Introduction
Autism spectrum disorder (ASD) is a syndrome with various subgroups, boundaries and treatments( Reference Willemsen-Swinkels and Buitelaar 1 ). Symptoms include a reduced ability to socialise, to communicate and to use imagination, and displays of stereotypical behaviour( Reference Filipek, Accardo and Baranek 2 ). The Diagnostic and Statistical Manual of Mental Disorders, fourth edition (DSM-IV) handbook lists five subtypes of ASD: autistic disorder; Asperger's disorder; childhood disintegrative disorder; pervasive developmental disorder not otherwise specified; and Rett's disorder.
Currently, no diagnostically relevant biological tests for ASD exist. Therefore, diagnoses are based on observed deficits in reciprocal social interaction and communication( Reference Newschaffer, Croen and Daniels 3 ). Among the children diagnosed with autism between 6 and 17 years old, the amount of co-morbidities, such as attention-deficit disorder, attention-deficit/hyperactivity disorder, anxiety problems and behavioural problems are reported as high as 87·3 %( Reference Kogan, Blumberg and Schieve 4 ). The prevalence of autism is currently estimated to lie between 45 and 110 per 10 000 individuals in the USA. A recently reported increase( Reference Duchan and Patel 5 ) has been ascribed to increased recognition, changed diagnostic criteria and changing public attitudes towards autism. However, a causal increase by environmental risk cannot be ruled out( Reference Rutter 6 ). Common postulated risk factors for autism include high maternal and paternal age, a low level of parental education, a child of male sex, autism in the family, low birth weight or gestational age, and prenatal virus and drug exposures (for example, rubella infection( Reference Chess, Korn and Fernandez 7 ), thalidomide( Reference Stromland, Nordin and Miller 8 ) and maternal smoking( Reference Hultman, Sparen and Cnattingius 9 )).
There is scientific consensus that autism involves a disorder in brain function and development( Reference Belmonte, Allen and Beckel-Mitchener 10 – Reference Bauman and Kemper 13 ). The cause of this disorder, however, is not clear. Although several genetic factors are known to influence the aetiology of different types of autism( Reference Rutter 14 ), these only apply to a minor part of the autistic population( Reference Sutcliffe 15 , Reference Bailey, Le Couteur and Gottesman 16 ). Moreover, several hypotheses point to environmental influences as possibly being causative to autism, including involvement of abnormal gastrointestinal (GI) microbiota composition, autoimmunity, early environmental exposures to viruses and drug compounds( Reference Yap, Li and Saric 17 ).
The ambiguity in causation has resulted in a wide variety of proposed treatments( Reference Gerlach 18 ). With this, autistic patients are currently subjected to numerous ‘alternative interventions’ by caregivers and researchers( Reference Whiteley, Rodgers and Savery 19 , Reference Whiteley and Shattock 20 ). Some of these are specifically aimed at the diet and improving gut health. Described interventions include gluten- and casein-free diets( Reference Whiteley, Rodgers and Savery 19 , Reference Millward, Ferriter and Calver 21 ), supplementation with pre- and probiotics( Reference Parracho, Bingham and Gibson 22 ) and supplementation with multivitamins( Reference Adams, Audhya and McDonough-Means 23 – Reference Adams, George and Audhya 25 ).
In the present review, we evaluate the current theories and hypotheses concerning the aetiology of autism, with a special focus on the gut–brain axis. On this axis, the mechanisms and interactions of sensory cues and biochemical signals that take place between food, the GI tract and the nervous system can best be described. Through this approach, we discuss the possible alternative nutritional interventions and their efficacy on reducing symptoms of ASD.
Discussion
To place the nutritional interventions in context, it is important to study the causation of disorders in brain function and development before developing symptoms related to the different subcategories of ASD. For the purpose of the review, available studies related to the gut–brain axis are described.
Gastrointestinal abnormalities
GI abnormalities in the gut of autistic children compared with healthy or sibling controls have been studied in multiple trials( Reference Parracho, Bingham and Gibson 22 , Reference Song, Liu and Finegold 26 – Reference White 36 ). In this respect, studies by Valenci-McDermott et al. ( Reference Valenci-McDermott, McVicar and Rapin 37 ) and Adams et al. ( Reference Adams, Johansen and Powell 29 ) have indicated that 70 % of children with ASD report having a history of GI complaints, against 28 % of neurotypical controls, and that GI symptoms are strongly correlated with the severity of autism (r 0·59; P< 0·001). The GI abnormalities found in autistic children include malabsorption( Reference Goodwin, Goodwin and Cowen 38 ), maldigestion( Reference Sun and Cade 39 , Reference Sun, Cade and Fregly 40 ), microbial overgrowth (fungal, bacterial and viral)( Reference Finegold, Dowd and Gontcharova 31 ) and abnormal intestinal permeability( Reference de Magistris, Familiari and Pascotto 28 , Reference D'Eufemia, Celli and Finocchiaro 41 ). These events could cause symptoms including diarrhoea, constipation, gas, belching, probing and visibly undigested foods( Reference Kerwin, Eicher and Gelsinger 42 ).
In the study of Wakefield et al. ( Reference Wakefield, Murch and Anthony 43 ), twelve children with regressive autism and GI abnormalities were examined. Of these children, ten displayed lymphoid-nodular hyperplasia (LNH) and eight also displayed abnormalities in the mucosa (including granularity, loss of vascular pattern, and patchy erythema). There were no neurological abnormalities detected in the children.
In 2004( Reference Wakefield, Ashwood and Limb 44 ), the same research group conducted a comparable study among 148 children with ASD as well as GI symptoms and thirty developmentally normal controls by ileo-colonoscopy. LNH was found in the ileum of 90 % of autistic children v. 30 % of controls and in the colon of 59 v. 23 % of autistic children and controls, respectively. The presence and severity of ileal LNH was not influenced by diet or age at colonoscopy.
Another study( Reference Horvath, Papadimitriou and Rabsztyn 27 ) examined thirty-six children with autism and abnormal gastroenterological symptoms. In this study, reflux oesophagitis in twenty-five of the children, chronic gastritis in fifteen, and chronic duodenitis in twenty-four were observed. Likewise, twenty-one children showed low activity of carbohydrate digestive enzymes in the intestine, and twenty-seven showed increased exocrine secretion of pancreatic–bilary fluid after intravenous administration of the GI hormone secretin (the peptide hormone secretin, released by endocrine cells within the duodenal mucosa, promotes sodium bicarbonate and water secretion by the pancreas( Reference White 36 )).
However, in a study of Black et al. ( Reference Black, Kaye and Jick 33 ), it was found that ninety-six children, that at a later stage were diagnosed as ‘autistic or ‘possibly autistic’, did not suffer more from GI inflammation, coeliac disease, food intolerance or recurrent GI symptoms than the 449 controls without subsequent development of autism. In a recent study( Reference Ibrahim, Voigt and Katusic 34 ), including 124 children with autism and two matched controls per case, the lifetime incidence of GI symptoms was found to be 77·2 and 72·2 %, respectively. Concentrating on the five formed categories of GI symptoms (constipation; diarrhoea; abdominal bloating, discomfort or irritability; gastro-oesophageal reflux/vomiting; and feeding issues or food selectivity), the autistic children did experience significant higher rates of constipation and ‘feeding/food selectivity issues’ (specified feeding problems, lactose intolerance, loss of appetite or loss of weight) than did controls. It is suggested, however, that these two categories are primarily influenced by behavioural rather than biological influences( Reference Ibrahim, Voigt and Katusic 34 ).
Microbiotic compositions: clostridia and vancomycin
Bolte( Reference Bolte 45 ) hypothesised that abnormal gut microbiota may be involved in the aetiology of ASD patients. Finegold et al. found that children diagnosed with late-onset autism differed from non-autistic controls in microbiotic compositions of faecal flora, gastric and small-bowel samples( Reference Finegold, Molitoris and Song 32 ). The authors state that there are several reasons to consider that micro-organisms may be involved in late-onset autism. First, onset of the disease often follows antimicrobial therapy, for example, to treat ear infections that often are present in high frequency and persistency among young ASD patients( Reference Konstantareas and Homatidis 46 – Reference Niehus and Lord 48 ). Second, GI symptoms are common at the onset of ASD and often persist. Finally, other antimicrobials may lead to a clear-cut response and relapse may occur when the antimicrobial is discontinued, which is demonstrated with, for example, the antimicrobials vancomycin( Reference George, Volpicelli and Stiner 49 ) and metronidazole( Reference Portnoy, Soneji and Murray 50 ). However, in higher doses, over a longer period of time (>6 d of treatment), vancomycin disrupts the anaerobic intestinal microflora and promotes colonisation by pathogens( Reference Pultz, Stiefel and Donskey 51 ).
Indeed, significantly more clostridia and ruminococci were found in the stools of the autistic group (geometric mean count of 2·1 × 106 colony-forming units) v. the control group (1·6 × 105 colony-forming units) using 16S ribosomal RNA gene sequencing. Non-spore-forming anaerobic and micro-aerophilic bacteria were found in four of five children with autism, whereas none of the control children showed these bacteria. Furthermore, both the gastric and small-bowel specimens from children with autism were more likely to have (a higher number of species of) clostridia than was true for the controls. Song et al. ( Reference Song, Liu and Finegold 26 ) found significantly higher mean cell counts of Clostridium cluster groups I and XI and Clostridium boltae in fifteen autistic children compared with eight controls using real-time PCR. Parracho et al. ( Reference Parracho, Bingham and Gibson 22 ) used fluorescence in situ hybridisation, and reported higher levels of C. histolyticum in fifty-two autistic children compared with both healthy unrelated children and healthy siblings (P< 0.01 and P< 0·05, respectively). However, no relationship was evident between the levels of any of the bacterial populations examined and age, sex, antibiotic history or diet type. GI problems were associated with high levels of clostridia (P< 0·001) in patients with ASD, but this is apparent, as 91·4 % of the autistic group and none of the unrelated control group had GI symptoms( Reference Parracho, Bingham and Gibson 22 ). Martirosian et al. ( Reference Martirosian, Ekiel and Aptekorz 52 ) detected higher levels of C. perfringens, but equal amounts of Clostridium spp. in forty-one autistic children compared with ten non-autistic controls using anaerobic bacterial cultivation.
Concluding from the findings listed above, it was hypothesised that: (1) relapse in autistic children after discontinuation of antibiotic treatment is due to the presence of Clostridium spores which then germinate to reproduce the disease; (2) the increased incidence of autism is related to the widespread exposure to Clostridium spores in the environment; and (3) the increase in families with multiple cases of autism is also due to contact with spores. However, the studies conducted so far are of low to moderate quality, predominantly due to small sample sizes and inadequate or absent explanation of sources of the sample, timing of the study and potential biases (for an extensive review, see also Cao et al. ( Reference Cao, Lin and Jiang 53 )). In addition, the studies used a wide range of different assessment methods, which makes it impossible to make qualitative comparisons. Clearly, carefully designed studies are warranted to verify any cause–effect relationships.
Indolylacryloylglycine
Another aspect in the relationship between micriobiota and (late-onset) ASD involves urinary indolyl-3-acryloylglycine (IAG). IAG is a regular constituent of human urine and is produced by gut microflora( Reference Anderson, Bendell and Garnett 54 , Reference Bull, Shattock and Whiteley 55 ). It has been speculated that high levels of IAG in urine are an indication of gut dysbiosis( Reference Shattock, Kennedy and Rowell 56 ). A few studies found increased levels of IAG in autistic individuals compared with asymptomatic controls( Reference Anderson, Bendell and Garnett 54 , Reference Bull, Shattock and Whiteley 55 ).
Normally, tryptophan is catabolised to indole pyruvate and indole acetate and can be detected in the urine of normal subjects( Reference Moore, Jessop and Osborne 57 ). The formation of IAG is possibly the result of another less-investigated pathway. It is likely that intestinal micro-organisms catabolise tryptophan to indole derivatives which are then absorbed and converted to indolylacrylic acid (IAcrA) and after conjugation of IAcrA with glycine in the liver IAG is formed( Reference Marklová 58 ). Shattock et al. ( Reference Shattock, Whiteley and Savery 59 ) hypothesised that IAG represents the detoxified version of a acidic precursor that affects membranes throughout the body, particularly those lining the gut wall and the blood–brain barrier, making them permeable to other biologically active products such as peptides. This would occur either by replacing the (flat) long-chain fatty acids that make up the lipid elements of the membrane, or by inserting itself between these layers( Reference Shattock and Whiteley 60 ). However, very little evidence exists to support this suggestion. To the best of our knowledge, only one publication showed that the levels of highly unsaturated fatty acids in erythrocyte membranes are affected by the presence of IAcrA( Reference Bell, Sargent and Tocher 61 ).
Abnormal intestinal permeability
Two studies( Reference de Magistris, Familiari and Pascotto 28 , Reference D'Eufemia, Celli and Finocchiaro 41 ) found an abnormally high intestinal permeability in autistic children, compared with normal controls. D'Eufemia et al. ( Reference D'Eufemia, Celli and Finocchiaro 41 ) included twenty-one autistic and forty healthy age-matched children, both groups without clinical evidence of any GI disease or allergy. The results highlighted some damage to tight junctions of the gut mucosa, and showed that in some patients with infantile autism damage to these junctions occurs in the absence of established GI disorders. Recent results from a study by de Magistris et al. ( Reference de Magistris, Familiari and Pascotto 28 ) replicated the finding of an abnormal intestinal permeability in 36·7 % of ninety autistic children, as well as in 21·2 % of their 146 siblings. This was significantly higher than in the 146 healthy controls (4·8 %). They also found GI symptoms in 46·7 % of the autistic children, although these symptoms were not related to the intestinal permeability. Furthermore, ASD patients on a reported gluten-free, casein-free (GFCF) diet had significantly lower intestinal permeability values compared with those on an unrestricted diet and controls. Specific causes were not described, although the authors suggest the existence of a genetic GI factor that is involved in the pathology of a subgroup of ASD( Reference de Magistris, Familiari and Pascotto 28 ).
Previously, intestinal permeability was found to be involved in the aetiopathogenesis of several autoimmune diseases, including Crohn's disease( Reference Secondulfo, De Magistris and Fiandra 62 ), coeliac disease( Reference Munkholm, Langholz and Hollander 63 ) and type 1 diabetes( Reference Watts, Berti and Sapone 64 ). Significantly lower levels of the health-promoting bifidobacteria species and the mucolytic bacterium Akkermansia muciniphila were found in children with autism( Reference Wang, Christophersen and Sorich 65 ). It is suggested that low levels of bifidobacteria are related to unhealthy (more putrefactive) gut microbiome composition and metabolism. The change in A. muciniphila suggests mucus barrier changes. A. muciniphila is a mucin-degrading bacterium extensively present in the guts of healthy adults, but reduced in patients with Crohn's disease, ulcerative colitis and in elderly individuals. A thinner GI mucus barrier could represent less substrate for mucin-degrading bacteria and hence lower numbers in the faeces. Thus, although a degraded mucus barrier and less mucus-degrading bacteria seems paradoxical, A. municiphila could be a possible marker for altered mucus turnover and a thin, thus possibly more ‘leaky’, gut barrier( Reference Kleerebezem and Vaughan 66 ).
However, another recent study showed no abnormal intestinal permeability in autistic children compared with healthy siblings and non-related controls( Reference Robertson, Sigalet and Holst 67 ). The same test (a differential sugar-absorption test) was used as well as measuring glucagon-like peptide-2, an enteroendocrine molecule that is released from the GI tract in response to nutrients, which has been found to reduce intestinal permeability( Reference Drucker 68 , Reference Sigalet, Bawazir and Martin 69 ). No differences were observed in the fourteen ASD patients compared with eight siblings and seven non-related controls. However, the small size of this pilot study may not have been sufficient to show a statistically significant difference between the two groups. Therefore, further research is warranted to establish whether there is a true higher incidence of enhanced intestinal permeability in ASD.
Metabolic abnormalities
Several metabolic abnormalities have been found in autistic children. These include, amongst others, defects in methylation, oxidative stress( Reference James, Cutler and Melnyk 70 , Reference James, Melnyk and Fuchs 71 ), and disturbed concentration of amino acids in plasma( Reference Tirouvanziam, Obukhanych and Laval 72 ). Also, abnormal sulfur metabolism( Reference Tirouvanziam, Obukhanych and Laval 72 – Reference Ryaskin 74 ), lower concentrations of mammalian–microbial co-metabolites and a more active nicotinic acid metabolism( Reference Yap, Angley and Veselkov 75 ), have been found in autistic individuals. Below, we will review this in more detail.
Methylation, sulfation and oxidative stress
Several studies have reported lower baseline plasma concentrations of methionine, S-adenosylmethionine, homocysteine, cystathionine, cysteine, and total glutathione and significantly higher concentrations of S-adenosylhomocysteine, adenosine and oxidised glutathione in autistic children( Reference James, Cutler and Melnyk 70 , Reference James, Melnyk and Fuchs 71 ). These findings implicate impaired methylation and oxidative stress in autistic individuals. Geier et al. ( Reference Geier, Kern and Garver 73 ) found that patients with ASD show decreased trans-sulfuration metabolites and reduced sulfate concentrations. The same group of researchers( Reference Geier, Kern and Garver 76 ) found significantly decreased plasma reduced glutathione, cysteine, taurine, sulfate, and increased oxidised glutathione in the plasma of autistic children relative to age-matched neurotypical controls. Other research also found a decreased ability to form sulfated metabolites( Reference Alberti, Pirrone and Elia 77 ) and lower plasma sulfation products( Reference Strous, Golubchik and Maayan 78 ) in autistic individuals. The inability of autistic individuals to properly respond to toxins may be partially due to an undersupply of sulfate substrate for the sulfotransferases, which results in impaired sulfur-dependent detoxification pathways. Sulfate substrate is presumably produced by the sulfoxidation of cysteine( Reference McFadden 79 ), which was found to be decreased( Reference Geier, Kern and Garver 73 ).
Geier et al. ( Reference Geier, Kern and Garver 73 ) concluded that the abnormal (oxidised) glutathione levels result in a disturbance of the reduced/oxidised glutathione redox equilibrium. This may subsequently affect processes in which this equilibrium is involved, such as nitrogen and oxygen free radical scavengers, protein redox status and enzyme activity, cell membrane integrity, signal transduction and gene expression. Furthermore, increased levels of oxidised glutathione in plasma were found to be correlated with increased levels of Hg-associated urinary porphyrins. Geier et al. ( Reference Geier, Kern and Garver 73 ) suggested that increased levels of oxidised glutathione may contribute to abnormal trans-sulfuration and consistently result, in co-occurrence with low glutathione levels and increased oxidative stress, in a higher risk of infections and inflammation in autistic children( Reference Geier, Kern and Garver 73 ).
These systemic impairments of sulfation in ASD patients could theoretically threaten the stability of the catecholamine transmitter systems, the integrity of the gut lining, and heighten vulnerability to food-borne or pollutant xenobiotic overload to tissues( Reference Alberti, Pirrone and Elia 77 ).
Glutamate, glutamine and other amino acids
An altered urinary amino acid excretion has been frequently found in autistic children compared with age-matched controls( Reference Evans, Dunstan and Rothkirch 80 ). In children with ASD, Pangborn & Baker( Reference Pangborn and Baker 81 ) found very low levels of some amino acids on urinalysis, such as taurine (in 62 % of subjects), lysine (in 59 %), phenylalanine (in 54 %) and methionine (in 51 %). The researchers also found, to a lesser extent, below-normal levels of tyrosine, leucine, glutamine, valine and asparagine( Reference Pangborn and Baker 81 ). In the plasma of autistic children( Reference Aldred, Moore and Fitzgerald 82 ), however, increased levels of glutamic acid, phenylalanine, asparagine, tyrosine, alanine and lysine were found. The parents and siblings of these patients had these increased plasma levels as well, compared with non-autistic and non-related controls. The authors suggest that this may reflect a reduced capacity to remove amino acids from the system, or, without supplying evidence, a relative permeable blood-brain barrier. An altered plasma level of amino acids could be related to nutritional intake, but the sampling was done at home, thus under different (nutritional) conditions between the families. Raised plasma levels of phenylalanine and tyrosine levels may theoretically lead to higher concentrations of catecholamines such as dopamine and noradrenaline( Reference Aldred, Moore and Fitzgerald 82 ). It is hypothesised that elevated dopaminergic( Reference Lam, Aman and Arnold 83 ) and (nor)adrenergic( Reference Young, Kavanagh and Anderson 84 ) functions could be involved in the development of autism. However, the existing research does not provide strong support for these mechanisms due to inconsistent results and lack of methodological quality( Reference Lam, Aman and Arnold 83 ).
Also, the balance between glutamic acid and glutamine, in plasma, was found to be different in ASD patients( Reference Shimmura, Suda and Tsuchiya 85 ) and their parents( Reference Aldred, Moore and Fitzgerald 82 ) compared with controls. Glutamic acid is an excitatory neurotransmitter crucial to neuronal plasticity and the maintenance of cognitive functioning( Reference Shimmura, Suda and Tsuchiya 85 ), which is normally removed from the synapse after a receptor has been activated and is carried by transporter proteins to astrocytes( Reference Kojima, Nakamura and Nidaira 86 ). Here, it is stored as glutamine via glutamine synthetase, and later transported back to the presynaptic neurons and reconverted to glutamate, via glutaminase. Glutamine is required for the metabolism of enterocytes, and lower levels may thus have a deleterious effect on gut function, or a disturbed microbiota composition( Reference Aldred, Moore and Fitzgerald 82 ). Glutamine synthase, which converts glutamate into glutamine( Reference Ortinski, Dong and Mungenast 87 ), is down-regulated by activated astrocytes. Glutaminase, which converts glutamine into glutamate( Reference Pais, Figueiredo and Peixoto 88 ), is activated by necrotic neurons in microglia. Activated astrocytes and necrotic neurons in microglia characterise gliosis, which is found to be increased in autistic brains( Reference Laurence and Fatemi 89 ).
Thus far, most observations made have led to speculations about possible metabolic disturbances. However, it is also suggested that amino acid deficiencies found in children with autism are due to poor protein nutrition and food selectivity( Reference Bandini, Anderson and Curtin 90 ). ASD children who were on restricted diets had an increased prevalence of essential amino acid deficiencies and lower plasma levels of essential acids, including tyrosine and tryptophan, than both controls and ASD children on unrestricted diets( Reference Arnold, Hyman and Mooney 91 ). Thus, findings of abnormal levels of several amino acids might be attributable to poor nutrition secondary to food selectivity( Reference Arnold, Hyman and Mooney 91 ), instead of actual metabolic abnormalities. However, the multiple findings indicating metabolic difficulties suggest that food selectivity may not be the only cause of changed amino acid levels in autistic children.
Carbohydrate digestion and absorption
Intestinal bacteria encode the enzymes glycoside hydrolase and polysaccharide lyase, which are absent in humans, necessary for the fermentation of poly- and oligosaccharides. These bacteria produce SCFA as endproducts of polysaccharide fermentation. These SCFA serve as energy substrates for colonocytes, modulate colonic pH, regulate colonic cell proliferation and differentiation, and contribute to hepatic gluconeogenesis and cholesterol synthesis( Reference Jacobs, Gaudier and Duynhoven 92 , Reference Wong and Jenkins 93 ).
Recently, intestinal biopsies (ileum) from children with autism and GI problems showed a deficiency of ileal transcripts encoding disaccharidases and hexose transporters, indicative of impairment of the primary pathway for carbohydrate digestion and transport in enterocytes. Since the conditions that impact on transcription are not specific for a specific segment but rather for the whole absorptive intestine, it is reasonable to assume that similar effects will also be present in the duodenum and jejunum. Indeed, one other study( Reference Horvath, Papadimitriou and Rabsztyn 27 ) reported low enzyme activities in 58 % of children with ASD in the duodenum. Reduced carbohydrate digestion and absorption can lead to accumulation of saccharides in the intestinal lumen, resulting in osmotic diarrhoea, bloating and flatulence( Reference Williams, Hornig and Buie 94 ). The expression levels of disaccharidases and hexose transporters may be controlled, in part, by the transcription factor CDX2 (caudal type homeobox 2). In autistic children with GI symptoms, 86·7 % had CDX2 levels below the 50th percentile of control children with GI symptoms. Moreover, 46·7 % of autistic children with GI symptoms had at least a two-fold decrease in mean CDX2 expression relative to the control children with GI symptoms( Reference Williams, Hornig and Buie 94 ). In addition, an altered gut microbiota was observed, including increased levels of caecal Firmicutes, especially clostridia, and a higher caecal Firmicutes:Bacteroidetes ratio( Reference Williams, Hornig and Buie 94 ). This dysbiosis of the mucoepithelium was associated with the deficiencies in host disaccharidase and hexose transporter messenger RNA expression.
In conclusion, abnormalities in carbohydrate digestion and absorption could possibly explain some of the GI problems observed in a subset of ASD patients, although their role in the neurological and behavioural problems remains uncertain.
Intestinal inflammation
Chronic inflammation in the gut can damage the epithelial cell layer( Reference Mowat 95 ). When present, this may explain the increased intestinal permeability found in ASD patients. In this respect, intestinal biopsies among fifty-two regressive autistic children revealed significantly increased CD3+ and CD3+CD8+ in the epithelium as well as CD3+ in the lamina propria compared with developmentally normal non-inflamed control groups, reaching levels similar to inflamed controls( Reference Ashwood, Anthony and Pellicer 96 ). Up-regulation of pro-inflammatory cytokines in the intestinal mucosa of autistic children with GI symptoms has also been reported( Reference Ashwood and Wakefield 97 , Reference Ashwood, Krakowiak and Hertz-Picciotto 98 ). Additionally, increasing cytokine levels were associated with more impaired communication and aberrant behaviours( Reference Ashwood, Krakowiak and Hertz-Picciotto 98 ). However, in another study( Reference DeFelice, Ruchelli and Markowitz 99 ), pro-inflammatory cytokines IL-6, IL-8 and IL-1β were not found to be elevated in the mucosa. In addition, two independent markers of inflammatory reactions in the gut, i.e. rectal NO and faecal calprotectin, were measured in twenty-four children with autism. As in only two of the children the level of one of these markers was increased, the investigators were unable to disclose evidence of a link between the autistic disorder and active intestinal inflammation( Reference Fernell, Fagerberg and Hellström 100 ). Thus, controversial results exist regarding a causal role of GI inflammation in the aetiology and/or behavioural aspects of autism.
Food allergies
In food allergy, presentation of food antigen leads to a response by T cells and subsequently to an initiation of a food-mediated immune response( Reference Eigenmann 101 ). Parental reports have shown a significantly greater incidence of food allergy in ASD patients compared with healthy controls( Reference Gurney, McPheeters and Davis 102 ). Lucarelli et al. ( Reference Lucarelli, Frediani and Zingoni 103 ) found in 1995 that an oral challenge with cows’ milk protein led to worsening of some of the behavioural symptoms of autistic children, and that these children express significantly higher serum levels of IgA, IgG and IgM for casein and IgA for lactalbumin and β-lactoglobulin compared with healthy controls. Recently, Sabra et al. ( Reference Sabra, Bellanti and Hartmann 104 ) hypothesised that food allergy is the pivotal causative factor that produces lesions in the ileum that consist of enlarged lymphoid nodules containing large collections of lymphocytes in the GI lymphoid tissues adjacent to Peyer's patches. These GI lesions would allow the entry of food antigens across the inflamed mucosa of the bowel and elicit an inflammatory response in the GI tract( Reference Sabra, Bellanti and Hartmann 104 ). They found LNH, reactive lymphoid follicular hyperplasia and chronic inflammation in twelve children with attention-deficit/hyperactivity disorder, autism, anorexia and/or migraine. Th1-associated cytokines were found to be decreased compared with control values, which, together with a predominance of CD4+ cells, support an immunological basis for non-IgE-mediated food allergy (NFA) in this group( Reference Sabra, Bellanti and Hartmann 104 ).
Another research group( Reference Jyonouchi 105 ) also supports the hypothesis of an existence of NFA in autistic children. They found more TNF-α and IL-12 in peripheral blood mononuclear cells of children with autism than controls, when stimulated with cows' milk protein, β-lactoglobulin and α-lactoalbumin, irrespective of objective GI symptoms( Reference Jyonouchi, Geng and Ruby 106 ). However, NFA may play a lesser role in GI symptoms in older children with autism, as most children probably outgrow NFA during the first 2 years of life with maturation of the gut immune system and the establishment of oral tolerance( Reference Hwang, Sohn and Kim 107 ). Concluding, NFA could partially explain GI symptoms and the suggested benefits of dietary interventions such as the GFCF diet in some autistic children under the age of 2 years.
Nutritional interventions
As introduced, some treatment strategies of autism are specifically aimed at dietary measures to improve gut health. The most important are described below.
Gluten- and milk protein-free diets
A regularly proposed treatment to reduce food-related effects in autism is a GFCF diet. Many non-peer-reviewed articles, books( Reference LeBreton 108 ) and websites support and encourage the application of this diet. Previous research among 284 autistic children indicates that approximately 15·5 % of autistic children in the USA use this diet as a complementary treatment( Reference Levy, Mandell and Merhar 109 ). Consequently, there have been many reports on the role of a GFCF diet on alleviating several symptoms of autistic individuals( Reference Whiteley, Rodgers and Savery 19 , Reference Bird, Russo and Cataldo 110 – Reference Knivsberg, Reichelt and Hoien 116 ) (however, not all of sufficient methodological quality; see below). Significant improvements have been noted within psychological and behavioural categories in vocal and non-vocal communication, attention and concentration, episodes of aggressiveness, affection, motor skills, sleeping patterns, displaying of routines and rituals, anxiety, empathy and responses to learning( Reference Whiteley, Rodgers and Savery 19 , Reference Patel and Curtis 111 , Reference Cade, Privette and Fregly 112 , Reference Knivsberg, Reichelt and Hoien 115 , Reference Knivsberg, Reichelt and Hoien 116 ). Moreover, reintroduction of dietary gluten elicited a worsening of behaviours in areas of hyperactivity and impulsivity, stereotyped behaviours, aggression and language and communication skills( Reference Whiteley, Rodgers and Savery 19 ). A slight initial worsening in behaviour after introduction of the GFCF diet was also noted, which was suggested to be comparable with the withdrawal behaviours exhibited by opioid addicts on the removal of opioids( Reference Whiteley, Rodgers and Savery 19 ). Changes in physical and physiological areas were measured in some studies as well. One patient showed abnormal peptides not found in controls, including β-casomorphin (BCM), α-gliadin, dermorphin, deltorphin I and II, and morphine-modulating neuropeptide( Reference Shanahan, Venturini and Daiss 117 ). Some of these have also been observed in other studies( Reference Moore, Jessop and Osborne 57 , Reference Cade, Privette and Fregly 112 , Reference Reichelt, Knivsberg and Lind 118 ).
Nevertheless, when critically reviewing these studies it appears that most of these lack sufficient methodological quality( Reference Mulloy, Lang and O'Reilly 119 , Reference Christison and Ivany 120 ), and a short overview of the trials that have been conducted, to our knowledge, is shown in Table 1.
Table 1 Studies assessing the effect of gluten and casein related dietary interventions on autism spectrum disorder (ASD) symptoms

m, Male; f, female; GFCF, gluten-free, casein-free; PND, percent non-overlapping data; UPL, urinary peptide level; GSRS, Gastrointestinal Symptoms Rating Scale; CARS, Childhood Autism Rating Scale; ITPA, Illinois Test of Psycholinguistic Ability; DIPAB, Diagnose of Psykotisk Adfœrd hos Børn (Diagnosis of Psychotic Behaviour in Children); GF, gluten-free; C-Raven, C-Raven Progressive Matrices; BSE, Behaviour Summarized Evaluation; ADHD, attention-deficit/hyperactivity disorder; K-ABC, Kaufmann Assessment Battery for Children; ADOS-G, Autism Diagnostic Observation Schedule-Generic; VABS, Vineland Adaptive Behavior Scale; GARS, Gilliam Autism Rating Scale.
All the studies except for one lack a control group, and therefore have a high placebo response rate, due to high levels of parental and/or clinician expectancy, a presumed lack of side effects and the degree of parental effort and resources that are invested in the treatment. The outcomes of the three studies with the highest experimental validity are negative, and showed no statistically significant difference between the control and intervention groups after implementing the GFCF diet( Reference Bird, Russo and Cataldo 110 , Reference Elder, Shanker and Shuster 113 , Reference Irvin 114 ). Also, other studies showed no changes in urinary peptide levels of gluten, casein and IAG( Reference Parracho, Bingham and Gibson 22 , Reference Wright, Brzozowski and Calvert 121 ). Moreover, exclusion of casein from the diet was found to decrease intake of dairy foods as well as micronutrients associated with dairy foods. Although supplementation improved many of the inadequacies seen in autistic children on a GFCF diet, nutrients important for bone health such as Ca and P were still inadequate( Reference Mantos, Ha and Caine-Bish 122 ).
The introduction of the GFCF diet is derived from the opioid excess theory of autism. Opioids are chemical substances that have a morphine-like activity in the body, and act by binding to opioid μ-, δ- and κ-receptors. These receptors are located principally in the central nervous system as well as the GI tract and activation elicits adenylatecyclase inhibition, K+ channel activation, or Ca2+ inactivation( Reference Teschemacher 123 ). The opioid excess theory states that children with autism are symptomatic due to excess of peptides, derived endogenously, as well as exogenously from incomplete breakdown of certain foods, both possibly due to peptidase deficiencies. In particular, gluten-derived peptides from wheat and some other cereals and casein from milk and dairy products are suspected to be involved in autism( Reference Whiteley, Rodgers and Savery 19 , Reference Panksepp 124 , Reference Reichelt, Hole and Hamberger 125 ). Opioid-like molecules include, for example, α-gliadin, dermorphin, novel autism peptide I and III( Reference Shanahan, Venturini and Daiss 117 ) and BCM( Reference Sun, Cade and Fregly 40 , Reference Brantl, Teschemacher and Bläsig 126 ). The resulting peptides may have direct opioid action or may act as ligands to the enzymes that would break down the endogenous opioids( Reference Reichelt, Hole and Hamberger 125 ). A few studies have reported indirect supportive results for this hypothesis, namely an abnormal intestinal permeability in autistic subjects, observed using a differential sugar-absorption test( Reference D'Eufemia, Celli and Finocchiaro 41 , Reference de Magistris, Familiari and Pascotto 28 ) and an abnormal (high) peptide content in the urine of autistic children( Reference Moore, Jessop and Osborne 57 , Reference Cade, Privette and Fregly 112 , Reference Shanahan, Venturini and Daiss 117 , Reference Reichelt, Knivsberg and Lind 118 , Reference Nelson, Grether and Croen 127 ). Through the immune system, an alternative account of this issue is proposed. It was recently discussed that microglial in the brain can be activated by infection and inflammation, causing behavioural changes( Reference Teeling and Perry 128 ).
Cows' milk-derived BCM, which is thought to have bioactive properties, is extensively studied for its potential role in several human diseases. It is found in bovine as well as mother's milk( Reference Kaminski, Cieslinska and Kostyra 129 ) and acts as a substrate for dipeptidyl peptidase 4 (DPP-IV), which is a cell-surface protease present on the brush border of the intestine, kidney and liver( Reference Kreil, Umbach and Brantl 130 ). A defect in DPP-IV, a brush-border peptidase which normally digests dietary opioids( Reference Lambeir, Durinx and Scharpé 131 ), could cause the presence of opioid peptides such as BCM in the urine of children with autism( Reference Shanahan, Venturini and Daiss 117 ). In a study by Kost et al. ( Reference Kost, Sokolov and Kurasova 132 ), ninety infants, of which thirty-seven were fed with mother's milk and fifty-three were fed with formula containing cows' milk, were investigated on levels of human and bovine BCM. Elevated basal bovine BCM was found in blood plasma of cows' milk-fed infants who showed delay in psychomotor development and elevated muscle tone. The authors therefore suggested the deterioration of bovine BCM elimination as a risk factor for delay in psychomotor development and autism. However, other studies, reviewed by Hunter et al. ( Reference Hunter, O'Hare and Herron 133 ), could not find defects or abnormalities in DPP-IV in autistic subjects. Lacking direct evidence, however, this theory remains speculative.
Dietary pre- and probiotic supplementation
The symptoms of inflammatory bowel disease and irritable bowel syndrome are quite similar to those of the GI symptoms in ASD patients, namely abdominal pain or discomfort (which sometimes lessens upon defecation), and are associated with changing stool frequency and/or changing stool consistency( Reference Longstreth, Thompson and Chey 134 ). Due to these similarities and the established role of probiotics in the treatment of inflammatory bowel disease (see below), a possible treatment of autism with probiotics has been proposed. Since autistic children also frequently suffer from other GI disorders, studies have been undertaken to understand the composition of gut microbiota in autistic children and to modify the gut microbiota and its metabolism favourably when required. In this respect, some bacteria are believed to be harmful through the production of harmful metabolites, and some bacteria are thought to be health-promoting, such as the bifidobacteria and lactobacilli( Reference Bingham and Bingham 135 ). The latter are believed to provide protection against infection in the gut and to help in maintaining an efficient barrier function and a healthy immune function( Reference Bingham and Bingham 135 ).
Probiotics are live micro-organisms, which when administered in adequate amounts confer a health benefit on the host( 136 ). They are supposed to give relief from lactose maldigestion( Reference Marteau, Flourie and Pochart 137 , Reference Sanders 138 ), reducing the related episodes of diarrhoea( Reference Oksanen, Salminen and Saxelin 139 , Reference Saavedra, Bauman and Oung 140 ). Additionally, probiotics are suggested to help reduce risk factors associated with inflammatory bowel disease( Reference Guslandi, Mezzi and Sorghi 141 , Reference Rembacken, Snelling and Hawkey 142 ), colorectal cancer( Reference Burns and Rowland 143 ) and impaired gut-associated immune responses( Reference Marteau, Minekus and Havenaar 144 – Reference Isolauri, Sütas and Kankaanpää 146 ).
In a double-blind, placebo-controlled study by Parracho et al. ( Reference Parracho, Gibson and Knott 147 ), Lactobacillus plantarum feeding of children with autism resulted in significant increased levels of the beneficial bacteria lactobacilli and enterococci, and a significant reduction of a cluster of Clostridium, compared with the placebo group. Through a 12-week study, the probiotic feeding resulted in reduced GI problems and, more importantly, in improved behaviour scores compared with baseline. In this respect, it is noteworthy that, during another double-blind, cross-over study, addressing the effects of the probiotic L. plantarum on autism failed during the changing of treatments in the cross-over period, because parents (who were blinded for the intervention) of children treated with the actual probiotics refused to make the switch, as they wanted their autistic children to continue their improvement( Reference Thomson 148 ). Noted improvements were decreased levels of clostridia bacteria in the stools and a positive effect on mood and general behaviour, as described by parents. Since this can only be considered as anecdotal evidence, further well-controlled studies are warranted.
Another probiotic trial in autistic children was recently conducted by Kałużna-Czaplińska & Błaszczyk( Reference Kałużna-Czaplińska and Błaszczyk 149 ). Probiotic supplementation with L. acidophilus over 2 months led to a significant decrease in d-arabinitol and to a significant improvement in the ability to concentrate and carry out orders. d-Arabinitol is a metabolite of most pathogenic Candida species and its excretion in urine is elevated in autistic patients( Reference Kałużna-Czaplińska and Błaszczyk 149 ). Candida infections have been associated with autism previously( Reference Shaw, Kassen and Chaves 35 ). In a trial regarding the oral supplementation of vancomycin, followed by supplementation of a probiotic mixture of L. acidophilus, L. bulgaricus and Bifidobacterium bifidum (40 × 109 colony-forming units/ml), positive results were found in communication and behaviour( Reference Sandler, Finegold and Bolte 150 ). However, the effects were attributed to vancomycin, the subject of study, and no attention was given to the possible contribution of the probiotic mixture.
Unfortunately, these studies were not of sufficient methodological quality due to the absence of control groups, multiple treatments at once and/or small sample sizes, as shown in an overview in Table 2.
Table 2 Studies assessing the effect of probiotic interventions on autism spectrum disorder (ASD) symptoms
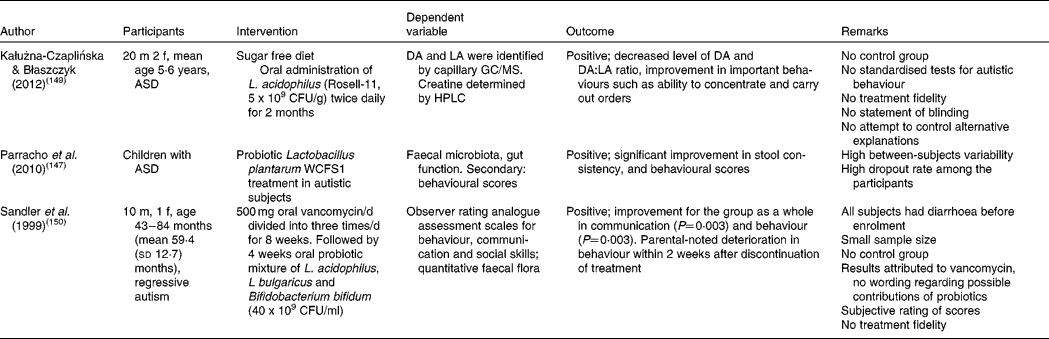
m, Male; f, female; CFU, colony-forming unit; DA, d-arabinitol; LA, l-arabinitol.
Prebiotics are non-digestible food ingredients that have a beneficial effect through their selective metabolism in the intestinal tract. A reason for a potential influence of prebiotics on the treatment of symptomatology of autism concerns especially the selective stimulation of growth of lactobacilli and bifidobacteria and the production of SCFA that have an influence on gut energy metabolism, gut barrier function, water fluxes and motility( Reference Gibson and Roberfroid 151 ). However, no systematic studies have been conducted so far.
Vitamin supplementation
Autistic children often experience significant eating difficulties, specific food selectivity( Reference Arnold, Hyman and Mooney 91 , Reference Williams, Dalrymple and Neal 152 , Reference Herndon, DiGuiseppi and Johnson 153 ), poor digestion( Reference Williams, Hornig and Buie 94 ), inflammatory conditions in the gut( Reference Jyonouchi, Sun and Le 154 , Reference Bauer, Kerr and Patterson 155 ) and reduced levels of vitamin-producing microbiota in the intestines( Reference Wang, Christophersen and Sorich 65 , Reference Guarner and Malagelada 156 ). These factors could lead to a poor nutritional status( Reference Kidd 157 ). A survey among physicians showed that multivitamin supplements are among the most widely recommended medical interventions for autism, and are recommended for children with autism by almost half of the interviewed physicians( Reference Golnik and Ireland 158 ). As such, a plethora of research has been published in respect of which vitamins, combined with a range of minerals, have been supplemented with various outcomes.
The available studies involving vitamin/mineral supplementation of autistic individuals generally lead to slightly promising results. Nutritional high-dose supplementation with ascorbic acid resulted in a statistically significant reduction in autism severity( Reference Dolske, Spollen and McKay 159 ). There have been many double-blind, placebo-controlled studies of very high doses of vitamin B6 with Mg, and all but one showed positive behavioural improvements( Reference Adams, George and Audhya 25 ). However, the studies were limited by lack of sufficient methodological quality due to small sample size and the use of assessment tools of limited validity( Reference Adams, George and Audhya 25 ).
Two small pilot studies involving multivitamin/mineral supplementation, although of limited quality, showed positive preliminary results( Reference Dolske, Spollen and McKay 159 , Reference Kaplan, Fisher and Crawford 160 ). Recently, 141 children and adults with autism enrolled in a randomised, double-blind, placebo-controlled vitamin/mineral supplementation study( Reference Adams, Audhya and McDonough-Means 23 ). The autistic subjects improved in levels of vitamins, minerals, and biomarkers of sulfation (higher levels of free and total sulfate), methylation (higher levels of S-adenosylmethionine and lower levels of uridine), glutathione (higher levels of reduced glutathione) and oxidative stress (higher reduced:oxidised glutathione ratio and lower levels of nitrotyrosine), compared with a control group. Significant improvements were also noted in behavioural problems, including hyperactivity, tantrumming (display of disruption, non-compliance, instigation and/or interruption frequently accompanied by aggression( Reference Duda and Heindel 161 )) and receptive language. In addition, plasma and whole-blood levels of several vitamins and minerals, plasma levels of ATP and coenzyme Q10, and erythrocyte levels of NADH and NADPH increased from below normal to normal levels after supplementation( Reference Adams, Audhya and McDonough-Means 23 ). The combined effects of vitamin B6 and Mg were systematically reviewed( Reference Nye and Brice 162 ), and it was concluded that the data were insufficient to use such supplementation as a treatment for ASD.
Summarising considerations
In conclusion, the exact possible biological causes and symptomatology of the autistic spectrum are still poorly understood. A tremendous amount of theories and hypotheses exist regarding this subject, even when only looking at metabolic aspects. It might well be that not all possible circulating hypotheses are described in the present paper. It can at least be stated firmly that there is considerable evidence that the gut–brain axis is involved in the aetiology of autism.
There is convincing evidence that a genetic predisposition, strengthened by early exposure to environmental agents in a vulnerable period, provides an unstable base allowing a possible development of autism. Factors such as nutrition, infections and the use of antibiotics might trigger this base and lead to the development of autism. However, there are many possible mechanisms, not all completely understood, by which this is possible and many contradicting results from an extensive amount of studies make it even harder for parents and children to find sufficient help in dealing with this disease. There is need for further research to improve diagnosing and treatment for different subsets of autism. Maybe not the severity of symptoms are diagnostic criteria for the different groups (classic autism, Asperger's syndrome, regressive autism, etc.), but the origin of symptoms (GI problems, presence of vitamin deficiencies, brain abnormalities, etc.) will determine the classification in the next Diagnostic and Statistical Manual of Mental Disorders (DSM), which would aid in finding the right treatment for the right individual.
Data supporting the presence of GI problems in ASD patients are convincing, at least for some kind of subset of children who experience a worsening of autistic symptoms along with GI symptoms. Furthermore, the increased incidence of autism might be related to exposure to Clostridium spores in the environment. In addition, cases of increased intestinal permeability were observed, although the evidence so far has been inconclusive. Multiple findings indicating metabolic abnormalities suggest that these are present in ASD patients, and that these abnormalities may partially cause the observed change in amino acid levels and levels of inflammation in these patients. Abnormalities in carbohydrate digestion and absorption could possibly explain some of the GI distress observed in a subset of ASD patients, although their role in the neurological and behavioural problems remains uncertain. Thus, a subset of ASD patients displays measurable changes in GI symptoms, composition of gut microbiota, excretion of metabolites, intestinal permeability and metabolism of several compounds. Feeding selectivity problems frequently detected in ASD patients can be a major cause of these abnormalities, and should certainly be taken into account when investigating other causes. However, this does not change the importance of investigating the effect of these abnormalities on autistic symptoms, and methods to alleviate both GI and autistic symptoms.
Moreover, we feel that additional trials involving gluten-free diets and casein-free diets should be conducted. Current research on the hypothesised relationship between an improved gut health and a reduction of symptoms in some patients is mostly lacking sufficient methodological quality. The few trials involving pre- and probiotic, and multivitamin supplementation have been conducted with contradictive but partly promising results in reducing symptomatology. Results from these trials show the importance of conducting further research in this area. It is clear, however, that children with autism need personal support and advice, because every child experiences slightly different symptoms.
Although there is substantial evidence that the GI tract and the gut–brain axis have a central role in autism, further studies are required to understand the aetiology and the mechanisms by which these factors act, and to understand the possible cause–effect relationship between food choice and autism.
Acknowledgements
This research received no specific grant from any funding agency in the public, commercial or not-for-profit sectors.
Both M. v. d. S. and F. B. initiated the literature search. M. v. d. S. and F. B. conducted the literature research and analysed the data; M. v. d. S., V. v. B. and F. B. wrote the paper, V. v. B. was responsible for final editing while M. v. d. S. was primarily responsible for final content. All authors read and approved the final manuscript.
The authors declare no conflicts of interest and had no interaction with the food and beverage industry with respect to the contents of this article.