Introduction
The Lower Rhine Basin extends from NW Germany through to the SE Netherlands, and is an embayment (Lower Rhine Embayment) which formed as a result of basin subsidence and related marine transgressions in Oligocene times (R. Teichmüller, Reference Teichmüller1958; Zagwijn & Hager, Reference Zagwijn and Hager1987; Hager, Reference Hager1993; Schäfer et al., Reference Schäfer, Hilger, Gross and von der Hocht1996; Klett et al., Reference Klett, Eichhorst and Schäfer2002; Becker & Asmus, Reference Becker and Asmus2005; Schäfer et al., Reference Schäfer, Utescher, Klett and Valdivia-Manchego2005; Schäfer & Utescher, Reference Schäfer and Utescher2014), and within which sub- to supratidal (Longhitano et al., Reference Longhitano, Mellere, Steel and Ainsworth2012) sediments were deposited (Schäfer et al., Reference Schäfer, Hilger, Gross and von der Hocht1996). The basin is a rift, extending into the Rhenish Massif and forming the NW part of the NW European Cenozoic Basin (Ziegler, Reference Ziegler1992, Reference Ziegler1994; Houtgast & van Balen, Reference Houtgast and van Balen2000; Klett et al., Reference Klett, Eichhorst and Schäfer2002; Sissingh, Reference Sissingh2003; Ziegler & Dèzes, Reference Ziegler and Dèzes2007; Fig 1). During the late early and middle Miocene, warm temperate climate conditions in a highstand systems tract (but at a point where eustatic sea level had already begun to fall and the highstand system sediment surface was exposed, but immediately prior to the erosion surface marking the falling-stage systems tract; Utescher et al., Reference Utescher, Ashraf, Dreist, Dybkjaer, Mosbrugger, Pross and Wilde2012; Schäfer & Utescher, Reference Schäfer and Utescher2014, fig. 13) led to the formation of extensive coastal swamps and thick (up to 300 m) peat accumulations that formed the present-day, up to 100 m thick Main Seam of the Ville Formation (Fig. 2). Peat formation was interrupted by the deposition of clastic sediments (Frimmersdorf and Neurath sands) as a result of repeated marine transgressions, which subdivided the Main Seam into three smaller seams (the Morken, Frimmersdorf and Garzweiler lignite seams; Fig. 2). Active exploitation of these deposits within the Lower Rhine Basin provides an excellent opportunity to examine the sedimentary succession (e.g. Petzelberger, Reference Petzelberger1994; Schäfer et al., Reference Schäfer, Hilger, Gross and von der Hocht1996; Klett et al., Reference Klett, Eichhorst and Schäfer2002; Schäfer & Utescher, Reference Schäfer and Utescher2014). The present study focuses on the Neurath Sand, a clastic unit which separates the Frimmersdorf and Garzweiler seams. The aim of the study is the three-dimensional reconstruction of the depositional setting of the Neurath Sand, integrating both detailed sedimentary profiles and mining-industry well logs.

Fig. 1. A structural map of the NW European Cenozoic Rift System. The Lower Rhine Basin is located at the northern end of the Upper Rhine Graben, NW of the Rhenish Triple Junction in the area of Frankfurt (Schumacher, Reference Schumacher2002; Sissingh, Reference Sissingh2003; Rasser et al., Reference Rasser, Harzhauser, Anistratenko, Anistratenko, Bassi, Belak, Berger, Bianchini, Cičić, Ćosović, Doláková, Drobne, Filipescu, Gürs, Hladilová, Hrvatović, Jelen, Kasiński, Kováč, Kralj, Marjanac, Márton, Mietto, Moro, Nagymarosy, Nebelsick, Nehyba, Ogorelec, Oszczypko, Pavelić, Pavlovec, Pavšič, Petrová, Piwocki, Poljak, Pugliese, Redžepović, Rifelj, Roetzel, Skaberne, Sliva, Standke, Tunis, Vass, Wagreich and Wesselingh2008). BG = Bresse Graben, HG = Hessen Graben, LG = Leine Graben, RG = Rhône Graben.

Fig. 2. Stratigraphic log of the Lower Rhine Basin (modified after Klett et al., Reference Klett, Eichhorst and Schäfer2002; Schäfer et al. Reference Schäfer, Utescher and Mörs2004, Reference Schäfer, Utescher, Klett and Valdivia-Manchego2005; Schäfer & Utescher Reference Schäfer and Utescher2014). The lithostratigraphic log based on two well logs (SNQ 1, Erft Block, and Efferen, Köln Block; RWE Power AG) represents the stratigraphy at the centre of the Erft Block; lithostratigrapical code established by Schneider & Thiele (Reference Schneider and Thiele1965). Biostratigraphical ages (Ma) on the left after Berggren et al. (Reference Berggren, Kent, Swisher and Aubry1995); cycle ages (Ma) on the right after Haq et al. (Reference Haq, Hardenbol and Vail1987, Reference Haq, Hardenbol and Vail1988) and Hardenbol et al. (Reference Hardenbol, Thierry, Farley, Jacquin, de Graciansky and Vail1998).
Geological framework
The extensional Lower Rhine Basin formed in early Oligocene times (Ziegler, Reference Ziegler1994; Klett et al., Reference Klett, Eichhorst and Schäfer2002; van Balen et al., Reference Van Balen, Houtgast and Cloetingh2005) as the NW extension of the European Cenozoic Rift System (Ziegler, Reference Ziegler1992, Reference Ziegler1994; Sissingh, Reference Sissingh2003, Reference Sissingh2006; Ziegler & Dèzes, Reference Ziegler and Dèzes2007; Fig. 1), with subsidence ongoing throughout the Neogene (Schäfer et al., Reference Schäfer, Utescher, Klett and Valdivia-Manchego2005). Extension was initiated along the NW margin of the Rhenish Massif, where several NW–SE-striking faults formed, which are still active today (Ziegler, Reference Ziegler1992; Hinzen, Reference Hinzen2003; Sissingh, Reference Sissingh2003, Reference Sissingh2006; Reicherter et al., Reference Reicherter, Froitzheim, Jarosiński, Badura, Franzke, Hansen, Hübscher, Müller, Poprawa, Reinecker, Stackebrandt, Voigt, Eynatten and Zuchiewicz2008; Schäfer & Utescher, Reference Schäfer and Utescher2014; Grützner et al., Reference Grützner, Fischer and Reicherter2016). These faults separate the basin into graben and horst structures (Fig. 3). Three tectonic units characterise the SE part of the Lower Rhine Basin. From E to W these are the Köln, Erft and Rur blocks, separated by the Erft and Rur faults, respectively. Towards the NW, three additional blocks occur, namely the Peel, Venlo (location of the Garzweiler open-cast mine) and Krefeld blocks. The Viersen Fault forms the contact between the latter two, while the Tegelen Fault separates the Venlo Block from the Peel Block (Fig. 3). The faults separating the individual blocks generally dip steeply (70–80°) to the SW (Schäfer et al., Reference Schäfer, Utescher, Klett and Valdivia-Manchego2005). Subsidence across the region is variable, with up to 2000 m recorded for the Peel Block in the Roer Valley Graben, while the Erft Block subsidence has been measured at 1300 m (Klett et al., Reference Klett, Eichhorst and Schäfer2002).

Fig. 3. A structural map of the Lower Rhine Basin. The six main tectonic blocks (Rur, Erft and Köln blocks in the SE; Peel, Venlo and Krefeld blocks in the NW) are bounded by the Rur (RF), Erft (EF), Peel Boundary (PF), Tegelen (TF) and Viersen faults (VF), as well as the fault system bounding the Jackerath Horst (JH). The Rur Block extends into the Roer Valley Graben in the NW. G = Garzweiler open-cast mine; H = Hambach open-cast mine; I = Inden open-cast mine; pink line: Germany–Netherlands–Belgium border. The two red lines mark the position of two cross sections (A–B and C–D), which are shown in Figure 4. Modified after Geluk et al. (Reference Geluk, Duin, Dusar, Rijkers, van den Berg and van Rooijen1994), Schäfer et al. (Reference Schäfer, Hilger, Gross and von der Hocht1996), Klett et al. (Reference Klett, Eichhorst and Schäfer2002) and Schäfer & Utescher (Reference Schäfer and Utescher2014).
The Peel Block, as well as the Rur Block in the German part of the basin, are structurally part of the Roer Valley Graben (Fig. 3; Houtgast & van Balen, Reference Houtgast and van Balen2000; Michon et al., Reference Michon, van Balen, Merle and Pagnier2003), an extended rift system in the Netherlands (Geluk et al., Reference Geluk, Duin, Dusar, Rijkers, van den Berg and van Rooijen1994; Grützner et al., Reference Grützner, Fischer and Reicherter2016). Formerly, the terms Roer Valley Graben and Lower Rhine Basin were used to refer to the same tectonic feature, and denoted either the German part (Lower Rhine Basin), or its NW extension in the Netherlands (Roer or Rur Valley Graben; Ziegler, Reference Ziegler1992; Sissingh, Reference Sissingh2003). Alternatively, the Roer Valley Graben has been interpreted as the central graben structure of the Lower Rhine Basin (e.g. Vanneste et al., Reference Vanneste, Meghraoui and Camelbeeck1999). However, in this study the terms are used sensu Geluk et al. (Reference Geluk, Duin, Dusar, Rijkers, van den Berg and van Rooijen1994), Houtgast & van Balen (Reference Houtgast and van Balen2000) and Michon et al. (Reference Michon, van Balen, Merle and Pagnier2003), and thus refer to two adjacent and structurally interlinked tectonic grabens.
As noted above, subsidence within the Lower Rhine Basin allowed the Cenozoic-age North Sea to transgress the region. In the resulting shallow-marine embayment (Schäfer et al., Reference Schäfer, Hilger, Gross and von der Hocht1996), a clastic succession of sub- to supratidal sediments was deposited during Oligocene times (Fig. 2). Sea-level highstand in the late early and middle Miocene, coupled with warm temperate climatic conditions (Zagwijn & Hager, Reference Zagwijn and Hager1987; Mosbrugger et al., Reference Mosbrugger, Utescher and Dilcher2005; Utescher et al., Reference Utescher, Mosbrugger, Ivanov and Dilcher2009), facilitated the development of extensive coastal swamps with associated peat bogs, marshes and bush forests (Mosbrugger et al., Reference Mosbrugger, Gee, Belz and Ashraf1994). Ongoing basin subsidence, combined with compaction of the peats, resulted in the formation of thick (up to 100 m) lignite accumulations (i.e. Main Seam) in the SE Lower Rhine Basin (Hager, Reference Hager1986; Schäfer et al., Reference Schäfer, Utescher, Klett and Valdivia-Manchego2005). To the NW, the Main Seam is separated into three subordinate seams: the Morken, Frimmersdorf and Garzweiler seams (=Ville Formation of Early to Mid-Miocene age). These are separated from one another by the marine sediments of the Frimmersdorf and Neurath sands, respectively. These sands were deposited as a result of short-lived marine transgressions during the Miocene which resulted in the deposition of thick (up to 100 m) marine beds along the NW rim of the Miocene-age coastal mires. Following regression of the North Sea in late Miocene to Pliocene times, meandering and, subsequently, braided river systems developed on top of the marine and paralic sediments (Klett et al., Reference Klett, Eichhorst and Schäfer2002; Schäfer & Utescher, Reference Schäfer and Utescher2014).
Regional distribution of the Neurath Sand in the Lower Rhine Basin
The Neurath Sand is one of two shallow-marine sand bodies within the NW part of the Lower Rhine Basin (the other is the Frimmersdorf Sand), which separate the Main Seam into three subordinate seams (Fig. 4). Based on a sequence stratigraphical model, deposition of the Neurath Sand was continuous from 13.8 to 12.7 Ma, i.e. over a time span of 1.1 Ma (Fig. 2; ages after Haq et al., Reference Haq, Hardenbol and Vail1987, Reference Haq, Hardenbol and Vail1988; Berggren et al., Reference Berggren, Kent, Swisher and Aubry1995; Hardenbol et al., Reference Hardenbol, Thierry, Farley, Jacquin, de Graciansky and Vail1998).

Fig. 4. Cross sections A–B (NW–SE) and C–D (W–E; positions in the Lower Rhine Basin marked in Fig. 3). The Main Seam (MS) of the Middle Miocene-age Ville Formation is separated by transgressive sand units into three subordinate units: the Morken (M), Frimmersdorf (F) and Garzweiler seams (G). The Neurath Sand (NS) terminates to the SE and W, and increases in thickness to the NW. Modified after Becker & Asmus (Reference Becker and Asmus2005).
Two cross sections (NW–SE- and W–E-oriented; Fig. 4) across the Lower Rhine Basin, and integrated with comprehensive well log data (Schäfer & Utescher, Reference Schäfer and Utescher2014), allow the Neurath Sand to be traced laterally across the basin, while also allowing its thickness variability to be determined. It would appear that the morphology of the Neurath Sand is that of an oval lens oriented NW–SE. The maximum thickness of the Neurath Sand is up to 100 m on the Venlo and Erft blocks (Fig. 4). Landward (i.e. towards the SE, and the margin of the Rhenish Massif in the W; Fig. 4), the Neurath Sand thins out. This thinning is accompanied by a change in lithology (from sand to muddy sand, and eventually mud; Boersma, Reference Boersma1991). The maximum SE extent of the sand-rich deposits is exposed in the Hambach open-cast mine (Fig. 5). Towards the region occupied by the coeval open sea (i.e. to the NW), the thickness of the Neurath Sand increases to c. 200 m, and it interdigitates with the shallow-marine Breda Formation (Burdigalian to Mid-Tortonian age), an up to 700 m thick unit covering most of the Netherlands (Wong et al., Reference Wong, Parker and Horst2001; Verbeek et al., Reference Verbeek, de Leeuw, Parker and Wong2002; Munsterman & Brinkhuis, Reference Munsterman and Brinkhuis2004).

Fig. 5. The maximum extent of the Neurath Sand as exposed in the Hambach open-cast mine (1998).
Methods
In the Garzweiler open-cast mine (located at the NW end of the mining region extending along the W margin of the Köln Block, i.e. the Ville Ridge, and including the older Ville, Frechen, Bergheim and Fortuna mines; Fig. 3), mining activity provided access to a vertical section prepared for this study by RWE Power AG. Three freshly cut field sections along the western exploitation ramp were measured. Profile 1, with a total length of 67 m, covered the entire Neurath Sand, from the underlying Frimmersdorf Seam through to the overlying Garzweiler Seam. Parallel profiles, located 200 m from each other along a N–S axis, were measured along the active exploitation ramp. Neither profile 2 (35 m) nor profile 3 (24 m) extended to the base of the Neurath Sand, due to the working level of the lignite in this part of the open-cast mine. The three profiles allowed a three-dimensional facies analysis of the Neurath Sand to be carried out, as well as detailed examination of the sediments (e.g. grain size, sorting, sedimentary structures), and the integration of ichnological data. Lignite-derived humic acids have led to the dissolution of calcareous tests and shells within the sands, so the only fossils present within the Neurath Sand are ichnofossils and wood/lignite fragments. Laboratory investigations (e.g. grain-size analysis) were used to support the field observations and to provide additional information (e.g. petrological analysis). This latter analysis focused on the examination of dark-coloured sand clasts, which had been observed in all three measured profiles. These were sampled (profile 2 (6.0–6.5 m); see below) and hardened with epoxy resin, with subsequent thin sections (n = 10) being used to determine lithic fragment frequency and composition.
Sedimentary facies and facies analysis
Six sediment facies have been recognised in the Neurath Sand and the overlying Garzweiler Seam, ranging from muddy and coarse- to fine-grained sands through to lignite (Table 1). These are described in detail below.
Table 1. Sedimentary facies of the Neurath Sand and the overlying Garzweiler Seam, Garzweiler open-cast mine.

Muddy sands with chert-pebble lag (Smu)
Description
Dark grey-coloured, fine- to medium-grained sands with up to 15% mud which contain clasts of mud, wood and lignite (up to 2 cm), concentrated near the bed base. The base of the facies is characterised by a chert-pebble layer (ranging in thickness from a single pebble to 0.75 m; Fig. 6a) which lacks grading or structure. The matrix of the chert-pebble layer is white to light grey in colour, changing upsection into the more characteristic darker-coloured sands. Bed thicknesses range from 0.5 to 2 m. The beds are internally non-stratified and are not graded. The ichnofossils include simple vertical and unbranched burrows of Skolithos linearis (up to 20 cm long; Seilacher, Reference Seilacher1964; Alert, Reference Alert1974; Hiscott et al., Reference Hiscott, James and Pemberton1984; Howard & Frey, Reference Howard and Frey1984; MacEachern et al., Reference MacEachern, Bann, Gingras, Zonneveld, Dashtgard, Pemberton, Knaust and Bromley2012) and subvertical unbranched burrows with spreiten (Teichichnus ichnosp.; Fig. 6b; cf.Crimes, Reference Crimes, Crimes and Harper1977), as well as unbranched, (sub)horizontal Planolites ichnosp (cf.Curran & Frey, Reference Curran and Frey1977). The ichnofossils are distributed throughout the beds.

Fig. 6. Facies characteristics of the Neurath Sand. (A) Chert-pebble bed at the base of the muddy sand (Smu) lithofacies; (B) Teichichnus ichnosp. in the muddy sand (Smu) lithofacies; (C) root horizons extending down into the Neurath Sand from the overlying Garzweiler Seam; (D) Ophiomorpha ichnosp. within fine-grained sands of the unstratified fine sand (Sf-u) lithofacies; (E) black sand-clast bed in profile 3 at c. 11 m; (F) microphotograph (crossed polars) of a black sand clast from profile 2 (c. 6 m in profile), showing mainly quartz grains, and dark, opaque humic substances occupying the interstitial pore volume.
Interpretation
The relatively fine grain size and the presence of up to 15% of mud would suggest deposition within a relatively low- to moderate-energy environment. The chert-pebble bed at the base of this facies (Fig. 6a), however, indicates an initial high-energy transport regime, and represents a lag deposit. The lack of any internal structure within the muddy sands may result from (a) rapid and turbulent flows, (b) bioturbation or (c) remobilisation of sediments due to liquefaction. While the facies is bioturbated, the bioturbation is discrete and the facies does not have the mottled appearance associated with high degrees of bioturbation (e.g. Taylor & Goldring, Reference Taylor and Goldring1993; Bromley, Reference Bromley1996; Tonkin et al., Reference Tonkin, McIlroy, Meyer and Moore-Turpin2010). Additionally, there is no evidence of li-quefaction (e.g. dish or pillar structures). Another possibility is that the lamination is present but not visible as a result of the preparation of the section by the bucket wheels of the front-wheel loaders cutting the access ramps.
Skolithos linearis is generally found in lower intertidal to shallow subtidal settings (above fairweather wave-base) with moderate- to high-energy conditions (MacEachern et al., Reference MacEachern, Bann, Gingras, Zonneveld, Dashtgard, Pemberton, Knaust and Bromley2012). However, Skolithos ichnosp. has also been observed in deeper environments, e.g. the transition zone on the shelf (Desjardins et al., Reference Desjardins, Mángano, Buatois and Pratt2010) or deep-sea sand fans (Crimes, Reference Crimes, Crimes and Harper1977; Uchman & Demircan, Reference Uchman and Demircan1999). The occurrence of Teichichnus ichnosp. (Fig. 6b) is restricted to muddy substrates in low-energy environments, which are permanently water-covered (i.e. below low-tide line; Seilacher, Reference Seilacher1964; Pemberton & Frey, Reference Pemberton and Frey1984; Frey, Reference Frey1990; MacEachern et al., Reference MacEachern, Bann, Gingras, Zonneveld, Dashtgard, Pemberton, Knaust and Bromley2012). Planolites ichnosp. is a facies-crossing ichnospecies and so does not provide any information as to specific water depths or energy milieus.
Fine-grained sands (Sf)
Description
Fine-grained sands (<10% of mud, <3% of medium- and coarse-grained sand) which are non-stratified (Sf-u), planar (Sf-p), cross- or trough cross-laminated (Sf-c). Within the latter two, fine-grained sands comprising very thin (1–2 mm) sand–mud laminae were observed. Non-stratified sands may contain glauconite. Along the bases of some of the individual cross-laminae, thin lags (generally one to two grains thick, and medium- to coarse-grained sand) may occur. Bed thicknesses range from 0.2 to 4.0 m for Sf-p down to 0.2 to 3.5 m for Sf-c and 0.5 to 1.0 m for Sf-u. Root horizons, where present, are restricted to the top of individual beds (Fig. 6c). Non-stratified and planar-laminated fine-grained sands are sporadically black and frequently comprise wood fragments.
Frequently observed Ophiomorpha ichnosp. (restricted to profile 1; Fig. 6d) are predominant within the non-stratified sands (Sf-u), but were also noted from one of the trough cross-laminated sand beds (Sf-c). The fine-grained sand beds can contain clasts of mud (up to 20 cm), lignite or wood (up to 30 cm, but generally smaller) that are either concentrated near the bed bases or distributed throughout the bed.
Within this facies (but also within other facies; see below) are beds, 20–50 cm thick, of black fine-grained sand which are overlain by thin beds (20–30 cm) comprising fine-grained, black sand clasts. The sand-clast beds (Fig. 6e) are covered by trough cross-laminated beds (up to 20 cm thick, containing wood fragments), topped by symmetrical ripples (2–7 cm high). The black sand clasts comprise mainly monocrystalline quartz (Qm, 47.51%; Fig. 6f; Table 2), showing undulose extinction and dust rims (indicative of phases of diagenetic crystal growth). Polycrystalline quartz (Qp; 16.69%) is also present. Both Qm and Qp may include mafic inclusions (often altered to kaolinite (3.97%) or replaced by humic gel particles) either within the crystals or along their margins. K-feldspar (5.74%) is also present, usually as microcline or perthite, while rare plagioclase (1.96%) also occurs. Muscovites (on average, 0.18%), where present, have an extinction colour suggesting they were originally biotite. Lithic fragments include quartz phyllites, phyllites, quartzites (8.91%), as well as rare mafic (0.83%, including volcanics, mostly weathered to kaolinite) and carbonate (0.1%) clasts. Possible glauconite was also noted (1.37%). The sand clasts can be classified as quartz-rich feldspathic litharenites (after Folk Reference Folk1974; Fig. 7; Table 2), with little variation between the various profiles or within an individual profile. The interstitial pore volume of the sand grains is filled with dark-coloured, opaque humic substances (Fig. 6f).
Table 2. Modal analysis of the thin sections (n = 10) of one black sand clast (profile 2).

Q = mono- and polycrystalline quartz; F = K-feldspar, plagioclase and feldspar/quartz aggregates; R = lithic rock fragments including heavy minerals, micas and chert.

Fig. 7. Modal analysis of thin sections (n = 10) of one black sand clast (profile 2) (after Folk, Reference Folk1974; Graham & Midgley, Reference Graham and Midgley2000). These data are from the same clast as illustrated in Figure 6e. F = feldspar, Q = quartz, R = rock fragments.
Interpretation
The Sf facies contains three subfacies, as well as sand–mud laminae and black sands. The fine-grained nature of the facies would suggest deposition under low-energy conditions, although the sedimentary structures (e.g. Sf-p, Sf-c) would suggest periods of higher energy. The ichnofossil-rich beds tend to coincide with the occurrence of the structureless fine-grained sands (Sf-u), suggesting that the lack of structure is related to intense bioturbation (up to BI 4–6; Tucker, Reference Tucker2003) rather than an absence of sedimentary structures per se.
The cross-laminated sands (Sf-c) were deposited from migrating ripples or dunes indicative of higher wave energy, as well as tidal, wave or wind-induced currents (Dabrio, Reference Dabrio1982; Masselink & Anthony, Reference Masselink and Anthony2001; Reynaud & Dalrymple, Reference Reynaud and Dalrymple2012; Hunt et al., Reference Hunt, Bryan and Mullarney2015). The parallel-laminated sands (Sf-p) were also deposited under low-energy conditions, most probably aeolian (Otvos, Reference Otvos2000; Collinson et al., Reference Collinson, Mountney and Thomson2006). However, the planar-laminated fine-grained sands can also include interbedded sand–mud laminae, which have been interpreted as tidal rhythmites (Tessier et al., Reference Tessier, Archer, Lanier and Feldman1995; Reesink & Bridge, Reference Reesink and Bridge2007; Bungenstock & Schäfer, Reference Bungenstock and Schäfer2009). Such laminae record the passage of tidal currents alternating with periods of tidal slack water (Visser, Reference Visser1980; Reesink & Bridge, Reference Reesink and Bridge2007). The occurrence of two coupled mud laminae alternating with thin sand laminae could be interpreted as ebb and flood cycles, with such coupled mud laminae generally restricted to environments below low-tide level (Hovikoski et al., Reference Hovikoski, Räsänen, Gingras, Roddaz, Brusset, Hermoza, Pittman and Lertola2005).
The black clasts were indurated as a result of their infiltration by black humic gels most probably derived from exposed and reworked lignites as well as from nearby coastal mires. Alteration, and related induration, was initiated by the passage of low-pH humic acids, which allowed the sands to be eroded from the underlying black sand, and redeposited as lags. The humic gels are preserved as dark-brown films surrounding the sand grains, while organic substances are preserved as black angular fragments in the pore spaces (Fig. 6f).
Wood fragments indicate the input of organic material from nearby coastal mires, whereas black sands at the top of the Neurath Sand have been infiltrated by humic gels from the overlying Garzweiler lignite seam. Root horizons within the fine-grained sands would appear to migrate downwards from the overlying lignite seams. Such concentrations of root horizons would suggest the former presence of a well-vegetated horizon, and one located in a continental/near-coastal setting (Darscheid, Reference Darscheid1981). This horizon would thus represent the period of initial colonisation by plants following the phase of marine regression. The black sand beds, frequently covering the rooted horizons, suggest an infiltration of humic gels from the overlying lignite deposits.
Ophiomorpha ichnosp. (Fig. 6b) occurs in a range of energy settings, from sand-rich, moderate- to high-energy, to muddy, low-energy marine environments. It is generally found in marine environments ranging from lower intertidal through to shoreface, and above storm wave-base (Seilacher, Reference Seilacher1964; Hiscott et al., Reference Hiscott, James and Pemberton1984; Howard & Frey, Reference Howard and Frey1984; MacEachern et al., Reference MacEachern, Bann, Gingras, Zonneveld, Dashtgard, Pemberton, Knaust and Bromley2012).
Fine- to medium-grained sands (Sfm)
Description
The fine- to medium-grained sands (Sfm) comprise three subfacies, including non-stratified (Sfm-u; 0.6–1.0 m thick), planar (Sfm-p; 0.3–1.0 m) and cross-laminated/bedded (Sfm-c; 0.3–2.0 m) sand beds. Lamination within the latter beds, in particular, results from the alternation of fine- with medium-grained sand laminae. Within individual beds, planar-bedded, fine- to medium-grained, sand alternations have been observed. These beds may show a fining upward. Within the trough cross-laminated sand beds (Sfm-c), mud–sand laminae may occur. Clasts of mud, wood and lignite, as well as roots, are very common within both the non-stratified and the planar-laminated sands.
Interpretation
Fine- to medium-grained sands indicate deposition within a moderate-energy setting, with the fine- and medium-grained sand alternations suggesting regularly chan-ging energy levels. Within the planar bedded sands, the fining-up would suggest waning energy levels. Clasts of lignite and wood within the non-stratified and planar-laminated sand possibly indicate seaward transport of organic matter from the adjacent peat/swamp environment, as well as the reworking of laterally exposed lignite deposits (e.g. peat/lignite accumulations of the present-day Frimmersdorf Seam). Rhizoturbated sediments, as described above, indicate the presence of a vegetation cover overlying the sand deposits.
Cross- and trough cross-lamination would suggest that these beds were deposited in a depositional setting controlled by wave or tidal currents. Such a setting would be supported by the presence of tidal bundles (sand–mud laminae) within the facies.
Medium-grained sands (Sm)
Description
This lithofacies comprises (trough or planar) cross-laminated/bedded sand beds (Sm-c; 1.0–1.5 m thick), as well as planar-laminated sand beds (Sm-p; 0.5–2.5 m thick). Planar-laminated, medium-grained sand beds (rarely black) may show evidence of upward fining within individual beds. In addition, lamina thickness decreases within individual beds. The cross-laminated/cross-bedded sand beds may contain mud clasts (up to 2 cm), as well as rare coarse-grained laminae.
Medium-grained, cross-laminated/bedded sand units also contain beds with concentrations of black sand clasts. These sand clasts are surrounded by a matrix of grey medium-grained sand, which also contains concentrations of coarse-grained sand (concentrated at the base of the clast bed), small chert pebbles (up to 0.5 cm in diameter and concentrated within the interstices between the black sand clasts) and wood fragments (up to 1 cm; also concentrated within the interstices). These sand-clast beds have a lateral extent of up to 4 m.
Interpretation
The medium-grained sands (Sm) were deposited in a setting characterised by moderate to high energy. Planar-laminated, medium-grained sands (Sm-p) are interpreted as high-energy laminites, which are characteristic of the swash zone. The presence of cross-laminated, medium-grained sands (Sm-c) would suggest deposition in an environment influenced by wave and tidal activity (de Raaf & Boersma, Reference De Raaf and Boersma1971; Hori et al., Reference Hori, Saito, Zhao, Cheng, Wang, Sato and Li2001; Yang et al., Reference Yang, Dalrymple and Chun2005) as well as the migration of large-scale dunes (Reynaud & Dalrymple, Reference Reynaud and Dalrymple2012), thus suggesting deposition within a ridge-and-runnel system (Dabrio, Reference Dabrio1982; Masselink & Anthony, Reference Masselink and Anthony2001). Such systems form as a result of the high wave energy within the breaker zone (dissipative shoreline; e.g. Stive et al., Reference Stive, Aarninkhof, Hamm, Hanson, Larson, Wijnberg, Nicholls and Capobianco2002; Aagaard & Hughes, Reference Aagaard and Hughes2006). The ridges comprise trough cross-laminated dunes which are oriented parallel to the coastline. However, due to wave activity, tidal currents or the influence of an estuarine system, dunes may also be orientated perpendicular to the shoreline (Dalrymple et al., Reference Dalrymple, Mackay, Ichaso, Choi, Davis and Dalrymple2012). On top of these dunes, wave activity can lead to the deposition of high-energy parallel, or planar cross-lamination (Schäfer, Reference Schäfer2010). Horizontal surfaces between individual trough cross-laminated sections formed as a result of the erosion of the upper part of dunes, followed by the build-up of new sand dunes on top (i.e. compound dunes; Reynaud & Dalrymple, Reference Reynaud and Dalrymple2012). Mud clasts were probably eroded from adjacent muddy tidal flats (Fan, Reference Fan, Davis and Dalrymple2012), and these were subsequently deposited on the lee sides of the sand dunes/ridges/mega-ripples. The coarser-grained laminae within the Sm-c are probably indicative of periods of increased wave or tidal energy.
Sand dunes in such ridge-and-runnel systems are often cut by offshore-directed rip currents (Aagaard et al., Reference Aagaard, Greenwood and Nielsen1997). The characteristic, laterally restricted black sand-clast beds with erosive lower boundaries may have been the result of these high-velocity currents, which erode small channels into the trough cross-laminated ridges and dunes (Dabrio, Reference Dabrio1982; Masselink & Anthony, Reference Masselink and Anthony2001; Hughes, Reference Hughes, Davis and Dalrymple2012). Within the sand-clast beds, coarse-grained sands and chert pebbles embedded into the matrix of medium-grained sands are indicative of periods of higher-velocity currents. The sand clast beds are covered by small-scale ripple marks, indicating a decrease in current velocity.
Rip currents, such as those presumed responsible for the generation of the black sand-clast beds, have been regarded as an important pathway for the exchange of water and sediment between the surf zone and the inner shelf environments (Aagaard et al., Reference Aagaard, Greenwood and Nielsen1997; Haller, Reference Haller2002; Castelle & Coco, Reference Castelle and Coco2012). The velocities within rip currents increase during ebb tides (Aagaard et al., Reference Aagaard, Greenwood and Nielsen1997) and therefore may have been responsible for the seaward-directed transport of wood fragments, peat or lignite clasts, as well as chert pebbles.
Coarse- to medium-grained sands (Sc)
Description
Coarse- to medium-grained sand beds, which range in thickness from 0.3 to 2 m, are exclusively cross-laminated/bedded (Sc-c), and may be graded (fining up). They contain evidence of both planar cross- and trough cross-lamination/bedding, although the former is more common. Individual cross-laminae within this section may contain fine-grained, organic-material-rich and black sands. The cross-laminated beds are internally bounded by horizontal surfaces, separating the individual sets of cross-lamination and bedding. Wood fragments (branches and roots, up to 1 cm in diameter), where present, have been deposited parallel to these horizontal, erosive surfaces, as well as parallel to the trough cross-laminae. Clasts also present within this facies include mud and lignite fragments. Up to 2 cm thick mud beds, covering the trough cross-laminated, coarse- to medium- grained sand beds were also present.
Interpretation
This lithofacies was probably deposited in a higher-energy environment in a shallow-marine setting, influenced by waves and tidal currents (de Raaf & Boersma, Reference De Raaf and Boersma1971; Hori et al., Reference Hori, Saito, Zhao, Cheng, Wang, Sato and Li2001; Yang et al., Reference Yang, Dalrymple and Chun2005; Reynaud & Dalrymple, Reference Reynaud and Dalrymple2012). The planar cross- and trough cross-laminated/bedded beds are indicative of large migrating compound dunes (Collinson et al., Reference Collinson, Mountney and Thomson2006; Reynaud & Dalrymple, Reference Reynaud and Dalrymple2012), and therefore may also be related to the development of a ridge-and-runnel system (see above). The lignite and wood fragments were most probably derived from the erosion of adjacent coastal mires.
Lignite (L)
Description
The lignite lithofacies comprises thick (up to 10 m) lignite beds. The beds may contain large wood fragments (branches, tree trunks, up to 4 m in length and 1 m in diameter), which were deposited parallel to the bedding direction (i.e. possibly not in situ), as well as (sub)vertically oriented wood remains (probably in situ). Root horizons, extending downward from the lignites into the underlying sands, are common.
Interpretation
The lignite deposits form part of the Garzweiler Seam, the uppermost of the three seams of the Ville Formation (Hager, Reference Hager1993; Schäfer et al., Reference Schäfer, Utescher and Mörs2004; Schäfer & Utescher, Reference Schäfer and Utescher2014). They were deposited within a coastal mire adjacent to the Serravallian-age North Sea. The mire comprised areas of different floral assemblages, based on their position and distance to the coastline, as well as water availability.
Facies associations in sediment profiles
Three profiles, ranging in thickness from 24 to 67 m, were measured from the Neurath Sand and overlying Garzweiler Seam in the Garzweiler open-cast mine and provide either a complete (profile 1) or partial (profiles 2 and 3) section through the sand body. The profiles can be subdivided, on the basis of the sedimentary facies present, into six facies associations (FA; Fig. 8), and are described below.

Fig. 8. The measured profile through the Neurath Sand, Garzweiler open-cast mine. The three sections 1, 2 and 3 are laterally positioned 200 m from one other along a N–S axis. Six facies associations (FA1–6) have been identified, composed of the various sedimentary facies (six in total), including lignite, medium- to coarse-grained sand, medium-grained sand, fine- to medium-grained sand, fine-grained sand and muddy sand.
Facies association 1
The lowermost facies (FA1) of the Neurath Sand is subdivided into three parts (FA1a, b, c) and comprises three facies (Sf-u, Sf-c, Sfm-c). The lowermost parts (FA1a, b) are only present in profile 1, which commences with 2.4 m (FA1a) of heavily bioturbated sands (Sf-u) containing Ophiomorpha ichnosp. The horizontal Ophiomorpha ichnosp. burrows are mainly oval in shape, presumably as a result of subsequent compaction. Following an 8 m interval of FA2 (see below), the second part (FA1b) commences. This comprises mainly bioturbated sands (Sf-u), but also includes cross-laminated sand (Sf-c) (Fig. 8). Within the former, the percentage of bioturbation increases markedly from base to top within the individual beds. Bed tops are sharp (erosion); bioturbation recommences in the overlying bed.
The uppermost part of the facies association (FA1c) can be traced across all three sedimentary profiles (Fig. 8). This part comprises cross-laminated sands (Sf-c) and fine- to medium-grained sands (Sfm-c), both of which also include tidal bundles, with a black sand/sand-clast layer also present in profiles 1 and 2.
Interpretation
As noted above, FA1 can be subdivided into three parts. The lowermost two parts (FA1a, b) are broadly similar (both comprising mainly Sf-u), while FA1c comprises mainly Sf-c and Sfm-c. FA1a, b would appear to have been deposited in a low-energy marine environment on the basis of grain size, and the presence of abundant Ophiomorpha ichnosp (Seilacher, Reference Seilacher1964; Hiscott et al., Reference Hiscott, James and Pemberton1984; Howard & Frey, Reference Howard and Frey1984; MacEachern et al., Reference MacEachern, Bann, Gingras, Zonneveld, Dashtgard, Pemberton, Knaust and Bromley2012).
The uppermost part (FA1c) comprises mainly fine- (occasionally medium-)grained sands with well-developed planar and trough cross-lamination (Sf-c, Sfm-c), as well as tidal bundles and black sand/sand–clast beds (Fig. 6e). The former suggest deposition in a higher-energy setting than FA1a, b, thus evidencing a gradual increase in environmental energy within the lower part of the Neurath Sand. Deposition was most probably within the upper shoreface part of a subtidal environment, in contrast to the lower shoreface setting for FA1a, b. It is also possible that the transition from FA1a, b to FA1c marks a time gap between the two phases of this facies association. However, if that were the case, then it might be expected that a marked erosional horizon would be present at the boundary between FA1b and FA1c in profile 1, or even possibly a lag deposit. In the marked absence of both, it would appear more feasible to conclude that the transition represents a phase of shallowing within the broader depositional setting. The presence of tidal bundles (occasionally including both flood and ebb couplets; Hovikoski et al., Reference Hovikoski, Räsänen, Gingras, Roddaz, Brusset, Hermoza, Pittman and Lertola2005; Schäfer, Reference Schäfer2010) in FA1c would confirm the subtidal setting. Trough cross-lamination may be indicative of wave activity; thus, FA1c was probably deposited within a ridge-and-runnel system below the low-tide line. Lignite and mud clasts are also present with the latter, most probably derived from the reworking of adjacent tidal flats (indicated by mud deposits bounding the Neurath Sands in the S; Schäfer & Utescher, Reference Schäfer and Utescher2014), while the large wood fragments (e.g. up to 30 cm long branches) were probably transported by fluvial and tidal currents from the coastal mires. Similarly, the presence of organic fragments within the black sand/sand–clast layers would suggest proximity to abundant organic sources.
Facies association 2
Facies association 2 (FA2), which is only present in profile 1, comprises just one facies (Smu) in five different beds. It commences with a chert-pebble lag where the matrix is light-coloured. This passes upsection into a darker-coloured sand, with no evidence of any grading. The darker sands contain clasts of wood and lignite (mud clasts are present at the base of one bed at c. 6 m) concentrated in the lowermost beds of FA2 (Fig. 8). The overlying beds contain ichnofossil assemblages with initially a concentration of Skolithos linearis in the middle part of FA2, with Planolites ichnosp., Teichichnus ichnosp. and Skolithos linearis concentrated near the top of FA2 at c. 9 m (Fig. 8).
Interpretation
The chert-pebble lag indicates an initial high-energy depositional environment which changed rapidly to a lower-energy one. Chert pebbles within the Ville Formation have been interpreted as marine deposits of the shoreface environment (Petzelberger, Reference Petzelberger1994), possibly reflecting a transgressive surface (Utescher et al., Reference Utescher, Ashraf, Dreist, Dybkjaer, Mosbrugger, Pross and Wilde2012). The cherts are possibly derived from the Aachen–Limburg carbonate plateau (Albers & Felder, Reference Albers and Felder1981). Karstification of the Cretaceous-aged carbonates in the Aachen–Maastricht–Liège area (W margin of the Lower Rhine Basin; or possibly from the London–Brabant High?) was followed by transport of chert pebbles, commencing with the Oligocene-age transgression (Albers & Felder, Reference Albers and Felder1981). During Oligocene and Miocene times, the chert pebbles were redistributed and deposited within marine sediments in the Lower Rhine Basin (Albers & Felder, Reference Albers and Felder1981; Petzelberger, Reference Petzelberger1994).
The overlying non-stratified muddy sands (Smu) most probably represent a period of increasing water depth (following FA1), and decreasing environmental energy, which would be indicative of a transition zone. This is confirmed by the presence of Skolithos linearis, Planolites ichnosp. and Teichichnus ichnosp., which are indicative of the low- to moderate-energy conditions below storm wave-base (MacEachern et al., Reference MacEachern, Bann, Gingras, Zonneveld, Dashtgard, Pemberton, Knaust and Bromley2012).
Facies association 3
Description
FA3 can be observed within all three profiles, although its maximum thickness is only seen in the northernmost profile (profile 3, 7.5 m at the base, and 6.4 m within the upper part of the profile, with up to 1.75 m of FA4 separating these two units); the thicknesses are reduced in profiles 1 (5.4 m) and 2 (5.2 m). The facies association consists of two lithofacies, comprising cross-laminated and cross-bedded, medium-grained sands (Sm-c), as well as cross-laminated/cross-bedded, coarse- to medium-grained sands (Sc-c). The sands in profiles 1 and 3 are mainly trough cross-laminated/bedded (Fig. 9a), while in profile 2 they are predominantly planar cross-laminated/bedded. In contrast to FA1 no tidal bundles were observed in FA3.
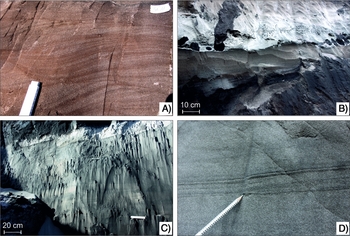
Fig. 9. Selected facies associations from the Neurath Sand. (A) Cross-laminated sands from FA3, profile 2; (B) planar-laminated, brown high-energy sands (FA4, swash zone), overlain by deposits of the breaker zone (FA3, light-grey sands). The overlying FA3 initiates with an erosionally bounded black sand-clast bed; (C) planar-laminated, fine- to medium-grained sands (comprising wood fragments) of the FA5 underlying the Garzweiler lignite seam (profile 1); (D) tidal rhythmite (sand-mud–sand-mud) in planar-laminated fine-grained sands of FA6 (profile 1).
Interpretation
The grain-size and sedimentary structures, as well as the absence of tidal bundles and ichnofossils, would suggest that the sand beds of FA3 were deposited in a lower intertidal (foreshore) environment. This setting is characterised by wave processes, as well as the regular exposure and covering during low and high tides, respectively (Le Hir et al., Reference Le Hir, Roberts, Cazaillet, Christie, Bassoullet and Bacher2000; Fan, Reference Fan, Davis and Dalrymple2012; Reynaud & Dalrymple, Reference Reynaud and Dalrymple2012).
Facies association 4
Description
Facies association 4 (FA4) comprises three facies, dominated by parallel-laminated, medium-grained sand beds (Sm-p), and rare fine- to medium-grained beds (Sfm-p), sometimes alternating, or fining/thinning upwards. In profile 1, the FA is topped by a 20 cm bed of cross-laminated sand with ripple lamination (Sm-c).
Interpretation
The medium-grained, planar-bedded sands indicate deposition within the upper foreshore (Clifton, Reference Clifton1981). These beds formed under a high-energy flow regime, when breaking waves cause swash and backwash flows on the beach surface (Aagaard & Hughes, Reference Aagaard and Hughes2006; Masselink & Puleo, Reference Masselink and Puleo2006). Alternations of fine- to medium-grained with medium-grained sand beds, as well as fining-up beds, suggest variable energy conditions within the upper foreshore, possibly related to variations in wave height (e.g. seasonal, varying tidal current vel-ocities). The thinning-upward pattern observed within the laminae of profile 3 may be related to changes in water depth and a possible shift of the depositional setting from the swash to the breaker zone at the top of the FA (Fig. 9b). The small-scale ripples (Sm-c) most probably were deposited at the high-tide level, and mark the transition from the high-energy foreshore setting to the overlying backshore environment (FA5; see below).
Facies association 5
Description
This FA (Fig. 9c), which can be traced across all three profiles, comprises three facies: fine-grained sand (which may be non-stratified, Sf-u, as well as planar-laminated, Sf-p), fine- to medium-grained, planar-laminated/bedded sand (Sfm-p) and thick (up to 10 m) lignite deposits (L). In addition, the FA5 overlies both FA4 and FA6 (Fig. 8). The sands are rich in both wood fragments and lignite clasts, with the concentrations increasing towards the lignite (i.e. near the top of the FA). The boundary between the lignites and sands is characterised by extensive root horizons (Fig. 6c), while the sands immediately underlying the lignites are generally black. The boundaries between sand deposits and the overlying lignite are commonly gradational.
Interpretation
The sediment grain sizes, sedimentary structures (predominance of parallel lamination as a result of aeolian deposition) and the presence of lignite would suggest that FA5 was deposited in a backshore environment with an adjacent coastal mire (Bartholdy, Reference Bartholdy, Davis and Dalrymple2012). The sand-rich lower part of the FA provides a link between the shallow-marine foreshore sediments and the coastal peat deposits, which formed subsequent to the marine regression (Bartholdy, Reference Bartholdy, Davis and Dalrymple2012). The black colour of the sands directly underlying the lignites resulted from infiltration of mobile dispersed organic matter (humic gels) derived from the lignites (see above).
Facies association 6
Description
This FA comprises sand-rich lenses which show an irregular lateral distribution within the Garzweiler Seam, and is present in only two of the measured profiles (Fig. 8). The sand-rich lenses of FA6 comprise three lithofacies, including planar-laminated, fine-grained sands (Sf-p), and fine- to medium-grained sands, which are either non-stratified (Sfm-u), or planar-laminated (Sfm-p), with a clear erosive boundary (characterised by a concentration of wood fragments and angular to rounded lignite clasts) present in profile 1. Tidal bundles may also be present (Fig. 9d). The upper part of the FA is characterised by the presence of root horizons (extending from the overlying lignite) and rare lignite concentrations.
Interpretation
The sand lenses most probably have a marine origin, based on the presence of tidal bundles (Dalrymple et al., Reference Dalrymple, Zaitlin and Boyd1992; Archer, Reference Archer1996; Stupples, Reference Stupples2002; Mazumder & Arima, Reference Mazumder and Arima2005; Kvale, Reference Kvale, Davis and Dalrymple2012), with deposition occurring in a moderate-energy setting. Although these deposits combine characteristics of the subtidal, intertidal and supratidal environments, it is probable that they represent deposition in a bight, lagoon or estuarine setting due to the limited lateral extent of the sand bodies.
Discussion
Depositional model
Based on our detailed facies analysis from three measured sedimentary profiles, the sediments of the Neurath Sand from the Garzweiler open-cast mine are interpreted as a transgressive–regressive succession. The sequence of events, which occurred in the shallow-marine North Sea in Serravallian times, resulted in the deposition of a series of units as outlined below.
The base of the succession is represented by the lignites of the Frimmersdorf Seam, originally laid down in a near-coastal swamp environment which was slowly transgressed by the North Sea. The contact between the Neurath Sand and the underlying Frimmersdorf Seam was not exposed during the profile survey of this study, although previous studies suggest it was erosional (Schäfer & Utescher, Reference Schäfer and Utescher2014). The lowermost preserved glauconite-bearing sediments (containing Ophiomorpha ichnosp.) were deposited in a shoreface environment (subtidal), i.e. within the area between low-tide level and the storm wave-base. Ophiomorpha ichnosp. are common in beach and shallow subtidal settings (Seilacher, Reference Seilacher1964; Hiscott et al., Reference Hiscott, James and Pemberton1984; Howard & Frey, Reference Howard and Frey1984; MacEachern et al., Reference MacEachern, Bann, Gingras, Zonneveld, Dashtgard, Pemberton, Knaust and Bromley2012) and can be compared to the modern shrimps Callianassa major (Frey et al., Reference Frey, Howard and Pryor1978). Ophiomorpha ichnosp. is frequently found in marine sediments of the Lower Rhine Basin (e.g. Ophiomorpha nodosa; Suhr, Reference Suhr1982, Reference Suhr1989; Petzelberger, Reference Petzelberger1994).
The Ophiomorpha ichnosp.-rich bed is overlain by an extensive chert-pebble bed which forms a significant marker horizon within the Garzweiler open-cast mine. Chert pebbles are widely distributed in the marine deposits of the Lower Rhine Basin (Petzelberger, Reference Petzelberger1994) and have been traced back to the Aachen–Limburg carbonate plateau (Albers & Felder, Reference Albers and Felder1981). Following intense karstification of Cretaceous-aged carbonates, the residual cherts were redistributed throughout the Lower Rhine Basin by the repeated transgressions which occurred across the region (Albers & Felder, Reference Albers and Felder1981). In the Neurath Sand, it has been suggested that the chert-pebble bed represents a transgressive surface (Utescher et al., Reference Utescher, Ashraf, Dreist, Dybkjaer, Mosbrugger, Pross and Wilde2012).
The overlying muddy sands (FA2) suggest a marked decrease in ambient energy levels within the depositional setting. This, together with a possible increase in water depth as suggested by the ichno-assemblage (Skolithos linearis, Planolites ichnosp. Teichichnus ichnosp.), marks a change to a transitional environment, namely a nearshore setting linking the beach and shelf environments (MacEachern et al., Reference MacEachern, Bann, Gingras, Zonneveld, Dashtgard, Pemberton, Knaust and Bromley2012). These transitional deposits represent the maximum flooding stage of the Lower Rhine Basin in Serravallian times, and their deposition (heralded by the deposition of the chert-pebble unit) was followed by the slow onset of regressive conditions (FA1 overlying FA2). The regressive period is represented by sands deposited within a subtidal environment. These contain Ophiomorpha ichnosp. in their lower parts, and are covered by (trough) cross-laminated sand units formed as dunes in a ridge-and-runnel system. The presence of sand–mud couplets has been interpreted as unequivocal evidence of tidal activity. These commonly occur as two paired couplets (i.e. sand-mud–sand-mud) and their presence would suggest that both ebb and flood currents were strong enough for sand transport to occur (Hori et al., Reference Hori, Saito, Zhao, Cheng, Wang, Sato and Li2001). These tidal bundles are indicative of subtidal conditions, as well as deposition within channels or estuaries (Dalrymple et al., Reference Dalrymple, Zaitlin and Boyd1992; Archer, Reference Archer1996; Stupples, Reference Stupples2002; Mazumder & Arima, Reference Mazumder and Arima2005; Kvale, Reference Kvale, Davis and Dalrymple2012).
The regressive trend continued with the deposition of a series of sand beds within the foreshore environment (FA3, FA4). This intertidal succession commenced with the deposition of the sediments of the breaker zone, a high-energy setting dominated by wave and tidal processes. The ridge-and-runnel system within this sedimentary environment results from wave activity, tidal processes and longshore currents (Dabrio, Reference Dabrio1982; Kroon & Masselink, Reference Kroon and Masselink2002). These ridges comprise large to very large sand dunes (Reynaud & Dalrymple, Reference Reynaud and Dalrymple2012) covered by smaller dunes and resulting in the formation of a complex pattern of compound cross-bedding (also observed in the Neurath Sand deposits). The overlying planar-laminated/bedded, medium-grained sands are also interpreted as intertidal deposits, although these high-energy laminites were probably deposited in the swash zone of the upper foreshore, as a result of the uprush and backwash of strong and unsteady wave flows on the beachface (Masselink & Puleo, Reference Masselink and Puleo2006).
The overlying supratidal sands (FA5) were deposited on the beach surface above high-tide level. Small ripple structures between the supratidal and the underlying intertidal deposits indicate the high-tide line, or beach berm, thus marking the position of maximum tidal flooding (Schäfer, Reference Schäfer2010). There is no evidence of aeolian dune deposition within the Neurath Sand. Boersma (Reference Boersma1991) suggested that low or non-existent dunes within the Lower Rhine Basin were the result of wet substrates at the backshore, associated with high water-levels in a back barrier lagoon. The backshore sands were, in turn, overlain by lignite, reflecting the accumulation of paralic peats in nearshore marshes and mires. The peat accumulations within the coastal mire can be up to 30 m thick (10 m thick present-day Garzweiler Seam) as a result of continuous subsidence of the basin. Roots from the plants growing in these paralic environments penetrated into the underlying sands (Fig. 6c) of the backshore environment. The degree of root penetration would suggest that the sands were flushed of marine interstitial pore waters and replaced with fresh water from rain and rivers. This flushing presumably occurred prior to the development of a continental flora (with the possible exception of saline-tolerant plants which represent the early pioneer plants on the recently drained surface; however, the remaining roots probably represent subsequent vegetation, based on the diameter and length of the roots; Darscheid, Reference Darscheid1981). Various floral assemblages growing within the Miocene-aged mires have been analysed, depending on the distance to the sea as well as water availability (M. Teichmüller, Reference Teichmüller1958; R. Teichmüller, Reference Teichmüller1958).
Based on anatomical studies of wood and palynological data, the typical peat-forming vegetation of the coastal mires consisted of diverse open mixed forests and scrubs comprising conifers and angiosperms (van der Burgh, Reference Van der Burgh1973; von der Brelie & Wolf, Reference Von der Brelie and Wolf1981; Mosbrugger et al., Reference Mosbrugger, Gee, Belz and Ashraf1994; Figueiral et al., Reference Figueiral, Mosbrugger, Rowe, Ashraf, Utescher and Jones1999). Analyses carried out on stump horizons indicate that, at times, relatively dense conifer forests existed. These were mainly composed of Taxodiaceae and Pinaceae (with Sciadopitys as the most common genus), and subordinate palms (Mosbrugger et al., Reference Mosbrugger, Gee, Belz and Ashraf1994). A detailed palaeovegetation analysis (based on palynology) carried out on the Garzweiler Seam in the Hambach open-cast mine provided the basis for a reconstruction of the vegetational succession of the seam (Huhn et al., Reference Huhn, Utescher, Ashraf and Mosbrugger1997). A pine–ericalean pocosin-type vegetation, representing well-drained soil conditions, developed at the base of the seam, and was succeeded by scrub vegetation dominated by plant species of the Myrica, Betula and Cyrilla genera. In the upper part of the seam, a gradual transition to a Taxodium–Nyssa association was recorded, suggesting a change to a very high water table. Pollen analysis would suggest that angiosperm herbs did not play a significant role in the Garzweiler vegetation (Huhn et al., Reference Huhn, Utescher, Ashraf and Mosbrugger1997). Organic petrological and organic geochemical analyses, carried out on samples from the Morken, Frimmersdorf and Garzweiler seams in the Garzweiler open-cast mine, suggested that the peat-forming vegetation of the coastal mires probably consisted of a combination of herbaceous-vegetated areas (Lücke et al., Reference Lücke, Helle, Schleser, Figueiral, Mosbrugger, Jones and Rowe1999; Stock et al., Reference Stock, Littke, Zieger and Thielemann2016).
The two lens-shaped sand intercalations within the Garzweiler Seam (FA5), separated by a thin lignite band, have been interpreted as a result of a regionally restricted inundation of the swamp environment at its northern boundary. The sand beds contain tidal bundles, which are typical for the shoreface environment below the low-tide level. Planar lamination, on the other hand, may indicate an intertidal depositional environment (i.e. high-energy laminites; see above). The fine-grained nature of these deposits, however, would suggest a low- to moderate-energy setting. As these sand deposits are of limited regional extent (tens of metres; Fig. 10) they are interpreted as representing a lagoonal or estuarine setting. To the north, the lens-shaped sand intercalations can be correlated with a cross-laminated, subaqueous (intertidal to subtidal) dune system (cross-lamination and tidal bundles in the upper part of profile 3). These represent either coast-parallel barrier sand bars (associated with barrier islands; i.e. wave breakers that protect the lagoonal milieu from the waves of the open sea; Fan, Reference Fan, Davis and Dalrymple2012) or, possibly, tidal sand bars (stream-parallel), which are characteristic of estuarine mouth areas (Dalrymple et al., Reference Dalrymple, Mackay, Ichaso, Choi, Davis and Dalrymple2012).

Fig. 10. Cross section of the Ville Formation from the Garzweiler open-cast mine (W of the measured profiles), interpreted from exploration drillings and geophysical well logs (RWE Power AG). The cross section comprises the northernmost extent of the Garzweiler Seam, as well as the laterally restricted sand bodies.
Channel deposits
Four characteristic beds containing black sand clasts were observed within the three measured profiles, and have been interpreted as channel deposits. The black sand clast-rich beds are interbedded with (trough) cross-laminated sands, which were deposited within a ridge-and-runnel system (e.g. Dabrio, Reference Dabrio1982; Masselink & Anthony, Reference Masselink and Anthony2001). These beach-parallel sand ridges (i.e. longshore bars or dunes; Boersma, Reference Boersma1991; Fan, Reference Fan, Davis and Dalrymple2012; Reynaud & Dalrymple, Reference Reynaud and Dalrymple2012) may be cut by rip channels (e.g. Aagaard et al., Reference Aagaard, Greenwood and Nielsen1997; Haller, Reference Haller2002; Castelle & Coco, Reference Castelle and Coco2012) or tidal channels (e.g. Weimer et al., Reference Weimer, Howard, Lindsay, Scholle and Spearing1982; Lanzoni, Reference Lanzoni2002; Dalrymple & Choi, Reference Dalrymple and Choi2007; Hughes, Reference Hughes, Davis and Dalrymple2012). Both rip as well as tidal channels are considered to be important pathways for water–sediment exchange between the coastal and shelf environments (Aagaard et al., Reference Aagaard, Greenwood and Nielsen1997; Fan, Reference Fan, Davis and Dalrymple2012; Hughes, Reference Hughes, Davis and Dalrymple2012).
The erosionally bounded black sand beds and the associated intraformational (i.e. rip-up) clasts were deposited in tidal channels (Collinson et al., Reference Collinson, Mountney and Thomson2006). According to Hughes (Reference Hughes, Davis and Dalrymple2012), sand-dominated deposits in tidal channels represent the higher-energy periods of spring tides. Studies of tidal channels (mostly within intertidal environments; e.g. Lanzoni, Reference Lanzoni2002; Hughes, Reference Hughes, Davis and Dalrymple2012), commonly note the presence of a coarse-sediment lag, including shells, wood fragments, mud clasts or pebbles, along the erosional bounding surface (Clifton, Reference Clifton1982; Hughes, Reference Hughes, Davis and Dalrymple2012). These lag deposits may also include mud block breccias, which formed as a result of bank slumping or channel edge erosion (Terwindt, Reference Terwindt1988; Hughes, Reference Hughes, Davis and Dalrymple2012). Neither shells nor mud blocks are found in association with the intraformational sands in the Neurath Sand, the former having been dissolved by the passage of humic acids through the sand body, and the latter most probably did not form due to the lack of adjacent mud banks.
Tidal channels are commonly characterised by features produced as a result of tidal activity (e.g. reactivation surfaces, mud drapes in cross-sets, low-angle dipping cross-sets with alternating thicker and thinner packages of heterolithic sediments, flaser- and/or lenticular bedding or tidal bundles; de Raaf & Boersma, Reference De Raaf and Boersma1971; Hughes, Reference Hughes, Davis and Dalrymple2012). None of these, however, has been observed in the Neurath Sand channel deposits. The absence of characteristic features indicative of changing flow directions (ebb and flood currents) suggests deposition under unidirectional flow conditions (i.e. either ebb or flood). Additionally, the presence of wood remains, as well as the black humic substances, forming coats around each individual grain within the channel deposits (see above), suggests an ebb-dominated environment, with strong ebb currents transporting the humic substances as well as wood remains from the coastal mires into the intertidal and subtidal environments. However, tidal bundles within the surrounding subtidal deposits would suggest that both ebb and flood currents were generally strong enough for sand transport. Tide-generated channels can also be interpreted in terms of estuarine systems (Dalrymple & Choi, Reference Dalrymple and Choi2007; and see below). These tide-generated swatchways separate elongate tidal sand bars at the transition from the estuary mouth to the marine setting (Dalrymple et al., Reference Dalrymple, Mackay, Ichaso, Choi, Davis and Dalrymple2012).
Another possible explanation for the formation of channels within this depositional environment may be related to rip currents. These offshore-directed currents are the result of seaward return flows through narrow channels between sand bars, and are induced by the backwash of waves (Aagaard et al., Reference Aagaard, Greenwood and Nielsen1997). The infill of channels may occur as a result of channel migration or decreasing wave energy (Aagaard et al., Reference Aagaard, Greenwood and Nielsen1997). However, rip currents are restricted to the intertidal environment (Masselink & Hegge, Reference Masselink and Hegge1995; Aagaard et al., Reference Aagaard, Greenwood and Nielsen1997; Castelle & Coco, Reference Castelle and Coco2012) and can thus only be regarded as responsible for the formation of the sand-clast beds within the breaker zone.
The unidirectional flow conditions within the black sand-clast beds, the presence of humic substances, chert pebbles and wood fragments may all also be interpreted in terms of a single high-energy flow event. According to Hunt et al. (Reference Hunt, Bryan and Mullarney2015), erosion of sediments within the intertidal environment only occurs when tidal currents are reinforced by increased wave or wind energy. Studies of recent rip channels have noted that their initial formation can occur as a result of storms (Aagaard et al., Reference Aagaard, Greenwood and Nielsen1997). Additionally, channel migration and channel infill have been observed after storm events (e.g. Castelle & Coco, Reference Castelle and Coco2012). During such events, increased wave energy can lead to extensive erosion of the coastal area (e.g. Russell, Reference Russell1993; Eisma, Reference Eisma1998), followed by the distribution of backshore deposits into the marine setting through tidal channels and rip currents. However, the absence of hummocky cross-stratification (generally characteristic of storm-induced sedimentation, and completely absent throughout the Lower Rhine Basin) might suggest an absence of storm activity. On the other hand, the presence of Groden bedding (which forms as a result of storm surges inundating coastal marshes; Boersma, Reference Boersma1991; Schäfer et al., Reference Schäfer, Utescher and Mörs2004; Bungenstock & Schäfer, Reference Bungenstock and Schäfer2009; Schäfer, Reference Schäfer2010) in the underlying Frimmersdorf Sand would suggest that storm deposition did indeed occur within the Lower Rhine Basin. Additionally, hummocky cross-stratification may be deformed or destroyed due to tides or storm wave activity (Eyles & Clark, Reference Eyles and Clark1986).
As noted above, flow velocities within rip currents generated by the backwash of waves can be increased/reinforced by tidal currents, in particular by seaward-directed ebb currents (Aagaard et al., Reference Aagaard, Greenwood and Nielsen1997). The deposits of the Neurath Sand show both the distinct influence of wave activity and the impact of tides. Therefore, both waves and tides (i.e. rip currents and tidal or estuarine currents) may have been responsible for the formation of the observed channel deposits.
Depositional environment: tidal delta vs estuary
The broader depositional setting of the Neurath Sand is problematic. In general, the Lower Rhine Basin in Miocene times formed an embayment, bounded by the uplifting Rhenish Massif in the SE and SW, and extending into the North Sea Basin in the NW (e.g. Schäfer et al., Reference Schäfer, Hilger, Gross and von der Hocht1996; Lücke et al., Reference Lücke, Helle, Schleser, Figueiral, Mosbrugger, Jones and Rowe1999; Klett et al., Reference Klett, Eichhorst and Schäfer2002).
Two sources of clastic sediment input have been identified from the Lower Rhine Basin (Hager, Reference Hager1993). The first, providing fine-grained quartz sands, was located in the SW North Sea, and the sediments were transported by coastal longshore currents (Breddin, Reference Breddin1932; Hager, Reference Hager1993) following their deposition in the Lower Rhine Basin. The presence of large sand dunes and tidal bundles within the Neurath Sand provides unequivocal evidence for tidal and wave activity within the shallow-marine environment. The tidal range within the Miocene-age North Sea has been estimated at 2–4 m (mesotidal; Schäfer & Utescher, Reference Schäfer and Utescher2014), although both tidal range and wave height can increase as a result of funnelling within embayments (e.g. estuaries or tidal inlets; Mazumder & Arima, Reference Mazumder and Arima2005; Dalrymple & Choi, Reference Dalrymple and Choi2007; Longhitano et al., Reference Longhitano, Mellere, Steel and Ainsworth2012).
The second sediment source was located to the SE (i.e. Rhenish Massif), with sediment transported by medium- to low-energy fluvial systems entering the Lower Rhine Basin (Hager, Reference Hager1993). It is probable that the Rhenish Massif in Miocene times was dewatered by several small river streams (compared to the present-day fluvial system within the Lower Rhine Basin). Peat accumulation of the Main Seam (i.e. the combined Morken, Frimmersdorf and Garzweiler Seams in the SE) took place on the banks of one of these systems, extending through the centre of the basin (Zagwijn & Hager, Reference Zagwijn and Hager1987). This river system, interpreted as a precursor of the present-day river Sieg (Hager, Reference Hager1993), subsequently shifted its location to the SW (Zagwijn & Hager, Reference Zagwijn and Hager1987).
It has been suggested that a deltaic depositional environment formed at the entry point of the various fluvial systems (Boersma, Reference Boersma1991; Hager, Reference Hager1993), based on the presence of a number of small to medium river systems, as well as the Rhenish Massif representing the provenance area. However, as described within this study, features that would represent characteristic deltaic deposits are not present within the Neurath Sand and, thus, such a model does not conform to the observations. Schäfer et al. (Reference Schäfer, Hilger, Gross and von der Hocht1996) have suggested that the sediments of the Neurath Sand were deposited in an estuarine system. Such a system develops in a transgressive setting (e.g. Dalrymple et al., Reference Dalrymple, Zaitlin and Boyd1992; Dalrymple, Reference Dalrymple2006; Dalrymple & Choi, Reference Dalrymple and Choi2007), followed by the infill of the estuarine channels during progradation of the shoreline (Dalrymple et al., Reference Dalrymple, Mackay, Ichaso, Choi, Davis and Dalrymple2012). As noted above, the Serravallian-age North Sea transgressed onto the peat accumulation of the present-day Frimmersdorf Seam, and the lowermost part of the Neurath Sand (i.e. FA1a, FA2) was subsequently deposited in a deepening marine setting (i.e. transgressive). Subsequent regression of the North Sea (FA1b, c – FA5) resulted in the deposition of a progradational shoreline sequence. Studies on recent and ancient estuarine systems have shown that infilling estuaries form features which are transitional with deltas (Dalrymple et al., Reference Dalrymple, Mackay, Ichaso, Choi, Davis and Dalrymple2012). Within the Lower Rhine Basin, the deposits of the Garzweiler Seam, and particularly of the overlying Inden Formation, mark the transition from a probable estuarine system (Neurath Sand) to a fluvial to deltaic system formed by the early Rhine river, which initially entered the Lower Rhine Basin in Tortonian times (Boenik, Reference Boenik2002; Sissingh, Reference Sissingh2006; Kemna, Reference Kemna2008; Westerhoff et al., Reference Westerhoff, Kemna and Boenik2008). As such, the Neurath Sand could possibly be interpreted in terms of the estuarine model.
This model is confirmed by the presence of channel deposits (i.e. the black sand clasts), tidal bundles and sand dunes. The channel deposits, represented by the black sand-clast beds, probably represent swatchways, separating elongate tidal sand bars (Dalrymple et al., Reference Dalrymple, Mackay, Ichaso, Choi, Davis and Dalrymple2012). The cross-laminated/-bedded dune deposits of the sub- to intertidal settings of the Neurath Sand are interpreted as subtidal and intertidal sand bars, which are ubi-quitous in the funnel-shaped mouth areas of estuaries (Dalrymple et al., Reference Dalrymple, Zaitlin and Boyd1992, Reference Dalrymple, Mackay, Ichaso, Choi, Davis and Dalrymple2012). The orientation of the cross-laminated dunes, however, could not be measured (due to the outcrop situation), so it is not possible to distinguish between coast-parallel orientated dunes (ridge-and-runnel systems, Dabrio Reference Dabrio1982; Masselink & Anthony Reference Masselink and Anthony2001) and tidal sand bars (estuary, Dalrymple et al., Reference Dalrymple, Zaitlin and Boyd1992), which are orientated mainly stream-parallel (and, therefore, broadly perpendicular to the coastline; Dalrymple et al., Reference Dalrymple, Mackay, Ichaso, Choi, Davis and Dalrymple2012). The lens-shaped sand intercalations within the Garzweiler Seam, as noted above, provide evidence of tide-dominated sand deposition within restricted areas of the mire, which may also be indicative of an estuarine origin (Dalrymple et al., Reference Dalrymple, Zaitlin and Boyd1992, Reference Dalrymple, Mackay, Ichaso, Choi, Davis and Dalrymple2012). In contrast to modern and ancient estuaries (e.g. Bostock et al., Reference Bostock, Brooke, Ryan, Hancock, Pietsch, Packett and Harle2007; Dalrymple & Choi, Reference Dalrymple and Choi2007; Choi, Reference Choi2010; Uncles, Reference Uncles2010; Dalrymple et al., Reference Dalrymple, Mackay, Ichaso, Choi, Davis and Dalrymple2012), however, there was no major fluvial system entering the Lower Rhine Basin in Miocene times, but several small river systems, dewatering the adjacent Rhenish Massif and the adjacent coastal mires (Zagwijn & Hager, Reference Zagwijn and Hager1987; Hager, Reference Hager1993).
Conclusions
The Serravallian-age Neurath Sand is interpreted in terms of a characteristic shoreline succession within a tide- and wave-dominated embayment. The transgression of the North Sea resulted in the deposition of subtidal deposits covering the peat accumulations of the present-day Frimmersdorf Seam, followed by mud-rich sediments of the transition zone as a result of increasing water depth. Subsequently, regressive conditions resulted in the deposition of a sequence of subtidal, intertidal and supratidal sediments and finally the re-establishment of coastal mires (present-day Garzweiler Seam). Lens-shaped sand intercalations within the Garzweiler Seam, which comprise tidal bundles and are bounded by subaquatic sand dunes in the N, have probably been deposited in an estuarine or lagoonal milieu.
The interaction of wave and tidal currents with river systems, entering the Lower Rhine Basin from the adjacent Rhenish Massif, and also dewatering the peat accumulations of the present-day Main Seam in the S, are indicative of an estuarine system. The estuarine system was formed by several comparably small rivers, flanked by coastal mires and marshes in the SE, which laterally passed into tidal mudflats, and, with increasing water depth to the NW, foreshore and shoreface environments in the centre of the Lower Rhine Basin.
Acknowledgements
We acknowledge the funding for this study from RWE Power AG. Thanks to the staff members of the department GOC-L, especially the field crew of the Garzweiler open-cast mine, as well as Ulrich Krüger and Horst Hassel for editing datasets. The manuscript benefited greatly from the comments of Wim Westerhoff, Marinus Eric Donselaar and an anonymous reviewer.