Introduction
The Younger Dryas cold period (c.12.9–11.7 ka cal BP) at the end of the Weichselian was caused by abrupt changes in the thermohaline ocean circulation (Bakke et al., Reference Bakke, Lie, Heegaard, Dokken, Haug, Birks, Dulski and Nilsen2009) in combination with moderate negative radiative forcing and an altered atmospheric circulation (Renssen et al., Reference Renssen, Mairesse and Goosse2015). The cooling resulted in an indirect response of the aeolian environment with enhanced aeolian deposition in two different ways. First, the Younger Dryas cooling caused a reduction of the vegetation cover (Van Geel et al., Reference Van Geel, Coope and Van der Hammen1989; Hoek, Reference Hoek1997a, Reference Hoek2000; Bos et al., Reference Bos, Verbruggen, Engels and Crombé2013) that resulted in local deflation and deposition of aeolian sand (Younger Coversand II, cf. Van der Hammen & Wijmstra, Reference Van der Hammen and Wijmstra1971). Second, the cooling caused a fluvial system change from meandering to a braided river style in several European lowland river systems, because of a more nival discharge regime and increased stream power, due to lower temperatures, lower evapotranspiration and deeper seasonal frost (Vandenberghe et al., Reference Vandenberghe, Kasse, Bohncke and Kozarski1994; Kasse, Reference Kasse1995a; Kasse et al., Reference Kasse1995, Reference Kasse, Hoek, Bohncke, Konert, Weijers, Cassee and Van der Zee2005, Reference Kasse, Van Balen, Bohncke, Wallinga and Vreugdenhil2017; Litt et al., Reference Litt, Schmincke and Kromer2003; Woolderink et al., Reference Woolderink, Kasse, Cohen, Hoek and Van Balen2018). The development of wide braid plains enabled deflation from the floodplain during low discharge and led to the formation of aeolian source-bordering river dunes (Vandenberghe, Reference Vandenberghe1991; Kasse, Reference Kasse, Troelstra, Van Hinte and Ganssen1995b, Reference Kasse2002).
Source-bordering dunes were mapped and described frequently, especially in the Rhine–Meuse area (Pons, Reference Pons1957; Bohncke et al., Reference Bohncke, Vandenberghe and Huijzer1993; Kasse et al., Reference Kasse1995; Berendsen et al., Reference Berendsen, Hoek and Schorn1995; Hoek et al., Reference Hoek2017; Woolderink et al., Reference Woolderink, Kasse, Cohen, Hoek and Van Balen2018). However, along the Scheldt, source-bordering dunes have been described less frequently (Mijs, Reference Mijs1974; Kasse, Reference Kasse1988, Reference Kasse1999; Kiden, Reference Kiden, Starkel, Gregory and Thornes2006). Upstream of the Rhine–Meuse terrace intersection point, the braid-plain source areas of these source-bordering dunes are partly preserved by later river incision and terrace formation at the Younger Dryas to Holocene transition. They are still visible in the landscape as terraces (Pons, Reference Pons1957: terrace X; Berendsen et al., Reference Berendsen, Hoek and Schorn1995: generation 4; Kasse et al., Reference Kasse1995: level 5). However, downstream of the terrace intersection, the Younger Dryas terrace and associated river dunes drowned by sea-level rise and were covered by Holocene deposits (Verbraeck, Reference Verbraeck1983; Hijma et al., Reference Hijma, Cohen, Hoffmann, Van der Spek and Stouthamer2009; Koster, Reference Koster2020). In the Scheldt Valley of the SW Netherlands, west of the Brabantse Wal escarpment, up to 15 m thick Holocene deposits occur (Vos & Van Heeringen, Reference Vos and Van Heeringen1997; Kiden, Reference Kiden, Starkel, Gregory and Thornes2006) and therefore the source and extent of the Younger Dryas aeolian dunes in this area are less well known.
River dunes are generally assigned to the Younger Dryas period, but absolute age control is rather limited and often indirect by radiocarbon dating of under- and overlying peat layers and biochronostratigraphic evidence. The top of organic beds below river dunes in the Meuse valley dates to the end of the Allerød or first part of the Younger Dryas (Bohncke et al., Reference Bohncke, Vandenberghe and Huijzer1993; Kasse et al., Reference Kasse1995). Palynologically, this coincides with a distinct drop in values of pine (Pinus) in favour of herbaceous taxa, indicating that the vegetation became more open (Hoek & Bohncke, Reference Bohncke2002). Optically stimulated luminescence enables direct dating of the aeolian sand and revealed a Younger Dryas age of dune formation along the Scheldt (Vandenberghe et al., Reference Vandenberghe2004; Bogemans & Vandenberghe, Reference Bogemans and Vandenberghe2011; Crombé et al., Reference Crombé, Cruz, De Graeve, De Grave, De Maeyer, Halbrucker, Teetaert, Vandenberghe and Vandendriessche2018). The Younger Dryas river dunes were modulated into drift-sand relief in the late Holocene (Schwan, Reference Schwan1991; Kasse & Aalbersberg, Reference Kasse and Aalbersberg2019).
Younger Dryas wind direction was derived mostly from large-scale aeolian landforms, e.g. based on the orientation of parabolic dunes (Mijs, Reference Mijs1974; Kasse, Reference Kasse, Troelstra, Van Hinte and Ganssen1995b; Isarin et al., Reference Isarin, Renssen and Koster1997). This large-scale morphology can be the result of long-term processes, giving a time-averaged wind direction of sand-transporting winds. On the other hand, it may reflect the wind direction in the last stage of dune formation before the dunes were stabilised. A west-southwesterly Younger Dryas wind direction was reconstructed for the Netherlands (Maarleveld, Reference Maarleveld1960; Isarin et al., Reference Isarin, Renssen and Koster1997). However, wind directions (and variability) derived from sedimentary structures are rarely reported to date. Horizontal bedding and low-angle cross bedding are the dominant bedding types in the dune sediments from which wind directions can hardly be defined. Rare dune slip-face crossbedding showed variable wind directions with a northerly (Kasse et al., Reference Kasse1995: site Bosscherheide) and west-northwesterly wind direction (dip direction/angle N120/30°) (Kasse & Aalbersberg, Reference Kasse and Aalbersberg2019: site Ossendrecht). Therefore, palaeowind direction during the formation of the river dunes is still uncertain. The reconstruction of palaeowind direction based on adhesion ripples, migrating in an upwind direction, has so far not been reported.
This paper aims to (1) reconstruct and explain the spatial distribution of Younger Dryas source-bordering dunes in the Scheldt River catchment; (2) determine vegetation development and age of river dune formation; and (3) reconstruct Younger Dryas wind direction based on dune morphology and sedimentary structures.
Geological and geomorphological setting
The investigated area is located in the Scheldt catchment in the southwestern Netherlands and northern Belgium (Fig. 1). The study area is situated west of the Roer Valley Rift System on the uplifting Campine block (Van Balen et al., Reference Van Balen, Houtgast and Cloetingh2005). Northward-dipping Neogene and Early Pleistocene marine and estuarine sand and clay deposits characterise the subsurface. Because of Quaternary uplift, the large-scale morphology is formed by erosion of the Scheldt and tributaries as witnessed by the presence of erosion-resisted highs such as the Boom Clay (Oligocene) cuesta south of Antwerp and Campine Clay (Early Pleistocene) microcuesta (Tavernier & De Moor, Reference Tavernier and De Moor1974). The Scheldt south of Ghent flows south-to-north in the direction of the former Flemish Valley. The Scheldt diverted to the east in the direction of Rupelmonde by the infill of the Flemish Valley, aeolian deposition and formation of the Maldegem–Stekene coversand ridge during the Late Pleniglacial and early Late Glacial (Heyse, Reference Heyse1983; Kiden, Reference Kiden, Starkel, Gregory and Thornes1991; Derese et al., Reference Derese, Vandenberghe, Zwertvaegher, Court-Picon, Crombé, Verniers and Van den haute2010; Bos et al., Reference Bos, Verbruggen, Engels and Crombé2013, Reference Bos, De Smedt, Demiddele, Hoek, Langohr, Marcelino, Van Asch, Van Damme, Van der Meeren, Verniers, Boeckx, Boudin, Court-Picon, Finke, Gelorini, Gobert, Heiri, Martens, Mostaert, Serbruyns, Van Strydonck and Crombé2017). At Rupelmonde the Scheldt turns sharply north in the direction of Antwerp and dissects the Boom Clay cuesta (Hoboken Gap) in the course of the Late Glacial, although it is likely that an initial depression, formed by smaller rivers from the south and east (Nete, Dijle, Rupel), was already present.
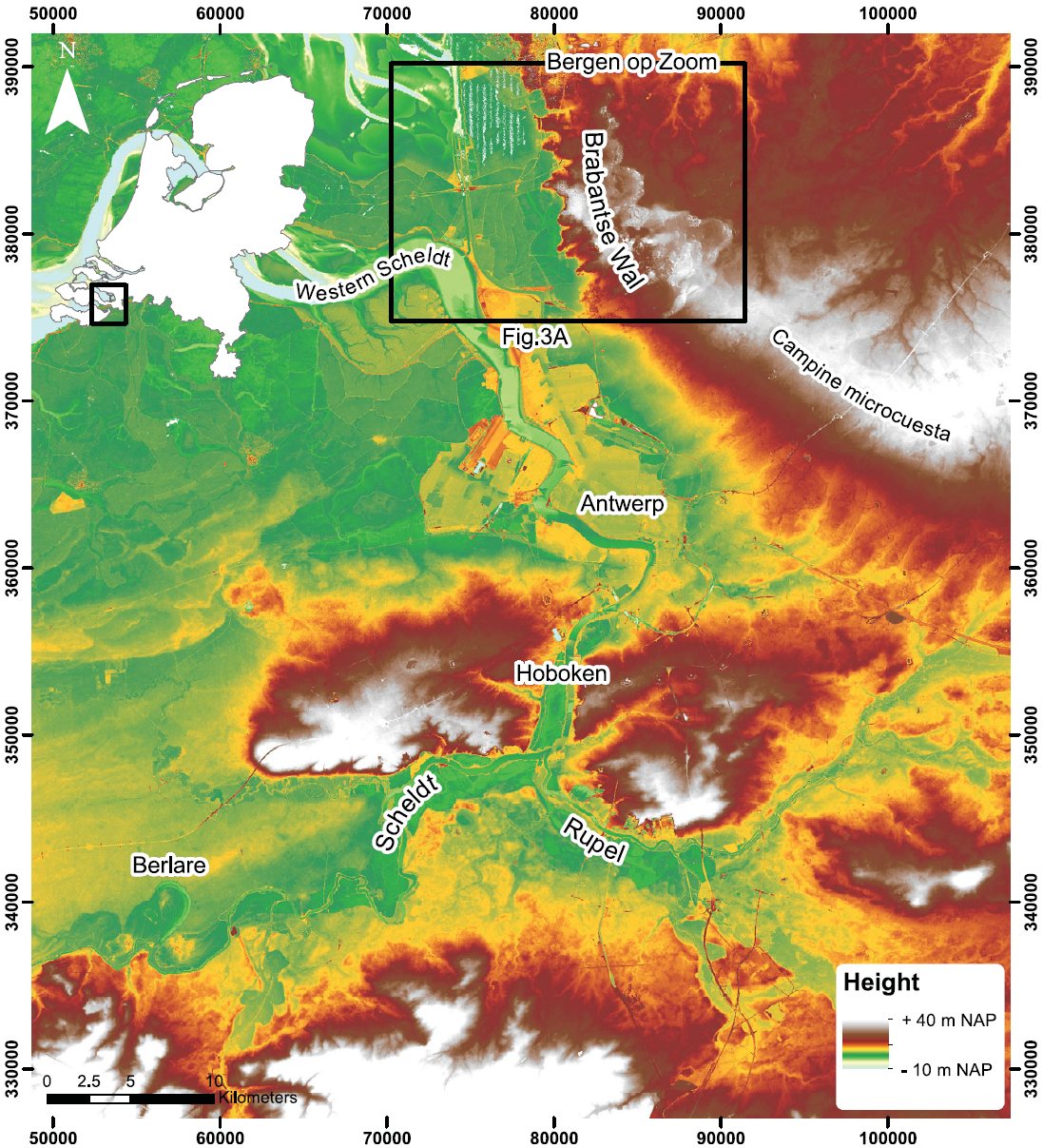
Fig. 1. Digital elevation model of the lower Scheldt catchment showing the geomorphological setting and location of the investigated area in the southern Netherlands and northern Belgium (black box).
North of Antwerp, the Scheldt follows the c.30 km long south–north escarpment of the Brabantse Wal that bends to the east at Kalmthout and can be continued into the Campine Clay microcuesta (Fig. 1). The escarpment and microcuesta formed by Middle and Late Pleistocene erosion by precursors of the Scheldt and its tributaries (Kasse, Reference Kasse1988; Westerhoff & Dobma, Reference Westerhoff and Dobma1995; Kiden, Reference Kiden, Starkel, Gregory and Thornes2006) and possibly by marine erosion during the Eemian. The escarpment separates the higher area in the east (Brabantse Wal) from the polder area in the west. The polders are at or slightly above sea level and were reclaimed in the last centuries.
The geology of the Brabantse Wal study area is characterised by Early Pleistocene estuarine fine sands and clay of the Waalre Formation of late Tiglian age (Kasse, Reference Kasse1988, Reference Kasse1990; Westerhoff et al., Reference Westerhoff, Kemna and Boenigk2008) (Fig. 2). These deposits are separated by a major unconformity from the overlying Late Pleistocene and Holocene coversand and dune deposits in the east (Boxtel Formation), at c.15 to 25 m above sea level (Vandenberghe et al., Reference Vandenberghe2004; Kasse & Aalbersberg, Reference Kasse and Aalbersberg2019). An up to 15 m thick, sea-level rise related, Holocene sequence is present in the west (Nieuwkoop and Naaldwijk Formations), completely filling the Late Weichselian Scheldt Valley (Vos & Van Heeringen, Reference Vos and Van Heeringen1997).

Fig. 2. Litho- and chronostratigraphy of the Brabantse Wal and polder area. Diffuse colours indicate diachronic boundaries between the units. OD is Older Dryas.
Previous age control on river dune formation in the region
Radiocarbon dates and calibrated ages in the text are referred to in years BP and years cal BP respectively (Table 1). In their Quaternary geological investigations of western Noord Brabant, Nelson and Van der Hammen (Reference Nelson and Van der Hammen1950) described the occurrence of organic deposits below the river dunes, at c.1 to 2 m below the surface, in three exposures near Hoogerheide and Ossendrecht on the Brabantse Wal (Fig. 3A). These organic sediments were interpreted, based on pollen analysis, to be formed during the Allerød period and Allerød – Younger Dryas transition (Fig. 2).
Table 1. Radiocarbon and OSL dates of Late Glacial and early Holocene organic deposits and sand units related to Younger Dryas river dune formation in the Netherlands. The dates are arranged in stratigraphic order from the base (old) to the top (young). Radiocarbon dates are calibrated to calendar years before AD 2000 using IntCal20 (Reimer et al., Reference Reimer, Austin, Bard, Bayliss, Blackwell, Bronk Ramsey, Butzin, Edwards, Friedrich, Grootes, Guilderson, Hajdas, Heaton, Hogg, Kromer, Manning, Muscheler, Palmer, Pearson, Van der Plicht, Reim Richards, Scott, Southon, Turney, Wacker, Adolphi, Büntgen, Fahrni, Fogtmann-Schulz, Friedrich, Köhler, Kudsk, Miyake, Olsen, Sakamoto, Sookdeo and Talamo2020). The most likely ages are in bold
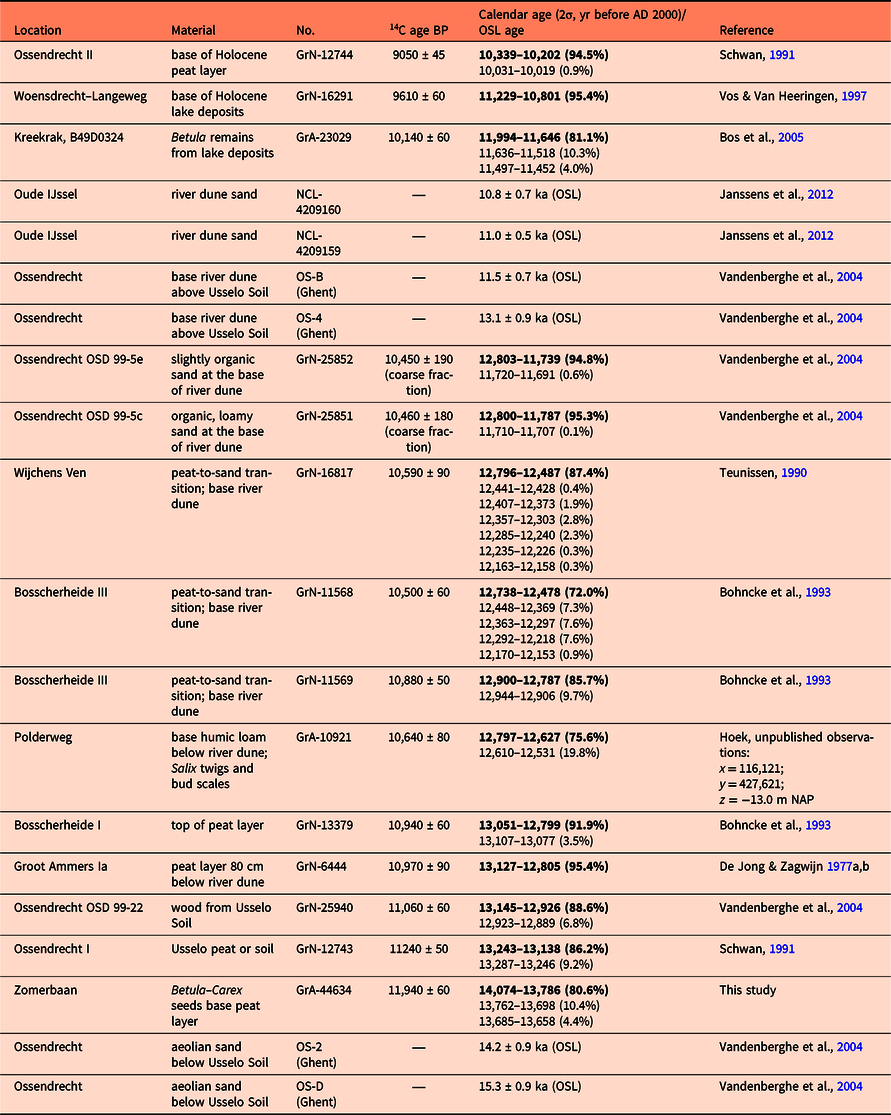

Fig. 3. Digital elevation model of the study area. (A) Large-scale Younger Dryas parabolic dune morphology and small-scale drift-sand relief on the Brabantse Wal, the Scheldt escarpment and the polder area in the west with tidal channel and marsh morphology. Site O = Ossendrecht (De Jong & Zagwijn, Reference De Jong and Zagwijn1966); W = Woensdrecht (Vos & Van Heeringen, Reference Vos and Van Heeringen1997); K = Kreekrak (Bos et al., Reference Bos, Huisman, Kiden, Hoek and Van Geel2005); (B) Polder area west of the Scheldt escarpment with higher areas that reflect subsurface dunes (dotted lines), visible due to embankment, drainage and differential subsidence. Cross sections Woensdrecht, Zomerbaan and Braakseweg and pollen site Zomerbaan are indicated. For location see (A).
Schwan (Reference Schwan1991), in his sedimentological study of the Weichselian Late Glacial to Holocene aeolian succession in sandpit Boudewijn (Ossendrecht), presented an uncalibrated radiocarbon date for the Late Glacial Usselo Soil of Allerød age (11,240 ± 50 BP; c.13.2–13.1 ka cal BP) (GrN-12743) underlying the Younger Dryas dunes, and a date for the base of the overlying Holocene peat layer (9050 ± 45 BP; c.10.3–10.2 ka cal BP) (GrN-12744). Vandenberghe et al. (Reference Vandenberghe2004) and Fink (Reference Fink2000) dated the aeolian sediments at Ossendrecht below and above the Usselo Soil by optical stimulated luminescence (OSL) dating. They obtained OSL ages between 14.2 ± 0.9 ka (OS-2) and 15.3 ± 0.9 ka (OS-D) (Vandenberghe et al., Reference Vandenberghe2004) and 18.1 ± 2.9 ka (Fink, Reference Fink2000) for the Late Weichselian aeolian sediments underlying the Usselo Soil. Younger Dryas river dune sediments overlying the Usselo Soil were OSL-dated between 11.5 ± 0.7 (OS-B) and 13.1 ± 0.9 ka (OS-4) (Vandenberghe et al., Reference Vandenberghe2004) and 10.0 ± 1.5 and 15.1 ± 3.9 ka (Fink, Reference Fink2000). Kasse & Aalbersberg (Reference Kasse and Aalbersberg2019) presented radiocarbon dates of 11,060 ± 60 (c.13.1–12.9 ka cal BP) (GrN-25940) for the Allerød soil and 10,460 ± 180 (c. 12.8–11.8 ka cal BP) (GrN-25851) and 10,450 ± 190 BP (c. 12.8–11.7 ka cal BP) (GrN-25852) for the Younger Dryas peat underlying the river dune sand at Ossendrecht.
In the polder area west of the Brabantse Wal, the Late Glacial Scheldt valley is covered with Holocene deposits due to sea-level rise. Two kilometres west of Ossendrecht, the Geological Survey investigated the 15 m thick Holocene infill of the Scheldt palaeovalley (borehole number B49D0772; x = 79,680; y = 379,005; z = +0.8 m NAP (normal Amsterdam water level), indicated with O in Fig. 3A). Pollen diagram Ossendrecht III comprises (sandy) gyttja deposits from c.15–13.5 m below surface, overlain by 1 m of peat (Basisveen) (De Jong & Zagwijn, Reference De Jong and Zagwijn1966; diagram #41 in Hoek, Reference Hoek1997b), showing that the organic deposition started at the Younger Dryas to Preboreal transition with relatively high values of juniper (Juniperus) followed by birch (Betula) and pine (Pinus) (Hoek, Reference Hoek1997a).
In the northwestern part of the research area near Kreekrak (borehole number B49D0324; x = 75,110; y = 383,240; z = −1.34 m NAP, indicated with K in Fig. 3A), Bos et al. (Reference Bos, Huisman, Kiden, Hoek and Van Geel2005) investigated the Early Holocene lake deposits on top of the coversands at c.14 m below surface. The lake sediments are overlain by c.0.35 m of amorphous peat (Basisveen). The pollen diagram shows that the infill of the lake started at the transition to the Holocene, which is supported by an accelerator mass spectrometry (AMS) radiocarbon date of 10,140 ± 60 BP (c.12.0–11.6 ka cal BP) (GrA-23029) on birch (Betula) macro remains from the base of the organic sequence.
Vos & Van Heeringen (Reference Vos and Van Heeringen1997) dated the base of an organic lake deposit in a depression of the Pleistocene surface in the polder area west of Woensdrecht (x = 79,065; y = 383,410; z = +0.8 m NAP, location W in Fig. 3A). The date of 9610 ± 60 BP (c.11.2–10.8 ka cal BP) (GrN-16291) indicates an early Holocene age for the start of organic deposition following the formation of the late Weichselian river dunes.
Methods
The study area has been investigated since the 1990s by Earth Sciences and Earth and Economics first-year students from the Vrije Universiteit (VU) Amsterdam.
Hand drillings were performed on the Brabantse Wal and in the polder area west of the Wal (Fig. 3B). The Edelman core was used for sediments above the groundwater table. A suction tube was used for water-saturated sands and a gauge was used for water-saturated clay and peat in the polder area. Student core descriptions are in accordance with NEN 5104. Based on coring descriptions from the Earth Sciences first-year students between 1994 and 2000, the top of the Pleistocene surface has been mapped using a total of >1500 corings, of which some 700 reached the Pleistocene surface (Fig. 8B further below). Based on these corings, overview cross-sections DD′ (Fig. 4) at y = 382,000 and AA′ (Fig. 5) at y = 383,750 have been constructed using projected core descriptions indicated with a vertical depth bar for boreholes within 100 m of the cross section, and a horizontal baseline for boreholes within 250 m. Detailed cross sections in the polder area, Zomerbaan BB′ at y = 382,250 (Fig. 6) and Braakseweg CC′ at y = 382,000 (Fig. 7), are based on Earth and Economics student reports 2016–2017–2018.

Fig. 4. General west–east cross section DD′ showing the geology of the study area and the relation of the Late Pleistocene and Younger Dryas dune deposits (unit 2) in the polder area and on the Brabantse Wal (for location see Figs 3B and 8B). Projected core descriptions within 100 m and 250 m of the cross section are indicated with a vertical depth bar and a horizontal baseline respectively.

Fig. 5. West–east cross section Woensdrecht AA′ showing the strongly undulating Late Glacial dune morphology (unit 2). The base of the lake deposits (unit 5) was dated at 9610 ± 60 BP (c.11.2–10.8 ka cal BP) (GrN-16291; projected to AA′) by Vos & Van Heeringen (Reference Vos and Van Heeringen1997) (Fig. 3A, site W) and presents the minimum age of the Pleistocene surface. For location see Figure 3B; for legend see Figure 4.

Fig. 6. Detailed west–east cross section Zomerbaan BB′ showing the subsurface Younger Dryas dune morphology (unit 2) buried by the Holocene deposits (units 3–7). Location of investigated core Zomerbaan is indicated by the star. For location see Figure 3B; for legend see Figure 4.

Fig. 7. Detailed west–east cross section Braakseweg CC′ showing the subsurface Younger Dryas dune morphology (unit 2) buried by the Holocene deposits (units 3–7). The deeply incised Late Glacial valley is demonstrated by the steep drop of the Pleistocene surface from −1 to −12 m NAP west of Zomerbaan and the rise of unit 2 to −8 m NAP in the western part of the cross section. For location see Figure 3B; for legend see Figure 4.

Fig. 8. (A) General elevation map and topographic profiles of the Pleistocene surface west of the Brabantse Wal, based on the ‘top Pleistoceen’ layer from GeoTOP (GeoTOP_v01r3_lagenmodel) of TNO Geological Survey of the Netherlands. Terrace levels T1–T2–T3 and topographic scarps are indicated by dotted lines. For location see Figure 3A. (B) Detailed elevation map of the top Pleistocene surface of the study area based on student corings 1994–2000 showing the deeply incised south–north Younger Dryas – Preboreal Scheldt valley (−15 m NAP) west of the escarpment and isolated high occurrences of the Pleistocene surface and Younger Dryas dunes east of the valley near Woensdrecht (W) and Zomerbaan (Z). Cross sections Woensdrecht AA′, Zomerbaan BB′, Braakseweg CC′ and DD′ are indicated. W = Woensdrecht (Vos & Van Heeringen, Reference Vos and Van Heeringen1997); K = Kreekrak (Bos et al., Reference Bos, Huisman, Kiden, Hoek and Van Geel2005); O = Ossendrecht (De Jong & Zagwijn, Reference De Jong and Zagwijn1966); Z = Zomerbaan (this study).
The reconstruction of the top Pleistocene surface (Fig. 8A) is based on the existing ‘top pleistoceen’ layer from GeoTOP (GeoTOP_v01r3_lagenmodel) of TNO Geological Survey of the Netherlands. Contour lines with an interval of 1 m were added to improve visualisation of the palaeotopography.
In cross section Zomerbaan in the polder area. a peat layer was found c.5.5 m below the surface and 4 m below the top of the Pleistocene river dune (Fig. 6). Core Zomerbaan (x = 79,175; y = 382,400; z = +1.1 m NAP) was retrieved at 540–588 cm depth using a hand-operated bailer to remove the water-saturated sand within a 10 cm PVC casing, until humic sand was reached at 540 cm. The humic sand and peat were retrieved with a 6 cm gauge and analysed for loss on ignition (LOI) and pollen. Macrofossils were selected for radiocarbon dating.
Pollen analysis enables reconstruction of temporal vegetation changes. Pollen Assemblage Zones (PAZ) are defined based on changes in species composition. They are correlated with the biochronostratigraphic zonation for the Late Glacial in the Netherlands (Van Geel et al., Reference Van Geel, Coope and Van der Hammen1989; Hoek, Reference Hoek1997a; Bos et al., Reference Bos, Bohncke and Janssen2006) which provides indirect dating for the deposition of the overlying aeolian river dune. The core was described in the laboratory, and pollen sample location was based on lithology and lithological transitions. The pollen samples were prepared following standard procedures (Faegri & Iversen, Reference Faegri and Iversen1989). The pollen slides were examined using a Zeiss Axioskop 50 light microscope with a magnification of 630× with and without phase contrast. Pollen was determined using the pollen key of Moore et al. (Reference Moore, Webb and Collinson1991). The pollen sum includes tree taxa, shrubs and upland herbs. Wetland taxa, aquatics and Cyperaceae are excluded from the pollen sum. Pollen diagrams were constructed using TILIA software in which taxa are arranged in ecological groups (Grimm, Reference Grimm1992).
An AMS radiocarbon date on selected terrestrial macrofossils was taken from the base of the peat in core Zomerbaan. For the top of the peat, which shows the onset of aeolian sand deposition, no terrestrial macrofossils for AMS dating could be retrieved. The aim of radiocarbon dating is to support the biostratigraphic interpretation that can subsequently be used to establish the start and end of peat formation and indirectly the onset of aeolian river dune deposition. Radiocarbon dates in the text are referred to in years BP. Calibrated radiocarbon dates mentioned in the text are calibrated using IntCal 20 (Reimer et al., Reference Reimer, Austin, Bard, Bayliss, Blackwell, Bronk Ramsey, Butzin, Edwards, Friedrich, Grootes, Guilderson, Hajdas, Heaton, Hogg, Kromer, Manning, Muscheler, Palmer, Pearson, Van der Plicht, Reim Richards, Scott, Southon, Turney, Wacker, Adolphi, Büntgen, Fahrni, Fogtmann-Schulz, Friedrich, Köhler, Kudsk, Miyake, Olsen, Sakamoto, Sookdeo and Talamo2020) and referred to in years cal BP.
Sandpit Boudewijn at Ossendrecht, previously exploited for brick production, was visited yearly (Fig. 3A). In the last stage of exploitation, five lacquer peels were taken from the Younger Coversand II unit, overlying the Usselo Soil, for sedimentological analysis and the reconstruction of wind direction. The pit face was cleaned with an angle of c.60°. Lacquer peels were made following standard procedures (Van Baren & Boomer, Reference Van Baren and Boomer1979). The orientation of the peels was chosen in different directions in order to enable a 3D reconstruction of wind directions during deposition.
Results
Geomorphology
The present-day topography and geomorphology reveals two different landscapes separated by an abrupt elevation transition at the Brabantse Wal escarpment (Fig. 3A). The eastern part is c.15–30 m above sea level and characterised by an up to 5 km wide dune field that stretches from Kalmthout in the southeast to Bergen op Zoom in the north. South of Kalmthout the dune field does not seem to be present (Figs 1 and 3A), and north of Bergen op Zoom the dunes are covered and eroded by Holocene deposits. The relief consists of large and high parabolic dune forms, especially at the eastern distal margin of the dune field near Huijbergen and northwest of Kalmthout. The parabolic forms are open to the southwest suggesting a northeasterly transport direction by wind from the southwest. The easternmost slope of the parabolic dunes shows an abrupt lee-side and transition to the gently undulating coversand landscape in the east (Fig. 3A). The relief within the dune field is characterised by smaller-scale parabolic dunes, and irregular dune forms (drift-sand relief). The digital elevation model (DEM) shows a higher elevation of the dune field in the south compared to the north. The dune field is widest (c.8 km) in the south near Kalmthout.
The western part of the study area is c.−1 to 2 m above sea level and characterised by tidal channel and man-made polder embankment morphology (Fig. 3B). The older polders which border the Brabantse Wal and embanked in AD 1685, are lower-lying (at c.0 m NAP) than the younger polders further west (at c.1.5 m NAP), the latter being embanked in the 19th century. The elevation difference is due to the short timespan of marine sedimentation in the low polders, after the 16th-century inundations and the embankment in AD 1685. The younger polders have a higher elevation due to longer-lasting sedimentation and increasing tidal range.
In addition, small-scale morphology is present in the older polders of AD 1685 indicated with dotted lines in Figure 3B. They represent circular to oval, c.500 m long and 250 m wide, up to 1 m high areas. Coring indicated that the top of the Pleistocene sand surface is close (0–2 m) to the present-day surface (Figs 4–7). Core Zomerbaan was taken at such a high location. The present-day highs correspond to subsurface Pleistocene highs that are visible due to embankment, drainage and differential subsidence. Compaction and subsidence is limited in the sandy sediments, while clay, peat and gyttja are more susceptible to compaction.
Lithostratigraphy and environmental interpretations
The chrono- and lithostratigraphy of the study area is summarised in Figure 2. General cross section DD′ (Fig. 4) shows the geological setting of the area and the relation between the Late Pleistocene and Holocene units on top of the Brabantse Wal and in the polder area. The Early Pleistocene deposits (unit 1; Waalre Formation) show a fining-upward trend with well-sorted, fine-grained silty sands rich in micas overlain by silty clay. On top of the Early Pleistocene massive clay, a thin coarse-grained sand or gravel layer was frequently recognised on the Brabantse Wal, which is interpreted as the Beuningen Gravel Bed of Weichselian Late Pleniglacial age. Late Pleniglacial and Late Glacial aeolian coversand (lower part unit 2; Boxtel Formation, Wierden Member) and Younger Dryas aeolian river dunes (upper part unit 2; Boxtel Formation, Delwijnen Member; Fig. 2) are present on the Brabantse Wal at +15–20 m NAP and east of the Scheldt palaeovalley in the polder area at 0 to −5 m NAP. At the base of the aeolian river dune deposits, a compact peat layer is found occasionally. On the Brabantse Wal, this peat layer is correlated with the Usselo Soil (Vandenberghe et al., Reference Vandenberghe2004). The Holocene drift sands (unit 8; Boxtel Formation) are separated from the Late Glacial river dune deposits by the Holocene podzol soil (Kasse & Aalbersberg, Reference Kasse and Aalbersberg2019). Calcareous and organic Holocene lake deposits (unit 5) are highlighted in the depression between the Late Glacial dunes in the polder area and the Brabantse Wal escarpment.
Cross section AA′ (Fig. 5) shows the general sedimentary sequence in front of the Brabantse Wal. The Late Glacial sand deposits (unit 2; Boxtel Formation, Delwijnen Member) are generally moderately sorted fine to medium coarse sands that show a subsurface dune relief. The lake deposits (unit 5; Nieuwkoop Formation, Hollandveen Member) in the polder area are covered with 2 m of clay deposits. The presence of the river dune probably caused isolation of the basin and a high water table related to groundwater seepage from the Brabantse Wal and the dune, as the basal fill of the basin was dated in the Early Holocene (9610 ± 60 BP; c.11.2–10.8 ka cal BP) (GrN-16291); Vos & Van Heeringen, Reference Vos and Van Heeringen1997).
The cross sections BB′ (Fig. 6) and CC′ (Fig. 7) illustrate the lithostratigraphy of the polder area in more detail and the stratigraphic position of the investigated core and pollen diagram Zomerbaan. Seven units are distinguished in the cross sections (Figs 2, 6 and 7). More detailed information related to the Holocene sequence and palaeogeographical development can be found in Vos & Van Heeringen (Reference Vos and Van Heeringen1997).
Unit 1 (Waalre Formation) is exposed in the Brabantse Wal escarpment and is characterised by well-sorted, fine to medium sands with clay pebbles or laminae and muscovite minerals. Unit 1 was deposited in the tidal and estuarine Rhine–Scheldt system of Early Pleistocene age (Kasse, Reference Kasse1988, Reference Kasse1990).
Unit 2 (Boxtel Formation, Delwijnen Member) is found both in the polder area at 0 to −10 m NAP and on the Brabantse Wal at 15 to 20 m NAP (Fig. 4). It consists of clean, moderately sorted medium sands which are interpreted as Late Glacial aeolian dune sands. Units 1 and 2 occur at the base of the cross sections and represent the top of the Pleistocene surface (Figs 6 and 7). Unit 1 is easily recognised in the Brabantse Wal escarpment; however, in the polder area the distinction between units 1 and 2 is less clear because of limited hand-core penetration depth. The top of units 1 and 2 shows a strongly undulating morphology (Figs 6 and 7). From east to west the elevation of units 1 and 2 drops from +13 m NAP at De Koepel to −2 m NAP and then rises slightly above 0 m NAP at Schenkeldijk/’t Marktje, followed by a depression at −3 to −6 m NAP and a next high at Zomerbaan around 0 m NAP. West of Zomerbaan, the top of unit 2 descends steeply to −12 m and deeper near the Agger. In the west of the profile the top of unit 2 rises again to −8 m NAP (Fig. 7). The morphology of the top of unit 2 indicates aeolian morphology in the central part of the cross sections and the presence of a deep Scheldt palaeovalley approximately at the location of the subrecent Agger (Vos & Van Heeringen, Reference Vos and Van Heeringen1997, map 1). In core 2018.9.8 (Fig. 7) unit 2 is covered by a non-calcareous stiff clay that can be interpreted as a floodplain deposit overlying fluvial sediments (equivalent of the Wijchen Layer). Therefore, the deeper parts of unit 2 can be fluvial in origin (Koewacht Formation). Locally, a peat layer was found in unit 2 at c.5.5 m below the surface (c.−4 m NAP (Fig. 6: core 2017.18.2), at similar depth to that in core Zomerbaan, which was sampled for pollen analysis and radiocarbon dating.
Unit 3 (Nieuwkoop Formation, Basisveen Bed) was found only occasionally in the west (Fig. 7). It consists of organic-rich sediments and peat. In core 2018.9.8 it was developed as a calcareous gyttja. Unit 3 is ascribed as formed by increasing surface wetness related to Holocene sea-level rise (Vos & Van Heeringen, Reference Vos and Van Heeringen1997).
Unit 4 (Naaldwijk Formation, Wormer Member) is found with great thickness in the Late Glacial Scheldt palaeovalley. Rapid lateral facies changes are observed from clean grey sandy clay in the axis of the palaeovalley to reed-grown clays at the valley margins (Fig. 7: core 2018.9.5). The absence of marine shells and presence of well-developed mm-scale, sand–clay laminations indicate tidal deposition in a fresh- to brackish-water environment in the upstream part of the Scheldt system (Vos & De Wolf, Reference Vos and De Wolf1997; Vos & Van Heeringen, Reference Vos and Van Heeringen1997: 52). The large thickness and upward-decreasing grain size in unit 4 reflect rapid sea-level rise and drowning of the Late Pleistocene palaeovalley in the early and middle Holocene (Vos & Van Heeringen, Reference Vos and Van Heeringen1997). In the investigated profiles, a humic clay/gyttja (Kreekrak Formation) overlying unit 3 and gradually grading into unit 4 has not been observed.
Unit 5 (Nieuwkoop Formation, Hollandveen Member) is a peat layer and is found over most of the cross sections, except where the dunes of unit 2 occur at a high elevation of c.0 m NAP (Figs 6 and 7: Zomerbaan, ’t Marktje, Schenkeldijk) and where the tidal channel of unit 6 has eroded the peat layer west of the Agger. The elevation of the top of the peat varies from +1 m to −4 m NAP, which is related to later compaction during and after sedimentation of units 6 and 7. Strongest compaction has occurred in the westernmost part and in the depression east of the Zomerbaan (Fig. 7: core 2018.2.5). Mesotrophic to oligotrophic sedge and moss peat occur in the western part of the transects. Eutrophic wood and reed peats are dominant in the central part and are related to the position of the Scheldt at that time. Mesotrophic sedge peat and gyttja are found in the eastern part east of Zomerbaan, related to groundwater seepage at the foot of the Brabantse Wal escarpment.
Unit 6 (Naaldwijk Formation, Walcheren Member) is found in the western part of the cross sections, west of the Agger, where it reaches a depth up to −11 m NAP. Peat unit 5 is absent there, which indicates deep channel erosion prior to deposition of units 6 and 7. Unit 6 consists of fine to medium, carbonate-rich sands and sandy clay. The presence of marine shell fragments and tidal laminations point to a tidal channel environment. In the Middle Ages the Scheldt channel at this location formed the western boundary of the Duchy of Brabant. During catastrophic floods in the 16th century the area was flooded by the sea and transformed in a tidal area with channels (unit 6) and tidal flats and marshes (unit 7). The tidal channel probably migrated to the east, and the Agger or Kapitale Uitwatering is the final remnant of this tidal channel that functioned till polder embankment in AD 1685 (Fig. 3B). The sand-filled tidal channel (unit 6) has a high surface elevation (Figs 3B and 7: core 2018.2.2) in comparison to neighbouring areas. In the tidal channel, peat was eroded and replaced by non-compactable fine-grained sands. East and west of the channel, compaction of peat and simultaneous subsidence led to inversion of the relief.
Unit 7 (Naaldwijk Formation, Walcheren Member) is thicker in the west and consists of carbonate-rich sandy clay. Unit 7 thins towards the east and consists of non-calcareous clay indicating lower-energy conditions at the foot of the Brabantse Wal. The presence of marine shells (Scrobicularia, Mytilus) and Hydrobia snails points to tidal-flat and salt marsh depositional environments. East of Zomerbaan, medium-grained, non-calcareous sand beds are present in unit 7 (e.g. Fig. 7: core 2018.1.12). These sands resemble unit 2, but because of their stratigraphic position, above peat unit 5, they probably represent reworked unit 2 sands. Abrasion by waves during formation of unit 7 eroded the former dune tops (Fig. 6: 2017.18.2; 2018.1.2) and the sand was deposited as splays in lee-side depressions east of the dunes.
Reconstruction of the top of the Pleistocene surface in the polder area
The top Pleistocene surface west of the Brabantse Wal was retrieved from the GeoTOP model from the Dutch Geological Survey, which is based on digital borehole logs and cone-penetration tests (Fig. 8A). The map shows the high area of the Brabantse Wal in the east and the up to 20 m deep elongated depression west of the Brabantse Wal escarpment. The depression is the combined effect of the Younger Dryas to Preboreal incision of the Scheldt (top of units 1 and 2) and late Holocene erosion of the medieval course of the Scheldt (Agger) (units 6 and 7 Walcheren Member) (see cross sections Figs 6 and 7). West and east of the valley, northward-dipping areas occur, parallel to the palaeovalley of the Scheldt. Three topographic levels are distinguished based on abrupt changes in elevation (topographic scarps). The highest level, T1, is present at −3 to −4 m NAP (Rug van Rilland; Kiden, Reference Kiden, Starkel, Gregory and Thornes2006: fig. 3). Level T2 occurs between −5 and −9 m NAP, and the lowest level T3 at −7 to −11 m below NAP. The deep valley at more than −15 m NAP has not been labelled as a fourth level (see Discussion). The spatial relation to the Scheldt palaeovalley suggests a fluvial origin and we tentatively interpret these surfaces as Late Pleistocene (partly Late Pleniglacial and Late Glacial) river terraces of the Scheldt, locally covered by aeolian deposits.
The subsurface relief of the Pleistocene sandy surface and dunes in the study area west of the Brabantse Wal was reconstructed in more detail by interpolation of VU student corings 1994–2000 that reached the top of the Pleistocene surface (Fig. 8B). The top of the Pleistocene surface declines rapidly to the west, where the up to 15 m deep palaeovalley is present west of the Brabantse Wal. West of the palaeovalley the top Pleistocene surface increases again up to −7 to −9 m NAP (level T3 in Fig. 8A) with local highs (possibly dunes) up to −4 m NAP. In addition, local high areas up to 0 m NAP are visible between the deep palaeochannel and the Brabantse Wal escarpment (Fig. 8B, indicated with Z and W). These higher areas also show up on the DEM of the present-day surface (Fig. 3B), especially where the Pleistocene dune sand is covered by thin Holocene deposits (see cross sections Figs 6 and 7). The pollen-investigated peat layer at Zomerbaan (Z in Figs 3B and 8B) is situated below such a local high of the Pleistocene surface where the sand is found at c.1 m below the surface.
LOI and pollen analysis of core Zomerbaan
Cross section Zomerbaan reveals the presence of a peat layer at the base of unit 2 at 5.4 to 5.9 m below the surface (Fig. 6). This layer was sampled at a nearby location for pollen analysis (Fig. 3B). Pollen Assemblage Zones (PAZ) are distinguished in pollen diagram Zomerbaan (Fig. 9) and are correlated with the biochronostratigraphic zonation for the Late Glacial in the Netherlands (Van Geel et al., Reference Van Geel, Coope and Van der Hammen1989; Hoek, Reference Hoek1997a; Bos et al., Reference Bos, Bohncke and Janssen2006).

Fig. 9. Pollen diagram Zomerbaan showing the birch (Betula) and pine (Pinus) phases of the Allerød (zones 2a, 2b) and the transition to the Younger Dryas (zone 3) in the top of the peat layer, covered by the Younger Dryas aeolian river dune sand. For location see Figures 3B and 6 (x = 79,175; y = 382,400; z = +1.1 m NAP).
PAZ 1c (590–588 cm, humic sand): In this interval, LOI values are still low (10%). This zone is characterised by low but gradually increasing arboreal pollen values. Pine (Pinus) values are low, juniper (Juniperus) and willow (Salix) are present and birch (Betula) values are increasing in this zone. Upland herbs are an important element of the vegetation. Relatively high values of Cyperaceae indicate the presence of sedges. The low arboreal pollen values and dominance of herbs indicate an open landscape, which is characteristic for the Older Dryas period (Hoek, Reference Hoek1997a; zone 1c).
PAZ 2a1 (588–572 cm, detrital gyttja, with moss layer near the top): LOI values increase abruptly at the base of this zone to 40%, and to a maximum of 70% in the upper part. Zone 2a1 shows lower non-arboreal pollen values than PAZ 1c. High arboreal pollen values are characterised mainly by the presence of birch (Betula) and juniper (Juniperus). The dominance of Betula in combination with Artemisia indicates an open birch forest. The high birch values are indicative for the early Allerød period (Betula phase; Hoek, Reference Hoek1997a; zone 2a1). The presence of twigs indicates local presence of birch. An AMS radiocarbon date on Betula and Carex seeds from the base of PAZ 2 yielded an age of 11,940 ± 60 BP (c.14.1–13.8 ka cal BP) (GrA-44634). The date indicates the start of the Allerød period and supports the biochronostratigraphic interpretation.
PAZ 2a2 (572–555 cm, moss peat): LOI values are between 50 and 70%, comparable to PAZ 2a1. Birch (Betula) is the dominant tree species and juniper has declined. Empetrum (crowberry) is present in low values and the presence of trilete moss and fern spores indicates more acid environmental conditions. Pine (Pinus) shows a gentle increase to 15% in the upper part of this zone. The dominance of birch is characteristic for the Betula phase of the Allerød period and this zone is correlated to zone 2a2 sensu Hoek (Reference Hoek1997a). The presence of Empetrum has been reported in some other diagrams as well during this phase, such as Usselo I (Van Geel et al., Reference Van Geel, Coope and Van der Hammen1989), although this species is more common during the Younger Dryas (Hoek, Reference Hoek1997a).
PAZ 2b (555–552 cm, moss peat): LOI values are between 60 and 70%. Pine (Pinus) shows a large increase up to 80%, and birch (Betula) values strongly decrease to 20%. Upland herb values are low, pointing to a closed pine-dominated forest. The decrease of moist taxa such as sedges and disappearance of horsetail (Equisetum) indicate drier local conditions. The high pine values are indicative for the Pinus phase of the Allerød (Hoek, Reference Hoek1997a; zone 2b).
PAZ 3 (552–540 cm, humic sand): The LOI values show a sharp drop to 5%, and the moss peat changes into a humic sand. Pine (Pinus) decreases and willow (Salix) shows an increase. Gramineae and Cyperaceae increase, indicating a more open landscape (Hoek & Bohncke, Reference Bohncke2002). The distinct drop of pine in favour of herbaceous taxa is commonly associated with the end of the Allerød or start of the Younger Dryas (Hoek, Reference Hoek1997a; zone 3). The biostratigraphical change coincides with the lithological transition from peat to sand, indicating the onset of aeolian deposition at or shortly after the Allerød – Younger Dryas transition.
Description and interpretation of lacquer peels
Five lacquer peels were made in sandpit Boudewijn (Ossendrecht; Fig. 3A) from the Younger Dryas sand unit overlying the Usselo Soil for the reconstruction of sand-transporting (storm) wind direction. The orientation of the peels was chosen in different directions in order to enable a 3D reconstruction of wind directions during deposition (Fig. 10A and B). The sedimentary structures are dominated by: (a). horizontal lamination and low-angle cross bedding formed by dry-aeolian deposition on a flat surface or in low dunes (Hunter, Reference Hunter1977); (b). crinkly lamination and massive bedding; and (c) adhesion ripple cross lamination formed by wet-aeolian deposition on a water-saturated flat surface (Hunter, Reference Hunter1980). As adhesion ripple cross lamination enables reconstruction of wind direction, the number of adhesion ripple cross-laminated sets was counted in the five peels. Peel OSD04-2 contains seven sets indicating a WSW wind direction (N239) (Fig. 10A); peel OSD04-1 contains six sets indicating a S wind direction (N175) (Fig. 10B); OSD04-3 contains five sets indicating a SW wind direction (N233), and OSD04-5 contains five sets indicating a WSW-wind direction (N250); OSD04-4 contains no adhesion ripple sets (orientation of the peel N113–N293). The number of sets and their orientation are plotted in a rose diagram (Fig. 11). The figure shows a strong dominance of adhesion ripple sets indicating southerly to westerly winds. No adhesion ripple sets were found indicating northerly and easterly winds.

Fig. 10. Lacquer peels OSD04-1 and OSD04-2 of the base of the Younger Dryas river dune deposits in sandpit Boudewijn at Ossendrecht (for location see Fig. 3A). Note the presence of multiple sets of adhesion ripple cross lamination (c) indicating southerly and southwesterly wind directions. Bedding types are: (a) horizontal lamination and low-angle cross bedding formed by dry-aeolian deposition on a flat surface or in low dunes; (b) crinkly lamination and massive bedding; and (c) adhesion ripple cross lamination both formed by wet-aeolian deposition on a water-saturated surface.

Fig. 11. Rose diagram of the Younger Dryas wind direction based on the number of adhesion ripple sets in five lacquer peels. Southwesterly and southerly (summer) wind directions are dominant.
Discussion
Spatial distribution and genesis of source-bordering dunes
The spatial distribution of the Younger Dryas dune field (for discussion on age see below) on the Brabantse Wal has been derived from the DEM analysis (Figs 1 and 3A). The eastern boundary is based on the steep eastern slope of large parabolic dune ridges east of Huijbergen, that are interpreted as the lee-side or slipface of the dune complex. The orientation of the parabolic dunes and the north–south orientation of the dune field indicate an important sand supply from the southwest.
The higher elevation of the dune field in the south (c.20–25 m) compared to the north (c.10–15 m east of Bergen op Zoom) (Figs 1 and 3A) is due to the northern tectonic-induced dip (c.0.8 m/km between Ossendrecht and Halsteren) of the Early Pleistocene Waalre Formation (Kasse, Reference Kasse1988). The aeolian sand was blanketed over the Waalre Formation, and therefore does not reflect more accumulation of dune sand in the south. The large width of the dune field (c.8 km) northwest of Kalmthout may be related to the absence of continuous clay beds in the Waalre Formation southwest of the line Ossendrecht–Putte–Kalmthout. At Ossendrecht the Brabantse Wal escarpment bends eastwards and continues as the Campine clay microcuesta in the direction of Turnhout (De Ploey, Reference De Ploey1961; Kasse, Reference Kasse1988) (Fig. 1). Southwest of the microcuesta (Ossendrecht–Putte–Kalmthout) the clay at the top of the Waalre Formation has been eroded and Early Pleistocene sands are exposed. Because of the deep groundwater level in the escarpment, drier conditions occur, more sand was available for deflation and the dunes migrated further to the east. However, northeastward dune migration retarded as soon as the sand was blown onto the escarpment or microcuesta, because of vegetation and wet surface conditions related to the perched water table above the impermeable clay beds of the Waalre Formation. The perched water table is presently still reflected by the presence of small lakes in that area (e.g. Groote Meer, Kleine Meer, Stappersven, Putse Moer).
West of the Brabantse Wal the Pleistocene surface (and Younger Dryas dunes) was mapped by corings (Figs 4–8). The Pleistocene surface shows an up to 15 m deep, elongated depression that is interpreted as the final Late Glacial Scheldt valley (Westerhoff & Dobma, Reference Westerhoff and Dobma1995; Vos & Van Heeringen, Reference Vos and Van Heeringen1997; Kiden, Reference Kiden, Starkel, Gregory and Thornes2006: fig. 3). The oldest organic fill in the palaeovalley (De Jong & Zagwijn, Reference De Jong and Zagwijn1966: pollen diagram Ossendrecht III; diagram #41 in Hoek, Reference Hoek1997b) shows that organic deposition started at the Younger Dryas to Holocene transition with relatively high values of juniper (Juniperus) followed by birch (Betula), characteristic for the start of the Preboreal (Hoek, Reference Hoek1997a). This indicates that the valley was formed by incision before the Preboreal, possibly at the Younger Dryas to Holocene transition. The deep valley can be interpreted as the fourth terrace (T4); however, it has not been labelled as such, because of the channel morphology instead of terrace morphology (Fig. 8A). In addition, the valley has a multi-genetic origin partly formed by fluvial incision at the Younger Dryas to Holocene transition, partly by late Holocene tidal channel erosion. Similar fluvial incisions at the Younger Dryas to Holocene transition are reported frequently for northwestern and central European river systems (Kozarski, Reference Kozarski1983; Berendsen et al., Reference Berendsen, Hoek and Schorn1995; Bohncke et al., Reference Bohncke, Kasse and Vandenberghe1995; Kasse et al., Reference Kasse1995, Reference Kasse, Bohncke, Vandenberghe and Gábris2010; Huisink, Reference Huisink1997; Starkel, Reference Starkel2002; Janssens et al., Reference Janssens, Kasse, Bohncke, Greaves, Cohen, Wallinga and Hoek2012; Woolderink et al., Reference Woolderink, Kasse, Cohen, Hoek and Van Balen2018).
West of the valley, the Pleistocene surface shows several plateau-like levels (T1–T2–T3) at c.−3 to −11 m NAP, that are interpreted as former terraces (Fig. 8A). The Preboreal age of the basal infill at c.−15 m NAP of the incised Scheldt palaeovalley indicates that the proposed terrace levels at the top of the Pleistocene surface west and east of the valley are older than the Holocene. The elevation of the Allerød peat layer at Zomerbaan (−5 m NAP) indicates that the floodplain surface and water level during and shortly before the Allerød were at c.−5 m NAP. Assuming a fairly flat river plain, this elevation corresponds with level T2 at this location (Fig. 8A: transect II–II′). In line with upstream Late Glacial channel patterns of the Scheldt (Kiden, Reference Kiden, Starkel, Gregory and Thornes1991, Reference Kiden2006) and other river systems (Rhine, Meuse, Warta) we propose a similar Pleniglacial and Late Glacial development of the Scheldt and suggest that the lowest level, T3, might represent the Younger Dryas terrace and the next higher levels the Bølling–Allerød (T2) and Pleniglacial (T1) terraces (Kozarski, Reference Kozarski1983; Kasse et al., Reference Kasse1995, Reference Kasse, Hoek, Bohncke, Konert, Weijers, Cassee and Van der Zee2005; Berendsen et al., Reference Berendsen, Hoek and Schorn1995; Bohncke et al., Reference Bohncke, Kasse and Vandenberghe1995; Erkens et al., Reference Erkens, Hoffmann, Gerlach and Klostermann2011; Woolderink et al., Reference Woolderink, Kasse, Cohen, Hoek and Van Balen2018).
Local highs of the Pleistocene surface (top of units 1 and 2; Fig. 8B) in between the incised palaeovalley and the Brabantse Wal escarpment are interpreted as dunes because of the morphology, homogeneous character of the sand and absence of grain-size trends (Figs 4–7: Langeweg, Zomerbaan, ’t Marktje, Schenkeldijk). During the Holocene, due to sea-level rise, the incised Scheldt valley and adjacent terraces drowned and were covered with clay and peat (Figs 6 and 7). Due to differential compaction of the Holocene peat and clay cover, these Pleistocene highs show up on the DEM (Fig. 3B) where the Holocene cover is thin in comparison to nearby areas with thick Holocene deposits.
The distribution and south–north orientation of the dune field on the Brabantse Wal, the location of the dunes in the polder area west of the Wal and the south–north direction of the Late Glacial incised palaeovalley indicate a genetic relation between the aeolian and fluvial systems. Therefore, the dunes are interpreted as source-bordering dunes (river dunes) that have been formed a short distance downwind of the fluvial source area. The medium to coarse grain size, presence of fine gravel and moderate to poor sorting are connected to the short transport distance of at most 10 km (Kasse & Aalbersberg, Reference Kasse and Aalbersberg2019).
The spatial distribution and genesis of the dune field is determined by: (i) the orientation of the river valley with respect to the prevailing winds from the southwest (see discussion below). The Weichselian Late Glacial Scheldt valley has a south to north orientation (Fig. 8) and sand that was deflated from the valley accumulated on the east bank of the river as source-bordering dunes. These dune fields resemble recent cold-climate analogues along the Kobuk River in Alaska (Koster, Reference Koster1992). Similar dune fields were described on the east bank of the south–north-flowing Meuse River (Teunissen, Reference Teunissen1983; Bohncke et al., Reference Bohncke, Vandenberghe and Huijzer1993; Kasse, Reference Kasse, Troelstra, Van Hinte and Ganssen1995b; Kasse et al., Reference Kasse1995; Hoek et al., Reference Hoek2017; Woolderink et al., Reference Woolderink, Kasse, Cohen, Hoek and Van Balen2018), along the east–west Rhine–Meuse system in the central Netherlands (Pons, Reference Pons1957; Verbraeck, Reference Verbraeck1983; Berendsen et al., Reference Berendsen, Hoek and Schorn1995; Hijma et al., Reference Hijma, Cohen, Hoffmann, Van der Spek and Stouthamer2009) and along the Oude IJssel River (Van de Meene, Reference Van de Meene1977; Janssens et al., Reference Janssens, Kasse, Bohncke, Greaves, Cohen, Wallinga and Hoek2012).
(ii) the channel morphology and floodplain width. The Younger Dryas cooling resulted in lower evapotranspiration, deeper seasonal frost and local permafrost (Isarin, Reference Isarin1997). As a consequence, a more nival discharge regime and higher stream power established, and single-channel meandering rivers changed into multichannel or braided rivers with a wide floodplain e.g. in the Meuse and Rhine valleys (Pons, Reference Pons1957; Berendsen et al., Reference Berendsen, Hoek and Schorn1995; Kasse, Reference Kasse1995a; Kasse et al., Reference Kasse1995; Huisink, Reference Huisink1997; Erkens et al., Reference Erkens, Hoffmann, Gerlach and Klostermann2011; Woolderink et al., Reference Woolderink, Kasse, Cohen, Hoek and Van Balen2018). Such braid plains were wide enough to create sufficient fetch for the wind to take up sand from the temporarily inactive floodplains during low discharge. Because of the extensive dune field east of the Scheldt valley it is postulated that the Scheldt in the study area (west of the Brabantse Wal escarpment) also changed into a braided system. However, this valley is covered by Holocene deposits, and the reconstructed subsurface morphology is not detailed enough to prove the existence of a Younger Dryas braid plain (Fig. 8). Furthermore, direct age control is lacking and therefore no definitive conclusion can be drawn about the Younger Dryas valley width and channel pattern. However, based on the altitudinal correlation of the investigated Allerød peat layer at Zomerbaan with level T2, it is postulated that level T3 likely represents the Younger Dryas floodplain of c.2–3 km width (Fig. 8A). The importance of floodplain width in source-bordering dune formation is further demonstrated in river systems that did not change to a braided style, but persisted in a meandering mode during the Younger Dryas. In the Roer Valley, a Younger Dryas braided system did not establish, nor river-connected dunes, despite the fact that local Younger Dryas deflation of higher sandy Pleniglacial terraces did occur (Kasse et al., Reference Kasse, Van Balen, Bohncke, Wallinga and Vreugdenhil2017). The same holds true for the Niers River that had a meandering planform during the Younger Dryas, and only local aeolian deposition occurred on sandy meander point bars (Kasse et al., Reference Kasse, Hoek, Bohncke, Konert, Weijers, Cassee and Van der Zee2005).
In addition to channel-pattern differences between different river systems, reach-specific channel patterns and related aeolian deposition may also occur (e.g. Houben, Reference Houben2003; Woolderink et al., Reference Woolderink, Kasse, Cohen, Hoek and Van Balen2018). Reach-specific different channel patterns were probably present in the Scheldt valley as well (Fig. 1). The west–east Scheldt valley between Ghent and Dendermonde shows large-scale meander morphology that was assigned to the Late Glacial (Kiden, Reference Kiden, Starkel, Gregory and Thornes1991, Reference Kiden2006; Bogemans & Vandenberghe, Reference Bogemans and Vandenberghe2011; Bogemans et al., Reference Bogemans, Meylemans, Jacobs, Perdaen, Storme, Verdurmen and Deforce2012; Meylemans et al., Reference Meylemans, Bogemans, Storme, Perdaen, Verdurmen and Deforce2013; Crombé, Reference Crombé, Grimm, Weber, Mevel and Sobkowiak-Tabaka2020). Braided systems, associated with the Younger Dryas cooling, are not reported here and probably the Scheldt was a meandering river in that reach during the Younger Dryas, comparable to the Niers and Roer river systems (Kasse et al., Reference Kasse, Hoek, Bohncke, Konert, Weijers, Cassee and Van der Zee2005, Reference Kasse, Van Balen, Bohncke, Wallinga and Vreugdenhil2017; Woolderink et al., Reference Woolderink, Kasse, Grooteman and Van Balen2019). The meandering character of the Scheldt in this part of the valley probably restricted deflation due to few exposed sands and short wind fetch. Limited Younger Dryas dune formation has been reported up to now in comparison to downstream Antwerp and dunes formed especially on higher point bars by deflation of point-bar sediments (Fig. 1 Berlare) (Kiden, Reference Kiden, Starkel, Gregory and Thornes2006: fig. 2; Bogemans & Vandenberghe, Reference Bogemans and Vandenberghe2011; Bogemans et al., Reference Bogemans, Meylemans, Jacobs, Perdaen, Storme, Verdurmen and Deforce2012; Meylemans et al., Reference Meylemans, Bogemans, Storme, Perdaen, Verdurmen and Deforce2013; Crombé, Reference Crombé, Grimm, Weber, Mevel and Sobkowiak-Tabaka2020). In the Hoboken gap the Late Glacial valley morphology is not well known and the south–north-oriented Scheldt valley does not show dune morphology, probably because of limited valley width and later Holocene erosion. The abrupt southern margin of the Younger Dryas dune field north of Antwerp (Figs 1 and 3A: Kalmthout) probably reflects the morphological transition of the Scheldt from an upstream meandering to downstream braided system during the Younger Dryas. According to Kiden (1991: fig 12.7, Reference Kiden2006: fig. 8) the Late Pleistocene Scheldt valley gradient shows a knickpoint (convexity) and gradient increase north of Antwerp, possibly related to the resistant Oligocene clays in the Hoboken gap south of Antwerp. The increase of floodplain gradient downstream of Antwerp caused higher stream power by which the threshold from meandering to braiding could be crossed (Van den Berg, Reference Van den Berg1995; Kleinhans & Van den Berg, Reference Kleinhans and Van den Berg2011). Upstream of Antwerp the Scheldt maintained its meandering character in the course of the Late Glacial.
(iii) Sand availability. Source-bordering dunes consist of aeolian sands that are genetically related to fluvial systems. Hence, the fluvial system must be dominated by sandy bed-load material that was deflated from the braided channel belt. In the Scheldt valley, west of the Brabantse Wal, these conditions are fulfilled as the subsoil consists mostly of Neogene and Early Pleistocene marine and tidal sands (Kasse, Reference Kasse1988, Reference Kasse1990; Westerhoff & Dobma, Reference Westerhoff and Dobma1995: fig. 2). The same holds true for the sand-dominated Meuse valley between Roermond and Nijmegen (Zandmaas) and the Rhine–Meuse valley in the central Netherlands where extensive source-bordering dune complexes have been reported (Pons, Reference Pons1957; Teunissen, Reference Teunissen1983; Verbraeck, Reference Verbraeck1983; Bohncke et al., Reference Bohncke, Vandenberghe and Huijzer1993; Berendsen et al., Reference Berendsen, Hoek and Schorn1995; Kasse, Reference Kasse, Troelstra, Van Hinte and Ganssen1995b; Kasse et al., Reference Kasse1995; Hijma et al., Reference Hijma, Cohen, Hoffmann, Van der Spek and Stouthamer2009; Hoek et al., Reference Hoek2017). South of Roermond the Maas is dominated by gravelly bed-load material and source-bordering sand dunes did not form (Woolderink et al., Reference Woolderink, Kasse, Cohen, Hoek and Van Balen2018).
Age of river dune formation
The age of the river dunes on the Brabantse Wal and in the polder area is mostly based on indirect dating by biochronostratigraphic interpretation of pollen records and radiocarbon dating of organic deposits underlying and overlying the dunes (e.g. Schwan, Reference Schwan1991; Vos & Van Heeringen, Reference Vos and Van Heeringen1997) (Table 1). The start of dune formation was established in core Zomerbaan (Fig. 9). The pollen diagram, retrieved from below the dune, shows a Late Glacial vegetation succession covering the Older Dryas (zone 1c), the birch (Betula) and pine (Pinus) phases of the Allerød (zone 2) and the start of the Younger Dryas period (zone 3). This interpretation is supported by the radiocarbon date of 11,940 ± 60 BP (c.14.1–13.8 ka cal BP) (GrA-44634) (Older Dryas – Allerød transition) from the base of the peat layer. The top of the diagram shows the Allerød to Younger Dryas transition which coincides with a change from peat to aeolian sand. Therefore, the onset of river dune formation at location Zomerbaan occurred during the early Younger Dryas period.
This start of river dune formation is in good agreement with previous palynological research in the area. According to Nelson & Van der Hammen (Reference Nelson and Van der Hammen1950) and Kasse & Aalbersberg (Reference Kasse and Aalbersberg2019: fig. 7A) organic deposits below the river dunes at Hoogerheide and Ossendrecht date from the Allerød period (Betula and Pinus phases) and early Younger Dryas. The Allerød – Younger Dryas transition is characterised by a drop in pine (Pinus), increase in birch (Betula) and upland herbs including crowberry (Empetrum). The lithology changes from detrital gyttja and peat in the Allerød to loamy and sandy gyttja in the Younger Dryas. Previous radiocarbon dates at Ossendrecht of 11,240 ± 50 BP (c.13.2–13.1 ka cal BP) (GrN-12743) (Schwan, Reference Schwan1991) and 11,060 ± 60 BP (c.13.1–12.9 ka cal BP) (GrN-25940) for the Allerød interval and 10,460 ± 180 BP (c.12.8–11.8 ka cal BP) (GrN-25851) and 10,450 ± 190 BP (c.12.8–11.7 ka cal BP) (GrN-25852) for the Younger Dryas part of the organic layer (Kasse & Aalbersberg, Reference Kasse and Aalbersberg2019) support the biochronostratigraphic interpretation (Table 1). Luminescence dates of the river dune sediments overlying the peat in Ossendrecht (Fig. 3A: sandpit Boudewijn) range between 13.1 ± 0.9 ka (OS-4) and 11.5 ± 0.7 ka (OS-B) (Vandenberghe et al., Reference Vandenberghe2004) and indicate a Younger Dryas age. These results demonstrate that river dune deposition in the study area culminated in the second part of the Younger Dryas, which is characterised by the occurrence of Empetrum (Hoek, Reference Hoek1997a).
The age of river dune formation along the Scheldt River during the second half of the Younger Dryas is in agreement with previously reported findings upstream in the Scheldt valley (Bogemans & Vandenberghe, Reference Bogemans and Vandenberghe2011) and in the Meuse and Rhine valleys (De Jong & Zagwijn, Reference De Jong and Zagwijn1977a,b; Verbraeck, Reference Verbraeck1983; Teunissen, Reference Teunissen1990; Bohncke et al., Reference Bohncke, Vandenberghe and Huijzer1993; Janssens et al., Reference Janssens, Kasse, Bohncke, Greaves, Cohen, Wallinga and Hoek2012). In the Meuse valley, Bohncke et al. (Reference Bohncke, Vandenberghe and Huijzer1993) dated the transition from peat to river dune deposits to occur after 10,940 ± 60 BP (c.13.1–12.8 ka cal BP) (GrN-13379), based on a date of the top of the peat layer and associated decrease in pine pollen in Bosscherheide I (diagram #189 in Hoek, Reference Hoek1997b). During this transition, an alternation of organic layers and blown-in sand represents the first part of the Younger Dryas in Bosscherheide III (Bohncke et al., Reference Bohncke, Vandenberghe and Huijzer1993; diagram #190 in Hoek Reference Hoek1997b), dated to between 10,880 ± 50 BP (c.12.9–12.8 ka cal BP) (GrN-11569) and 10,500 ± 60 BP (c.12.7–12.5 ka cal BP) (GrN-11568), after which a thick and continuous layer of river dune sands was deposited (Table 1).
Along the northeastern margin of the river Meuse, Teunissen (Reference Teunissen1990) described Younger Dryas river dune sand blown into Wijchens Ven, an abandoned Allerød channel. A radiocarbon date just below this sand layer and above the decrease in pine pollen yields an age of 10,590 ± 90 BP (c.12.8–12.5 ka cal BP) (GrN-16817).
In the western part of the Rhine–Meuse delta a few radiocarbon dates from organic deposits and peat just below river dune deposits yield ages that support the palynological Allerød interpretation of the organic deposits. The dates of 10,970 ± 90 BP (c.13.1–12.8 ka cal BP) (GrN-6444) from Groot Ammers Ia (De Jong & Zagwijn, Reference De Jong and Zagwijn1977a,b; pollen diagram #233 in Hoek, Reference Hoek1997b) and 10,640 ± 80 BP (c.12.8–12.6 ka cal BP) (GrA-10921) from Polderweg (Hoek, unpublished observations) concur with the dates from the Meuse valley.
OSL dates from aeolian river dunes in the Oude IJssel valley of 10.8 ± 0.7 (NCL-4209160) and 11.0 ± 0.5 ka (NCL-4209159) also point to a late Younger Dryas age of river dune formation (Janssens et al., Reference Janssens, Kasse, Bohncke, Greaves, Cohen, Wallinga and Hoek2012).
It is generally assumed that changes in the thermohaline circulation or a combination of different forcing factors caused the abrupt onset of the Younger Dryas cold period (Bakke et al., Reference Bakke, Lie, Heegaard, Dokken, Haug, Birks, Dulski and Nilsen2009; Renssen et al., Reference Renssen, Mairesse and Goosse2015), although a strongly debated extraterrestrial impact has been suggested as well (Firestone et al., Reference Firestone, West, Kennett, Becker, Bunch, Revay, Schultz, Belgya, Kennett, Erlandson, Dickenson, Goodyear, Harris, Howard, Kloosterman, Lechler, Mayewski, Montgomery, Poreda, Darrah, Que Hee, Smith, Stich, Topping, Wittke and Wolbach2007; Van der Hammen & Van Geel, Reference Van der Hammen and Van Geel2008; Van Hoesel et al., Reference Van Hoesel, Hoek, Braadbaart, Van der Plicht, Pennock and Drury2012, Reference Van Hoesel, Hoek, Pennock and Drury2014). However, the dates of the onset of aeolian deposition show a delayed response of the sedimentary system to the Younger Dryas cooling. Although vegetation shows a rapid response to the Younger Dryas cooling (pine decline, increase in herbs), the sedimentary response shows a time lag between the climate forcing and start of aeolian deposition. Temperatures decreased and species composition changed at the start of the Younger Dryas (Isarin & Bohncke, Reference Bohncke1999); however, vegetation cover, determining sand supply, persisted in the first part of the Younger Dryas. In addition, the first part of the Younger Dryas was relatively wet as indicated by high lake levels (Bohncke & Wijmstra, Reference Bohncke and Wijmstra1988; Bos et al., Reference Bos, Bohncke and Janssen2006) which may have hampered sand transport. The second part of the Younger Dryas was dryer with lower lake levels (Hepp et al., Reference Hepp, Wüthrich, Bromm, Bliedtner, Schäfer, Glaser, Rozanski, Sirocko, Zech and Zech2019) and these drier conditions enhanced the transport of aeolian sand.
The end date of river dune formation in the study area is based on radiocarbon dates of organic material overlying the dunes on both the Brabantse Wal and in the polder area near site Zomerbaan (Fig. 3A; Table 1). Schwan (Reference Schwan1991) presented an uncalibrated radiocarbon date of 9050 ± 45 BP (c.10.3–10.2 ka cal BP) (GrN-12744) for the base of a Holocene peat layer overlying the river dunes at Ossendrecht. In the polder area west of the Brabantse Wal, the Late Glacial Scheldt valley is covered with Holocene deposits. Circa 2 km west of Ossendrecht, the organic deposition started at the Younger Dryas to Holocene transition with relatively high values of juniper (Juniperus) followed by birch (Betula), characteristic for the start of the Preboreal (De Jong & Zagwijn, Reference De Jong and Zagwijn1966, site Ossendrecht III, diagram #41 in Hoek Reference Hoek1997b). Bos et al. (Reference Bos, Huisman, Kiden, Hoek and Van Geel2005) reported Early Holocene lake deposits near Kreekrak (indicated with K in Fig. 3A) at c.14 m below surface. The pollen diagram shows that deposition started at the transition to the Holocene, supported by a radiocarbon date of 10,140 ± 60 BP (c.12.0–11.6 ka cal BP) (GrA-23029). According to Vos & Van Heeringen (Reference Vos and Van Heeringen1997: 82) the base of an organic deposit in a depression of the Pleistocene surface 0.5 km northwest of Woensdrecht (location W in Fig. 3A) was dated at 9610 ± 60 BP (c.11.2–10.8 ka cal BP) (GrN-16291), indicating an early Holocene age for the start of organic deposition following river dune formation (Table 1; Fig. 5).
The dates of organic material overlying the sandy Pleistocene surface and river dunes indicate that deflation of sand from the Scheldt valley and river-dune formation probably stopped, within age uncertainty, close to the Younger Dryas to Holocene transition (10,150 BP; 11,700 cal BP). However, aeolian deposition may have continued locally during the Preboreal at a decreasing rate (Hijma et al., Reference Hijma, Cohen, Hoffmann, Van der Spek and Stouthamer2009). This suggests a rapid response of the aeolian environment and a short time lag to the early Holocene climate warming. Although vegetation was sparse locally in the fluvial source area and in the aeolian river dune field, vegetation persisted regionally, although the species composition had changed during the Younger Dryas. At the start of the Holocene, aeolian transport stopped and the dunes were stabilised by rapid colonisation of the surface by the nearby vegetation (herbs and birch) (Hoek, Reference Hoek1997a, Reference Hoek2001). In addition to the vegetation response, the Scheldt River probably incised and channel pattern changed from braided to meandering at the Younger Dryas to Holocene transition (see above discussion on spatial distribution). The smaller floodplain width resulted in less wind fetch, and deflation from the floodplain was less effective. Therefore, sand supply in the source area stopped and river dune formation came to a halt. Luminescence dating of Younger Coversand II deposits in the eastern and southern Netherlands also demonstrated that aeolian deposition mainly occurred during the Younger Dryas stadial (12.9–11.7 ka cal BP) (Vandenberghe et al., Reference Vandenberghe, Derese, Kasse and Van den haute2013: YCII between 13.6 ± 1.1 ka and 12.2 ± 0.9 ka); Kasse et al., Reference Kasse2018: YCII between 12.6 ± 1.0 and 11.5 ± 0.9 ka).
Wind direction during the Younger Dryas
Younger Dryas wind direction is derived in this study from geomorphological analysis and by the study of sedimentary structures in aeolian deposits. The parabolic dune morphology of the river dunes on the Brabantse Wal indicates southwesterly winds during their formation (Fig. 3A). The south–north-oriented Weichselian Late Glacial Scheldt valley is located west of the dune field and is therefore the likely source of the aeolian sediment (source-bordering river dunes) (Fig. 8: level T3). Sand that was deflated from the valley accumulated partly west of the Brabantse Wal escarpment (Fig. 3B; site Zomerbaan). Most of the sand was blown over the escarpment and accumulated on the Brabantse Wal on the east bank of the Scheldt River (Fig. 4). Climate modelling experiments and proxy-based reconstructions also show strong westerly (winter) circulation during the Younger Dryas in northwestern Europe (Renssen et al., Reference Renssen, Lautenschlager and Schuurmans1996; Isarin et al., Reference Isarin, Renssen and Koster1997; Brauer et al., Reference Brauer, Haug, Dulski, Sigman and Negendank2008).
However, reconstruction of wind direction based on the dune morphology reflects the average direction of sand-transporting winds or the wind direction in the final stage of dune formation. The study of sedimentary structures in dune deposits provides additional information on the wind direction. However, reconstruction of wind direction based on sedimentary structures is often hampered by the dominance of horizontal bedding and low-angle cross bedding in dune deposits. Dune slipface cross bedding is rarely found and the few reported cases demonstrate a northerly to west-northwesterly wind direction (Kasse et al., Reference Kasse1995; Kasse & Aalbersberg, Reference Kasse and Aalbersberg2019), which is different from the morphology-derived wind direction.
Previous studies show that the lower part of the Younger Dryas source-bordering river dunes overlying the Allerød peat at Ossendrecht (sandpit Boudewijn) is characterised by wet aeolian deposition (Kasse, Reference Kasse1988; Vandenberghe et al., Reference Vandenberghe2004; Kasse & Aalbersberg, Reference Kasse and Aalbersberg2019). Crinkly lamination, involutions and adhesion ripple cross bedding testify to wet depositional conditions. Sand blown over the surface was trapped in areas with a high (ground) water table, forming adhesion wrats or adhesion ripples. Adhesion ripple cross lamination enables the reconstruction of former wind direction as adhesion ripples grow and migrate upwind (Hunter, Reference Hunter1980).
The analysis of adhesion ripple sets on five lacquer peels from the river-dune deposits at Ossendrecht reveals a dominance of southerly to westerly winds (Figs 10 and 11). It should be realised that this direction does not necessarily indicate the dominant wind direction during the Younger Dryas. Sand is transported during high wind speeds and therefore the derived wind direction is indicative for strong sand-transporting winds. Furthermore, the wind direction is derived from adhesion ripple structures that form on a wet or water-saturated surface (Hunter, Reference Hunter1980). During the second part of the Younger Dryas, wet surface conditions most likely only occurred during the summer season when temperatures were around 14°C and the soil was thawed (Bohncke, Reference Bohncke1993; Isarin & Bohncke, Reference Bohncke1999). Low mean winter temperatures lower than −10°C resulted in a deeply frozen soil and a freeze-dry surface. Therefore, we postulate that the adhesion-ripple based southwesterly wind directions reflect summer storm-wind events. Winter storm events, possibly from other directions, may have formed the horizontally laminated and low-angle cross-bedded sets and rare dune slipface cross bedding.
Conclusions
The source-bordering Younger Dryas aeolian dune field along the Late Glacial Scheldt course in the southwestern Netherlands was investigated by geomorphological analysis, coring, pollen analysis, dating and sediment interpretation.
Our study shows an extensive dune field on the east bank of the Scheldt north of Antwerp and in the subsurface of the polder area west of the Brabantse Wal escarpment. The top of the Pleistocene surface reveals a drowned Late Glacial terraced landscape. Reach-specific braiding, induced by a higher gradient of the Scheldt in comparison to upstream regions, enabled deflation from the braid plain and accumulation in source-bordering river dunes east of the valley.
The period of dune formation occurred during the second part of the Younger Dryas stadial. The onset of aeolian deposition shows a delayed response to the Younger Dryas cooling, while the end of aeolian deposition reveals limited time lag to the Holocene warming.
A southwesterly wind direction is established based on the parabolic dune morphology of the dune field. For the first time, Younger Dryas wind directions are reconstructed based on adhesion ripple cross-laminated sets. Sand-transporting south-southwesterly winds were important during the Younger Dryas, most likely during summer.
Acknowledgements
Dr F. Busschers and an anonymous reviewer are thanked for constructive comments that improved the manuscript.