nature of this polyphenol.
Flavonoid–microbiota interaction: modulation of the gut microbiota composition
In mammals, the microbiota is involved in the maintenance and development of the immune system, in the regulation of several metabolic pathways, and in general body homeostasis( Reference Jorth, Turner and Gumus 123 , Reference Guarner and Malagelada 124 ). It has been suggested that both dietary flavonoids, which are the substrates of intestinal bacteria, and the metabolites produced during flavonoid degradation in the colon may modulate and induce oscillations in the composition of the microbiota populations by means of prebiotic and antimicrobial effects against gut pathogenic micro-organisms( Reference Etxeberria, Fernández-Quintela and Milagro 125 – Reference Queipo-Ortuño, Boto-Ordóñez and Murri 127 ). However, the mechanisms involved are still poorly understood. In the following section, we summarise the effects of flavonoids and their metabolites from colonic metabolism on the gut microbiota composition (Table 2).
Table 2 Summary of flavonoid effects and their metabolites on the modulation of gut microbiota composition
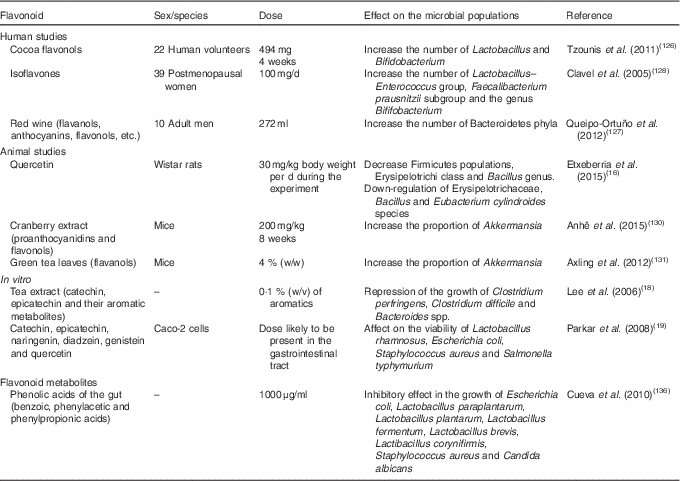
Interesting results were obtained in human studies from Tzounis et al. ( Reference Tzounis, Rodriguez-Mateos and Vulevic 126 ) who evaluated the prebiotic effect of cocoa flavanols in a randomised, double-blind, cross-over intervention study that included twenty-two human volunteers. The administration of 494 mg of cocoa flavanols for 4 weeks significantly increased the number of Lactobacillus and Bifidobacterium populations but significantly decreased the Clostridia counts. These microbial changes were correlated with reductions in plasma C-reactive protein concentrations, which is considered to be a blood marker of inflammation and a hallmark of the acute-phase response( Reference Tzounis, Rodriguez-Mateos and Vulevic 126 ). These changes in the dominant bacterial communities were similar to those found by Clavel et al. ( Reference Clavel, Fallani and Lepage 128 ) in a randomised, double-blind, placebo-controlled study undertaken by thirty-nine postmenopausal women. After 1 month of supplementation with 100 mg/d of isoflavones, the percentages of the Lactobacillus–Enterococcus group, the Faecalibacterium prausnitzii subgroup and the genus Bifidobacterium were significantly increased( Reference Clavel, Fallani and Lepage 128 ). Queipo-Ortuño et al. ( Reference Queipo-Ortuño, Boto-Ordóñez and Murri 127 ) performed a randomised, cross-over, controlled intervention study, in which ten adult men participated. The results showed that a daily consumption of 272 ml of red wine, which is mainly rich in flavanols, anthocyanins, flavonols and other flavonoids, decreased the plasma levels of TAG and HDL-cholesterol, and these significant reductions may be partly due to the flavonoid-induced increase in the number of the Bacteroidetes phylum( Reference Queipo-Ortuño, Boto-Ordóñez and Murri 127 ). Other authors noted a significant reduction in the plasma concentration of C-reactive protein after red wine treatment, which was related to an increase in the percentage of Bifidobacterium ( Reference Hage and Szalai 129 ).
Regarding animals studies, Etxeberria et al. ( Reference Etxeberria, Arias and Boqué 16 ) assessed, in Wistar rats, the potential of quercetin to reverse alterations of the gut microbial composition associated with diet-induced obesity. All of the animals were fed a high-fat sucrose diet, containing 17 % of the energy as sucrose, for 6 weeks, and the treated group was also supplemented with quercetin at 30 mg/kg body weight per d during the experiment. According to the results, quercetin generated a significant impact on different taxonomic grades of the gut microbiota composition. At the phylum level, quercetin administration attenuated the Firmicutes:Bacteroidetes ratio, decreasing Firmicutes populations by 34·2 %. Quercetin also significantly reduced Erysipelotrichi (–83·9 %) and Bacillus (–74·3 %) abundance at the class and genus levels, respectively. Furthermore, the treated group showed a statistically significant down-regulation detected in the mean relative abundance of some bacterial species previously associated with diet-induced obesity (Erysipelotrichaceae, Bacillus and Eubacterium cylindroides). Overall, quercetin administration effectively reduced the high-fat sucrose diet-induced gut microbiota dysbiosis. Meanwhile, Anhê et al. ( Reference Anhê, Roy and Pilon 130 ) evaluated the impact of a cranberry extract rich in proanthocyanidins and flavonols in the modulation of the gut microbiota on mice fed a high-fat sucrose diet. The daily supplementation with 200 mg/kg of cranberry extract for 8 weeks noticeably increased the proportion of the mucin-degrading bacterium Akkermansia ( Reference Anhê, Roy and Pilon 130 ). Similarly, in another study, feeding mice a high-fat diet supplemented with 4 % (w/w) powdered green tea leaves high in flavanols has also been recently associated with an increase in the proportion of Akkermansia after 22 weeks( Reference Axling, Olsson and Xu 131 ). According to the literature, Akkermansia administration as a probiotic was reported to reduce systemic LPS levels in high-fat-fed mice, which is possibly associated with the ability of Akkermansia to preserve the mucus layer thickness, therefore reducing gut permeability and LPS leakage( Reference Everard, Belzer and Geurts 132 ). Therefore, the results discussed previously might suggest another possible modulatory pathway of intestinal barrier integrity by flavonoids, resulting from their demonstrated effect on Akkermansia abundance, in a dysbiosis situation, through the preservation of the mucus layer thickness, although further studies are required in order to confirm this issue.
In vitro studies have focused on evaluating the effect of flavonoids on the growth pattern of intestinal bacteria as an approach to understanding the role of these phytochemicals in the gut microbiota. Lee et al. ( Reference Lee, Jenner and Low 18 ) assessed the influence of the phenolic components of a tea extract, rich in catechin and epicatechin, and their aromatic metabolites upon the growth of common pathogenic, commensal and probiotic intestinal bacteria as representative intestinal microflora. Tea phenolics and their derivatives significantly repressed the growth of specific pathogenic bacteria, such as Clostridium perfringens, Clostridium difficile and Bacteroides spp.( Reference Lee, Jenner and Low 18 ). Furthermore, Parkar et al. ( Reference Parkar, Stevenson and Skinner 19 ) also tested the effect of the most representative dietary flavonoids on the growth of probiotic (Lactobacillus rhamnosus), a commensal (Escherichia coli) and two pathogenic bacteria (Staphylococcus aureus, Salmonella typhimurium), together with their effects on adhesion of pathogenic and probiotic bacteria to cultured Caco-2 cells. The incubation with catechin, epicatechin, naringenin, diadzein, genistein or quercetin affected the viability of representative gut flora in vitro, at doses likely to be present in the gastrointestinal tract. In addition, naringenin showed an effective inhibition of Salmonella typhimurium adherence to Caco-2 enterocytes( Reference Parkar, Stevenson and Skinner 19 ).
In accordance with the literature, the proportion of Bacteroidetes to Firmicutes is altered in obese individuals, which produces signals that control gene expression in epithelial intestinal cells( Reference Ley, Bäckhed and Turnbaugh 10 , Reference Furet, Kong and Tap 38 , Reference Hildebrandt, Hoffmann and Sherrill-Mix 133 ). In addition, the metabolism of flavonoids by gut microbiota includes the cleavage of glycosidic linkages, which generates different products, such as glycans, that are necessary for the survival of the intestinal microbiota. The Firmicutes family possesses fewer glycan-degrading enzymes than Bacteroidetes and is more repressed by antimicrobial effects of flavonoid compounds than the Bacteroidetes family. Instead, the Bacteroidetes family prevails following dietary flavonoid intake, and the flavonoids are fermented to phenolic compounds due to the presence of more glycan-degrading enzymes( Reference Rastmanesh 134 ). Therefore, taking into account the previous evidence, the prebiotic power of dietary flavonoids could be a possible mechanism by which these phytochemical substances exert their beneficial effects.
Oligomeric and polymeric forms of flavonoids are metabolised by the intestinal microbiota into various phenolic acids, including phenylpropionic, phenylacetic and benzoic acid derivatives( Reference Déprez, Brezillon and Rabot 135 ). It has been reported that these metabolites may modulate the growth of bacteria in the gut microbial milieu. As an approach towards the evaluation of their effect in the gut, Cueva et al. ( Reference Cueva, Moreno-Arribas and Martin-Alvarez 136 ) assessed the antimicrobial activity of different phenolic acids against different commensal, probiotic and pathogenic bacteria. Some phenolic acids demonstrated an inhibitory effect on the growth of Escherichia coli ATCC 25922, a non-pathogenic strain, at a concentration of 1000 μg/ml, as well as on the growth of lactobacilli (Lactobacillus paraplantarum LCH7, Lactobacillus plantarum LCH17, Lactobacillus fermentum LPH1, L. fermentum CECT 5716, Lactobacillus brevis LCH23, and Lactobacillus coryniformis CECT 5711) and pathogens (Staphylococcus aureus EP167 and Candida albicans MY1055)( Reference Cueva, Moreno-Arribas and Martin-Alvarez 136 ). Recently, it has been reported that these metabolites may also exert several biological activities, such as the inhibition of platelet aggregation and activation function( Reference Rechner and Kroner 137 ), inhibition of COX-2 in HT-29 colon cancer cells( Reference Karlsson, Huss and Jenner 138 ), reduction in the synthesis of prostanoids in colon cells( Reference Russell, Drew and Scobbie 139 ), antiproliferative activity in prostate and cancer cells( Reference Gao, Xu and Krul 140 ) and, finally, influence cell proliferation, apoptosis and signalling pathways in human colon carcinoma cells( Reference Glinghammar and Rafter 141 ).
To sum up, although there are few studies regarding the effects of flavonoid consumption on the gut microbiota composition, results in this field seem to indicate that the effects of flavonoids in human health depend, to a large degree, on their transformation by the gut microbiota. In turn, flavonoids and their metabolites contribute to the maintenance of gut health, inducing the growth of beneficial bacteria and inhibiting the growth of pathogen species. However, the mechanisms involved in this two-way relationship remain to be clearly elucidated.
Conclusions
Flavonoids are a large and diverse group of natural compounds of which only a few have been evaluated regarding their effect on intestinal alterations. The strongest conclusion that can be drawn from the revision of the current literature is that some flavonoids are able to reduce the intestinal inflammatory processes targeting the TLR4/NF-κB pathway. Although there are few studies regarding the flavonoid effects on intestinal permeability, most of them point out that flavonoids are able to protect barrier integrity by primarily acting on TJ stability. Finally, the review of the literature on the effects of flavonoid consumption on the gut microbiota populations suggests that flavonoids may modulate the microbiota composition by means of prebiotic and antimicrobial properties. However, the mechanisms involved are still poorly understood.
According to the present review, it is essential to establish an adequate animal model to further evaluate intestinal alterations associated with metabolic–homeostasis disruption states, as in obesity. Future investigations are required to elucidate the precise mechanisms underlying flavonoid effects on these obesity-induced intestinal alterations. The progress in this field of investigation may lead to novel therapeutic modalities (for example, probiotics and prebiotics, or immunomodulators) to reduce the impact of the Western lifestyle on whole-body homeostasis.
Acknowledgements
The present review was supported by a grant (no. AGL2014-55347-R) from the Spanish government. K. G.-C. and I. G. received a grant for PhD students from Universitat Rovira i Virgili. M. P. is a Serra Húnter fellow.
Both K. G.-C. and I. G. initiated the literature search, were in charge of drafting the manuscript and designed the figures. Both K. G.-C. and I. G. contributed equally to all parts of the paper. M. P., A. A. and M. B. revised the first drafts. X. T. was responsible for final editing and was responsible for the final content. All authors critically reviewed the manuscript and approved the final version.
The authors declare that they have no conflicts of interest.