Introduction
The metallogenic processes intimately associated with a variety of late- to post-magmatic events are sometimes poorly understood in detail due to a wide compositional diversity of the fluids involved in metal transport and deposition, where the late silicate phases may be an indicator of a distinct stage of mineralization. One of the most common late silicate phases is chlorite. It is frequently found either in hydrothermal alteration haloes that host ores or accompanying the ore-forming phases. The chlorite represents an alteration product following the breakdown of different primary minerals as the H+ or Mg2+,Fe2+-metasomatism proceeds. These reactions are usually related to propylitic alteration (i.e. actinolite + epidote + chlorite or epidote + chlorite + calcite or chlorite + calcite + hematite assemblages). The reactions are quite common and well developed in a wide range of ore-forming systems, including iron-oxide copper gold, porphyry Cu, Cu-Mo or Cu-Au, epithermal Au-Ag, and retrograde alteration stages of skarn-type deposits (e.g. Lowell and Guilbert, Reference Lowell and Guilbert1970; Gustafson and Hunt, Reference Gustafson and Hunt1975; Meinert, Reference Meinert1992; Sillitoe, Reference Sillitoe2000, Reference Sillitoe2010; Cooke et al., Reference Cooke, Hollings, Wilkinson, Tosdal, Holland and Turekian2014). The chemical composition of chlorites from the distal fringes of hydrothermal envelopes is distinct from that characteristic of metamorphism.
The wide range of crystal chemistry and structure displayed by the chlorite minerals group may also be used as a tool to determine the direction towards the mineralized area, to estimate the temperature under which the ore process took place (e.g. Cathelineau and Nieva, Reference Cathelineau and Nieva1985; Walshe, Reference Walshe1986; Inoue et al., Reference Inoue, Kurokawa and Hatta2010) or to estimate the heat flux from a magmatic-hydrothermal centre (Sillitoe, Reference Sillitoe2010). Recently, the relationships ‘chlorite vs. metal assemblages’ were revisited and explored to use chlorite as a proxy for detecting porphyry ore deposits (Wilkinson et al., Reference Wilkinson, Zhaoshan Chang, Cooke, Baker, Wilkinson, Inglis, Chen and Gemmell2015).
Dispersion or remobilization of metals in long-lived magmatic hydrothermal systems occurs via circulation of compositionally diverse fluids under a relatively large range of temperatures, generating useful environmental markers when ore-forming minerals are deposited with other phases such as chlorite. When precipitated directly from the hydrothermal fluid, chlorite displays a chemical composition that reflects many of the constraints imposed by the fluid chemistry during mineralization. For example, a genetic linkage between Mg,Fe-chlorite or Fe,Mg-chlorite and Cu-Au mineralization (Dora and Randive, Reference Dora and Randive2015) or a Pb-Zn, Bi, Ag vein-type ore deposit (Inoue et al., Reference Inoue, Kurokawa and Hatta2010) has been established. Beside the relative enrichment of Fe or Mg in the octahedral sheet in chlorite, the systematic presence of measurable amounts of Mn has been tested as a new pathfinder for chlorite developed during an ore-forming processes. For instance, Fe,Mg,Mn-chlorite has been identified in Pb-Zn, Bi, Ag ore veins from the Toyoha geothermal system, Japan (Inoue et al., Reference Inoue, Kurokawa and Hatta2010), and Fe,Mn,Mg-chlorite was recognized in the Zn-Pb, Ag vein-type ore epithermal system in NE Chile (Chinchilla et al., Reference Chinchilla, Arroyo, Merinero, Piña, Nieto, Ortega and Lunar2016).
The main goal of the present contribution is to characterize the optical, structural and crystal chemistry of chlorite minerals associated with tungstate and sulfide mineralization from the W, (Cu,Mo) ore deposit of Borralha (Portugal). The relationships between the development of chlorite minerals and different ore-forming stages as well as discrimination of different mineralization events based on stable isotopic studies of chlorite minerals are addressed.
Geology
The Variscan orogeny is an obduction-collision orogenic event (e.g. Ribeiro et al. Reference Ribeiro, Pereira, Dias, Dallmeyer and Martinez1990, Reference Ribeiro, Munhá, Dias, Mateus, Peirera, Ribeiro, Fonseca, Araújo, Oliveira and Romão2007; Matte, Reference Matte1991), where three main deformation phases (D1, D2 and D3) have been documented in northern Portugal (Ribeiro, Reference Ribeiro1974; Noronha et al., Reference Noronha, Ramos, Rebelo, Ribeiro and Ribeiro1981). The maximum crustal thickening was achieved by the end of D1/D2 phases, whereas the D3 phase (intra-Westphalian age) relates to the final stages of the continental collision process. Most of the granite intrusions and the associated thermal metamorphic peak are coeval with the D3 phase (e.g. Ferreira et al., Reference Ferreira, Iglesias, Noronha, Pereira, Ribeiro, Ribeiro, Bea, Carnicero, Gonzalo, Lopez Plaza and Rodriguez Alonso1987; Dias and Ribeiro, Reference Dias and Ribeiro1995; Noronha et al., Reference Noronha, Cathelineau, Boiron, Banks, Dória, Ribeiro, Nogueira and Guedes2000; Ribeiro et al., Reference Ribeiro, Munhá, Dias, Mateus, Peirera, Ribeiro, Fonseca, Araújo, Oliveira and Romão2007). The syn- to late-orogenic magmatic activity generated voluminous granitoid batholiths, often with contrasting compositions, and the emplacement was, in most cases, controlled by major Variscan structures.
The W, (Cu,Mo) deposit of Borralha is located in the northwestern Iberian Peninsula near the boundary between the Central Iberian Zone and the Galicia Trás-os-Montes subzone (Fig. 1), where different synorogenic Variscan granites intrude Palaeozoic metasedimentary rocks to form two-mica peraluminous (syn-D3) granites and biotite-rich (syn-D3) granites. The ore deposit occurs at the contact between metasedimentary formations (Silurian?) and the syn-D3 porphyritic Borralha biotite granite (~315 Ma) and a syn-D3 two-mica granite (Fig. 2) assumed to be related to a concealed post-D3 granite body (i.e. the Gerês granite).
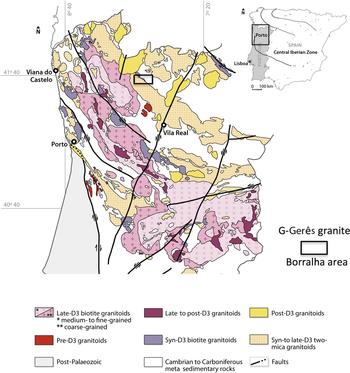
Fig. 1. Geological map of the northern part of Portugal showing the location of the W, (Cu,Mo) ore deposit of Borralha, Gerês Mountains (after Ferreira et al., Reference Ferreira, Iglesias, Noronha, Pereira, Ribeiro, Ribeiro, Bea, Carnicero, Gonzalo, Lopez Plaza and Rodriguez Alonso1987).
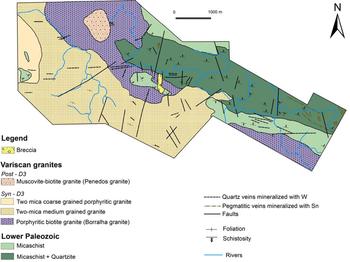
Fig. 2. Geological map of the Borralha region (after Noronha, Reference Noronha1983).
Petrogenetic and petrophysical studies of the post-D3 Gerês granite show that the magma rose relatively high in the crust and settled at upper crustal levels (6–7 km), generating a temperature gradient which triggered contact metamorphism at 200 MPa and 500–600°C which was sufficient to promote a vigorous hydrothermal convection cell (Noronha and Ribeiro, Reference Noronha and Ribeiro1983; Noronha et al., Reference Noronha, Cathelineau, Boiron, Banks, Dória, Ribeiro, Nogueira and Guedes2000).
The Borralha ore deposit is an important tungsten deposit of Portugal second only to Panasqueira. It was mined from 1903 to 1985 for wolframite, scheelite and argentiferous chalcopyrite. The mineralization occurs in quartz veins and in the two breccia pipes known as Santa Helena and Venise. The Santa Helena breccia occurs in outcrop (Fig. 2), whereas the Venise breccia was identified underground during mining. Rock fragments of variable size, cemented with mineralized coarse-grained quartz aggregates, characterize both breccias. Shallow levels of the Santa Helena breccia were mined for tungsten (i.e. wolframite). The ore mineral assemblage consists of tungstate (wolframite and scheelite), various sulfide minerals (chalcopyrite + molybdenite and pyrite, pyrrhotite, sphalerite, galena, bismuthinite, marcasite), native bismuth and Pb-Bi-Ag sulfosalts. Fluid-inclusion studies of quartz related to the W-mineralization identified aqueous-carbonic fluids (300°C < T < 400°C and 50 MPa < P < 100 MPa) and aqueous fluids (250°C < T < 300°C and 30 MPa < P < 50 MPa) in sulfide mineralization (Noronha, Reference Noronha1984a; Noronha et al., Reference Noronha, Vindel, López, Dória, García, Boiron and Cathelineau1999).
Other lode-quartz systems carrying W-Mo, (Sn) mineralization were described at Carris and Las Sombras (20 km NNW of Borralha), and are assumed to be related to the post-D3 Gerês granite (Cheilletz and Giuliani, Reference Cheilletz and Giuliani1982; Noronha, Reference Noronha1983, Reference Noronha1984a). An integrated metallogenic model was proposed by Noronha (Reference Noronha1984a) with four progressive stages of magmatic-hydrothermal evolution of the post-D3 Gerês granite: (1) Mo, (W) at Bouzadrago (Galicia, Spain); (2) W-Sn, (Cu,Mo,Bi) at Las Sombras (Galicia, Spain), Carris and Borrageiro; (3) W, (Cu,Mo,Bi,Sn) at Penedos; and (4) W, (Cu,Mo,Bi) at Borralha.
K-Ar dating of muscovite associated with molybdenite from the Venise breccia provided an age of 280 ± 5 Ma (Portugal-Ferreira and Noronha, unpublished data). K-Ar dating of feldspar from episyenitic rocks of the Gerês granite (Carris facies) yielded an age of 273 ± 11 Ma (Jaques et al., Reference Jaques, Noronha, Liewig and Bobos2016), whereas Re-Os dating of molybdenites from Carris quartz veins placed the ore-forming stage at 279.5 ± 1.7 (Moura et al., Reference Moura, Dória, Neiva, Leal Gomes and Creaser2014).
Samples and analytical techniques
Samples
Samples containing tungstates and Cu-,Zn-,Bi-sulfides were collected from three drill cores (at −40 m; −70 m; −176 m levels) of the Santa Helena breccia where the chlorite vs. mineralization occurs unambiguously at different scales. Representative samples of chlorite associated with Mo-mineralization were collected from the Venise breccia at the −110 m level. Illustrative features of these samples are: (1) a Fe,Mg-chlorite with quartz, albite and adularia assemblage (Fig. 3a); (2) Fe,Mn-chlorite with scheelite (Fig. 3b); (3) Fe-chlorite with W-mineralization (Fig. 3c); (4) Fe-chlorite with chalcopyrite + sphalerite ± pyrite and secondary muscovite (Fig. 3d); and (5) Fe,Mg-chlorite with molybdenite (adularia + quartz) (Fig. 3e). Fractions of Fe,Mg-chlorite (Fig. 3a) with quartz, albite and adularia (compositionally identical to Fe,Mg-chlorite with molybdenite), Fe-chlorite (Fig. 3d) and Fe,Mn-chlorite (Fig. 3b) were separated and used further in X-ray diffraction (XRD), optical microscopy, electron microprobe analyses (EMPA) and oxygen isotopic measurements. The samples involving chlorite + tungstates and chlorite + sulfides were studied by optical microscopy and EMPA and special attention was given to the chlorite minerals with W-Cu (Mo) mineralization. Chlorite minerals were identified in each mineralization type for complete structural, chemical and stable isotopic characterization using the same samples for each of these analyses.
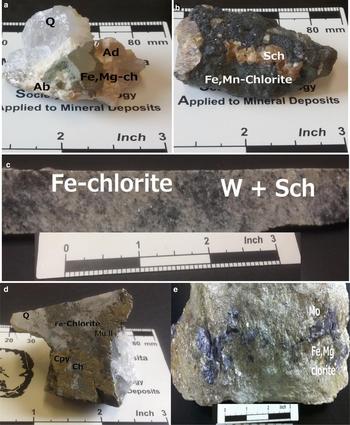
Fig. 3. Selected samples of chlorite minerals studied: (a) sample from Santa Helena collected at –48 m: Q – quartz; Ad – adularia; Ab – albite; Fe,Mg-chlorite; (b) sample from Santa Helena collected from veins at –148 m: Sch – scheelite; FeMn-chlorite; (c) sample collected from the Santa Helena breccia structure (North Sto. António at –160 m): W – wolframite; Sch – scheelite; Fe-chlorite; (d) sample from the Santa Helena breccia structure collected at –176 m: cpy – chalcopyrite; chl-Fe-chlorite, Mu II – secondary muscovite; (e) sample from Venise collected at –110 m; Mo – molybdenite; Fe,Mg-chlorite.
Analytical techniques
Optical microscopy
Thin polished sections were prepared for optical microscopy in transmitted and reflected light. Textural relationships between chlorite minerals and opaque minerals (i.e. tungstates and sulfides) were examined using a Nikon E400 polarized transmitted-reflected light microscope using thin polished sections (30 µm).
X-ray diffraction
Chlorite fractions (<2 µm) were separated from bulk-rock mineralized pulps by centrifugation. The oriented specimens of <2 µm fractions prepared on glass slides were analyzed in an air-dried (AD) condition and saturated in ethylene-glycol (EG) vapour for 24 h. Structural characterization of the <2 µm oriented clay-fraction specimens was performed using a Philips X'Pert diffractometer with CuKα radiation and a monochromator, working at 50 kV and 30 mA. A scanning speed of 0.05°2θ/min for the range 2–65°2θ and a counting time of 5 s/step were used. The interlayer nature, the polytype and d 060 values of the chlorite minerals were examined using randomly oriented specimens. The XRD patterns of these specimens were acquired using a RINTUltima+ diffractometer with a Cu X-ray tube, Ni-filter, and silicon strip X-ray detector (Rigaku D/teX Ultra). A measurement condition of 0.25° divergence slit, 10 mm mask confining the beam width, and 8 mm anti-scatter slit was used. The XRD pattern was obtained with a scanning speed of 0.10°2θ/min at a counting time of 20 s/step over the range 2–75°2θ.
EMPA
Quantitative compositions were obtained using an electron microprobe analyser, a JEOL Hyperprobe JXA-8500F operated at 15 kV accelerating voltage and 10 nA beam current for silicates and at 20 kV and 20 nA for tungstates and sulfides. Samples of chlorite with W, (Cu,Mo) mineralization were analysed using doubly polished thick sections (200 µm). The major elements of minerals were determined using detection limits (3σ) of 0.03 wt.% for most oxides with counting times of 80 s. The standards used for silicates were: albite (NaKα), orthoclase (AlKα, SiKα, KKα), apatite (CaKα, PKα), MgO (MgKα), MnTiO3 (MnKα), TiO2 (TiKα), FeO (FeKα); for W minerals: cassiterite (SnKα), pure Ta (Lα), Mo (Lα), Ni (Kα), Co (Kα), Cu (Kα), Ag (Lα) and Au (Mα) metals, SrBa NbO4O12 (NbLα), scheelite (WLα), Sc2O3 (ScLα), UO2 (UMβ), ThO2 (ThMβ), galena (PbMα), LaP5O14 (LaLα), CeP5O14 (CeLα), YAg (YLα), Cd (Lα), Sb2S3 (SbLα), AsGa (AsLα), Bi2Se3 (BiMα). Calculation of the structural formula was based on 28 oxygens for chlorite and on 4 oxygens for the tungstate minerals. The Fe3+ estimation in chlorite was carried out according to Droop (Reference Droop1987) and Jacobson (Reference Jacobson1989).
Oxygen isotopes
Fe-, Fe,Mn- and Fe,Mg-chlorite samples (previously analysed by XRD) were selected for oxygen isotope analyses carried out at the Laboratory for Stable Isotope Science, University of Western Ontario (London, Ontario, Canada). Oxygen isotopic analysis of quartz samples from the W- and Mo- mineralization were analysed at the University of Salamanca (Spain). A conventional procedure was used for the extraction of oxygen from chlorite and quartz with BrF5 and quantitative conversion to CO2 prior to analysis by stable-gas mass spectrometry (Clayton and Mayeda, Reference Clayton and Mayeda1963). The δ18O values are reported in ‰ relative to Vienna-Standard Mean Ocean Water (V-SMOW) and normalized to the NBS-30 reference (+5.17‰ vs. V-SMOW) and NBS-28 (+9.34‰ vs. V-SMOW). The reproducibility of δ18O measurements is ±0.2‰ (1σ). The following isotope fractionation equations were used here between the mineral and water system: the chlorite–H2O (Wenner and Taylor, Reference Wenner and Taylor1971), the quartz–H2O (Clayton et al., Reference Clayton, O'Neil and Mayeda1972), and albite–H2O (Matsuhisa et al., Reference Matsuhisa, Goldsmith and Clayton1979).
Results
Macroscopic observations and optical microscopy
Five distinct assemblages of chlorite minerals were identified in the W, (Cu,Mo) ore deposit of Borralha (Fig. 3): (1) quartz + albite + adularia + Fe,Mg-chlorite; (2) Fe,Mn-chlorite + scheelite; (3) Fe-chlorite + wolframite + scheelite; (4) Fe-chlorite + chalcopyrite + sphalerite ± pyrite + secondary muscovite (+ sericite); (5) Fe,Mg-chlorite + molybdenite + bismuthinite ± adularia + quartz. Mg,Fe-chlorite with chalcopyrite was observed by optical and electron microscopy. Chlorite minerals observed in transmitted light were recognized easily by their green pleochroism, varying from pale green for some massive aggregates to dark green with a basal cleavage and anomalous birefringence in cross-polarized light. Under the microscope, Fe,Mn-chlorite is characterized by strong green pleochroism with an atypical dark orange interference (Fig. 4a,b), which is in accordance with octahedral occupancy by Mn2+ (Bailey, Reference Bailey and Bailey1988). The elongation sign is opposite to the optical sign and the optical plane is almost parallel to (010). The Fe,Mn-chlorite aggregates are larger (800–1500 µm) than those of the Fe-chlorite. Fe-chlorite (Fig. 4c,d) shows flaky micrometric aggregates with greenish pleochroism and a much lower birefringence compared to Fe,Mn-chlorite. Small flaky aggregates of Mg,Fe-chlorite (Fig. 4e,f) with chalcopyrite show a randomly oriented grey to pale green pleochroism and small sheet aggregates, <100 µm in size.
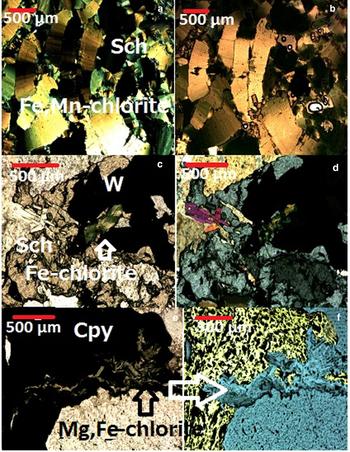
Fig. 4. Photomicrographs showing textural relationships between chlorite and W- and sulfide-mineralization: (a,b) Fe,Mn-chlorite and scheelite (a = plain polarized light, b = crossed nicols); (c,d) Fe-chlorite and W mineralization: wolframite (W) and scheelite (Sch) (c = plain polarized light, d = crossed nicols); (e,f) Mg,Fe-chlorite and chalcopyrite (cpy) in reflected light (e = plain polarized light, f = crossed nicols).
Structural data
Oriented specimens of Fe-, Fe,Mn- and Fe,Mg-chlorite were examined both air-dried (AD) and following treatment with ethylene-glycol (EG) vapour (Supplementary material, see below). XRD patterns of the three samples studied show the (00l) reflections distinctive of chlorite minerals, where the 002 peak at 7 Å is sharper than the 001 peak at 14 Å and the 001 and 003 peaks show approximately equal intensities. This is a typical structural feature for Fe-chlorite, where a large number of Fe atoms are distributed between the two octahedral sites. The different relative intensities of the 00l reflection planes may be used to measure the total metals (e.g. Ni, Co, Cr, Mn) incorporated between the silicate and hydroxide octahedral sheets (Brown and Brindley, Reference Brown, Brindley, Brindley and Brown1980). Greater intensities of the 00l reflections in Fe,Mn-chlorite are due to the presence of Mn (measured by EMPA) in the octahedral sheet. Increasing the amounts of Fe and Mn in the chlorite structure causes a decrease in the 001, 003 and 005 reflections relative to the 002 and 003 reflections. The normal 001 peak at 14.2 Å occurs at 14.60 Å which is typical of clinochlore (daphnite), or at 14.3 Å for pennantite. This is explained by the presence of the metals in the hydroxide layer and, to a lesser extent, by the presence of a few tri-smectite layers interstratified with tri-chlorite. There is a marked effect of Fe symmetry on the intensity ratio of the 001 and 003 peaks and approximately equal intensities indicate a symmetrical Fe substitution. The 001 and 005 reflections are weak relative to the 003 reflection due to the presence of Fe in the hydroxide layer. After EG treatment the peak positions did not change, but the intensity of the 00l peaks decreased. This probably indicates that expandable layers such as those of smectite do not occur in these chlorites although changes in the oxidation state may complicate this issue.
Textural relationships: chlorite vs. mineralization
The areas of the sections examined by means of optical microscopy were selected for detailed subsequent analysis by electron microscopy for to better understand the textural relationships between the chlorite minerals and the oxides or sulfides. Several textural relationships were examined, in particular those involving Fe,Mn-chlorite and scheelite (Fig. 5a), Fe-chlorite and wolframite and scheelite (Fig. 5b), Fe-chlorite and wolframite (Fig. 5c), Fe-chlorite vs. wolframite and Mg,Fe-chlorite vs. chalcopyrite (Fig. 5d), Fe-chlorite + secondary muscovite + chalcopyrite + wolframite (Fig. 5e), and Fe,Mg-chlorite along the cleavage planes of molybdenite (Fig. 5f).
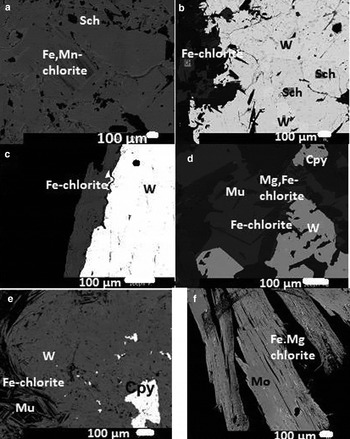
Fig. 5. Back-scattered electron images: (a) Fe,Mn-chlorite + scheelite; (b) Fe-chlorite + wolframite and scheelite; (c) Fe-chlorite and wolframite; (d) Fe-chlorite, muscovite (Mu) and wolframite; Mg,Fe-chlorite and chalcopyrite (cpy); (e) Fe-chlorite, wolframite and chalcopyrite; (f) Fe,Mg-chlorite along the cleavage planes of molybdenite (Mo).
Crystal chemistry of the chlorite minerals
Fe,Mn-chlorite
The EMPA analyses of Fe,Mn-chlorite (Table 1, analyses 1, 2, see also Supplementary material) show an average crystal chemical composition corresponding to: (Al2.62Fe7.53Mn1.09Mg0.62Zn0.02Ca0.01)11.89(Si5.31Al2.69)8O20(OH)8. The Si⇔Al(IV) exchange does not confirm tetrahedral substitution, but there is evidence of R 2+ exchange (Mn2+ for Mg2+ or Mn2+ for Fe2+) in the octahedral sheet. The Fe3+ calculated is present in minor amounts. It is assumed here that Fe3+ replaces only Al(VI) in the chlorite structure. Small amounts of Zn2+, Ca2+, Na+ and K+ were identified in Fe,Mn-chlorite. The oxidized chlorites are distinguished by the Si contents of 5.6–6.2 atoms per formula unit (apfu), whereas for the non-oxidized chlorites the Si contents range from 5 to 7 apfu for a maximum of 8 apfu (Deer et al., Reference Deer, Howie and Zussman1976). The Fe/(Fe + Mg) ratio shows an average value of 0.92 (Supplementary material). Compositionally, the Fe,Mn-chlorite falls between daphnite and amesite (see Fig. 11) in the diagram of R 2+ vs. Si4+ (Wiewióra and Weiss, Reference Wiewióra and Weiss1990).
Table 1. EMPA results of chlorite and calculated structural formula based on 28 oxygens.
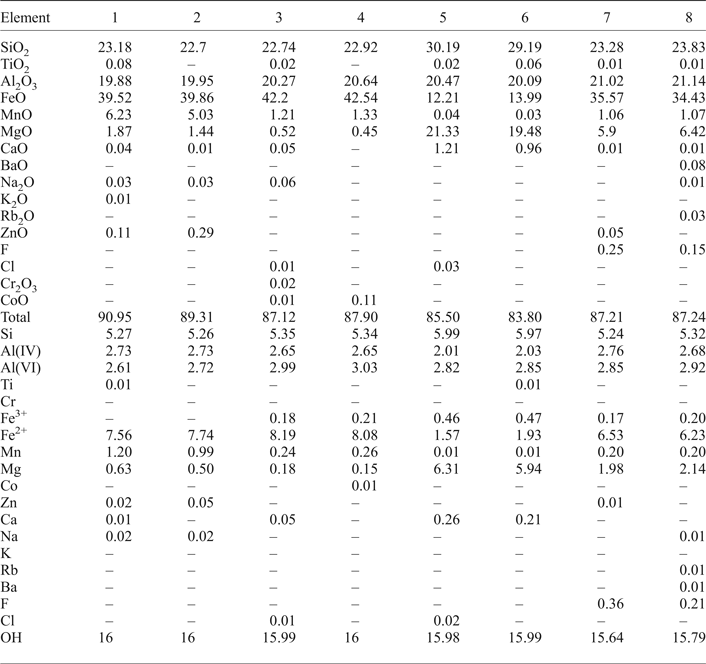
Fe2+/Fe3+ and OH calculated assuming full site occupancy.
1, 2 Fe,Mn-chlorite (around scheelite crystals).
3, 4 Fe-chlorite (around wolframite and scheelite integrowth).
5, 6 Mg,Fe-chlorite. 7, 8 Fe,Mg chlorite.
Fe-chlorite
The EMPA analyses of Fe-chlorite (Table 1, analyses 3, 4; see also Supplementary material) display an average crystal chemical composition of: (Al3.01Fe3+0.25Fe2+7.95Mn0.26Mg0.19)11.66(Si5.44Al2.56)8O20(OH)8. Small amounts of Mn and Mg (<0.34 apfu) are present and the Fe/(Fe + Mg) average ratio is ~0.98 (Supplementary material), suggesting an oxidized state. The chlorite composition corresponds to daphnite. These compositions are similar to those of Fe-chlorite occurring with chalcopyrite and secondary muscovite (sericite) (Figs 3d and 5e).
Mg,Fe-chlorite
The EPMA analyses (Table 1, analyses 5, 6; see also Supplementary material) show an average chemical composition characterized by small Al and Fe contents: (Al2.63Fe3+0.37Fe2+1.72 Mn0.01Mg6.40Ca0.26)11.39(Si6.02Al1.98)8O20(OH)8. This reflects crystallization conditions which are different from those related to the formation of Fe,Mg-chlorite. The Mn2+ is ~0.01 apfu and Ca2+ ~0.26 apfu, higher than was found in the chlorite samples analysed previously. The Fe/(Fe + Mg) average ratio is ~0.26 (Supplementary material) and the chlorite composition corresponds to pycnochlorite.
Fe,Mg-chlorite
The average crystal chemical composition (Table 1, analyses 7, 8; see also Supplementary material) is (Al2.89Fe3+0.24Fe2+6.42Mn0.21Mg2.08)11.84(Si5.21Al2.79)8F0.31O20(OH)8. A decrease in the Al, Fe and Mn contents coupled with an increase in the F content is clear and it is considered to be a typical feature of this Fe,Mg-chlorite. The Fe/(Fe + Mg) average ratio is ~0.76 (Supplementary material) and the chlorite composition corresponds to that of ripidolite.
X-ray maps of chlorite minerals vs. tungstate and sulfide minerals
X-ray maps illustrate the Fe and Mn distribution and support the notion of chlorite crystallization and metal precipitation directly from the fluid, as discussed below.
Fe,Mn-chlorite
The area illustrated in the inset of Fig. 6 shows Fe,Mn-chlorite associated with scheelite grains. The Fe,Mn and Mg distributions are distinct and reflect the incorporation of Fe followed by Mn. The X-ray maps show the W and Ca distributions in the scheelite (Fig. 7, inset) and the EPMA of the scheelite (Table 2, analyses 1, 2; see also Supplementary material) indicate traces of Sn, Pb, Bi and Mo.
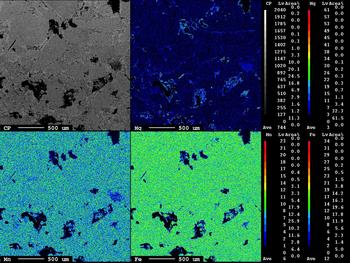
Fig. 6. X-ray maps of Fe, Mn and Mg distributions in Fe,Mn-chlorite. Black areas correspond to scheelite. (CP – composite.)
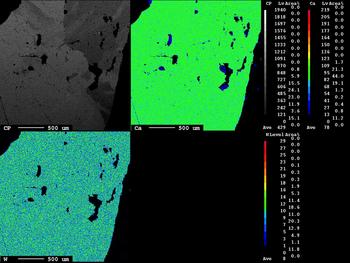
Fig. 7. X-ray maps of Ca and W distributions in scheelite.
Table 2. EPMA results from scheelite and wolframite, and calculated structural formulae based on 4 oxygens.
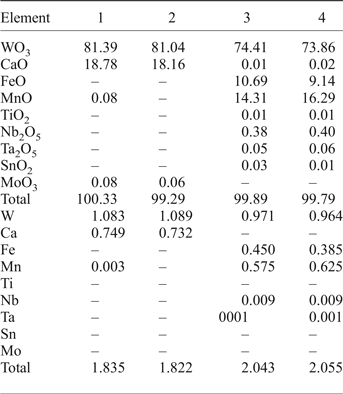
1, 2 scheelite.
3, 4 wolframite.
Bi, Sc, Th, U, Pb below detection.
Fe-chlorite
The area illustrated is a veinlet infill of Fe-chlorite aggregates associated with wolframite (Fig. 8). The X-ray map shows that the Fe distribution in the chlorite aggregates is clearly defined, Mg is limited to the edges of the chlorite aggregates and Mn is confined to the central part of the chlorite (Fig. 8, inset). The EMPA analyses of the wolframite (Table 2, analyses 3, 4; see also Supplementary material) show trace amounts of Nb, Ta, Sn and Ti. The wolframite is not homogeneous revealing variations of in terms of the MnO and FeO composition as reported by Noronha (Reference Noronha1983). In contrast to the wolframite from Panasqueira which is enriched in the ferberite component (FeWO4), the hübnerite (MnWO4) component of wolframite is more significant at Borralha (Fig. 9) and as well as in other Iberian tungsten deposits (Neiva, Reference Neiva2008).
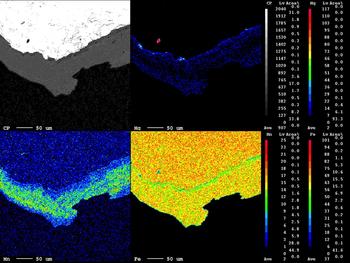
Fig. 8. X-ray maps of Fe, Mn and Mg distributions in Fe-chlorite associated with wolframite.
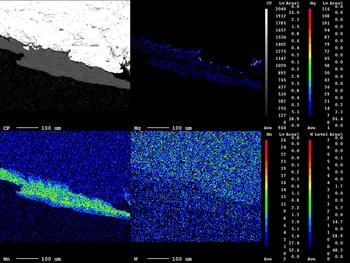
Fig. 9. X-ray maps of Mg, Mn and W distributions in wolframite (hübnerite).
Mg,Fe-chlorite
The areas illustrated correspond to chlorite veins close to chalcopyrite. The back-scattered image (Fig. 10, inset) shows aggregates of secondary muscovite (sericite) which may be a greisen-type alteration, and Fe-chlorite associated with wolframite and chalcopyrite. Particular attention was given to the X-ray map of Mg distribution, where small chlorite aggregates associated with chalcopyrite are enriched in Mg (shown in red) at the expense of Fe.
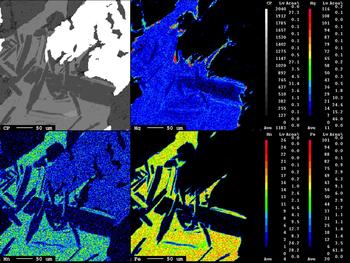
Fig. 10. X-ray maps of Fe, Mn and Mg distributions in Fe-chlorite and Mg,Fe- chlorite (red areas).
Chlorite geothermometry
Chlorite shows a wide compositional range because it is sensitive to P-T and redox conditions, as well as the bulk-rock and fluid composition (Walshe, Reference Walshe1986; Cathelineau, Reference Cathelineau1988; Vidal et al., Reference Vidal, Parra and Trotet2001, Reference Vidal, Parra and Vieillard2005, Reference Vidal, de Andrade, Lewin, Muñoz, Parra and Pascarelli2006; Inoue et al., Reference Inoue, Meunier, Patrier-Mas, Rigault, Beaufort and Vieillard2009; Bourdelle et al., Reference Bourdelle, Parra, Chopin and Beyssac2013; Lanari et al., Reference Lanari, Wagner and Vidal2014). Here we used the geothermometry model of Bourdelle et al. (Reference Bourdelle, Parra, Chopin and Beyssac2013) and Bourdelle and Cathelineau (Reference Bourdelle and Cathelineau2015) which considers all the iron to be Fe2+. Those authors justified this approach by the fact that, accounting for Fe3+ in the structural formula, fixed numbers of oxygens reduce the number of cations, implying an increase in octahedral vacancies. That in turn creates an indirect link between the Fe3+ content and the temperature. The chlorite temperature estimates are controlled in the first place by Tschermak substitution, where Fe3+ has a subordinate role that may be disregarded as a first approximation. The mineral compositions were plotted in the Si4+ vs. R 2+ diagram (Wiewióra and Weiss, Reference Wiewióra and Weiss1990), and four distinct geothermometric fields were (Fig. 11): (1) Fe,Mn-chlorite falls into a field with T ranging from 400 to 500°C; (2) Fe-chlorite plots in a field where T varies from 250 to 350°C; (3) Fe,Mg-chlorite corresponds to a field with T of ~200–250°C; and (4) Mg,Fe-chlorite falls in a field with T of ~150°C.
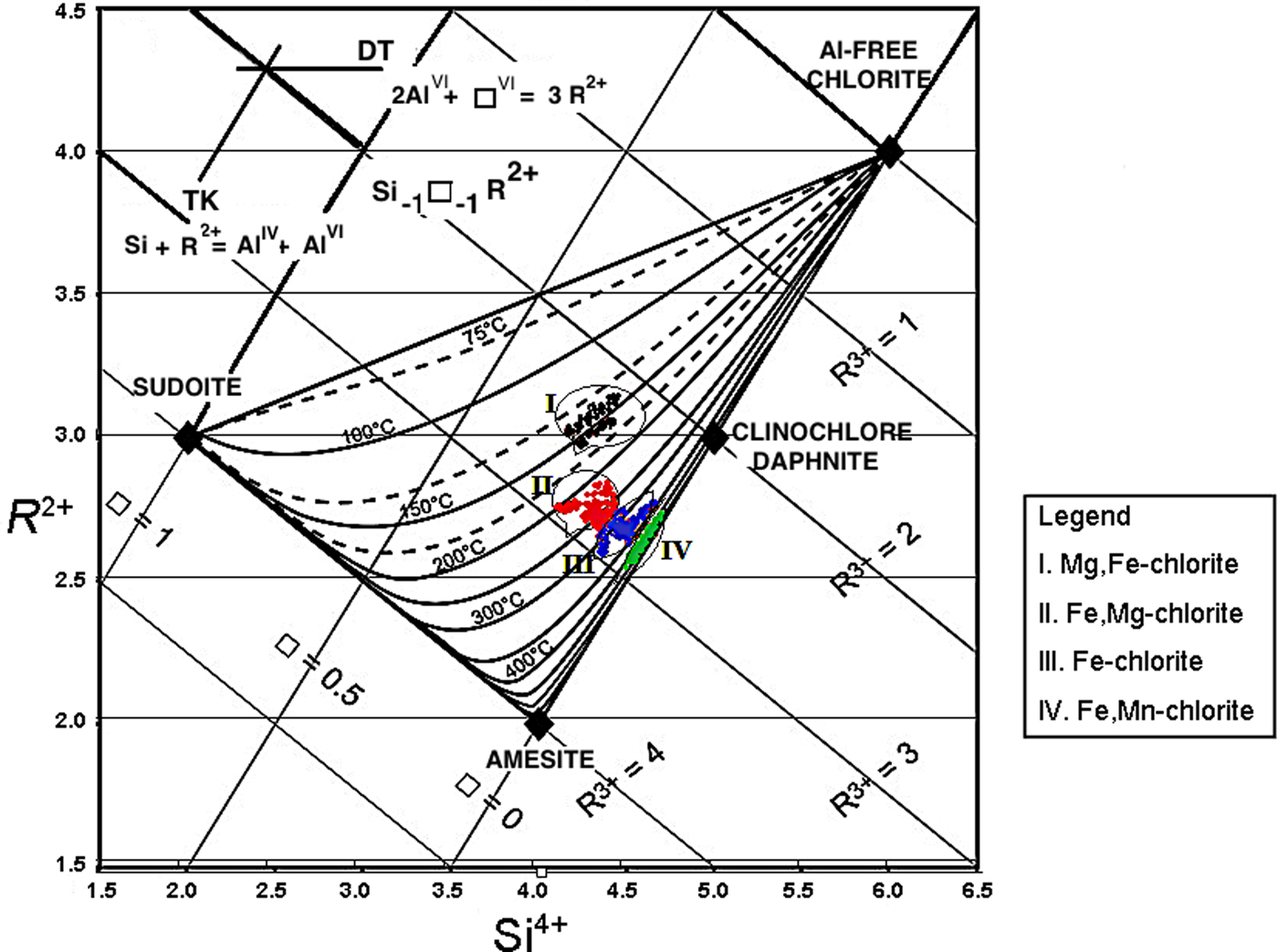
Fig. 11. Projection of chemical compositions of Fe,Mn-chlorite (I), Fe-chlorite (II), Fe,Mg-chlorite (III) and Mg,Fe-chlorite (IV) in the R 2+–Si diagram) for chlorite compositions (Wiewióra and Weiss, Reference Wiewióra and Weiss1990). The temperature estimation is after Bourdelle et al. (Reference Bourdelle, Parra, Chopin and Beyssac2013).
Stable isotope data
Oxygen isotopic measurements of the Fe-, Fe,Mn-, Fe,Mg-chlorites, quartz from W- and Mo-mineralization (Santa Helena and Venise breccia pipes), adularia from the quartz + Fe,Mg-chlorite + molybdenite assemblage and the oxygen isotopic fractionations between chlorite, quartz, adularia and H2O related to W- and sulfide mineralization are shown in Table 3. These data are compared with the isotopic data for quartz from the Gerês late-orogenic granitic massif (Jaques et al., Reference Jaques, Noronha, Liewig and Bobos2016) and from the Panasqueira deposit (Polya et al., Reference Polya, Foxford, Stuart, Boyce and Fallick2000).
Table 3. Oxygen isotope data of chlorite, quartz and adularia from the W, (Cu, Mo) ore deposit of Borralha, Portugal.
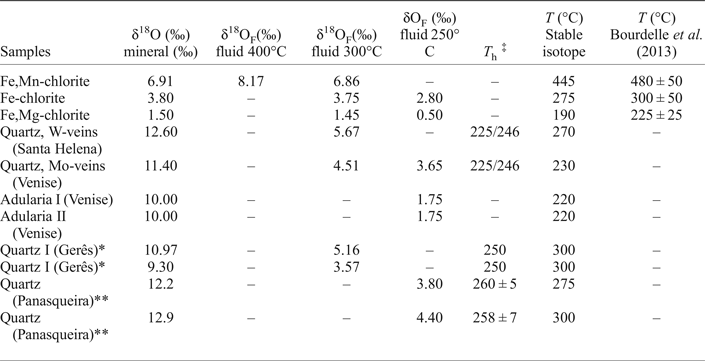
* After Jaques et al. (Reference Jaques, Noronha, Liewig and Bobos2016); **After Polya et al. (Reference Polya, Foxford, Stuart, Boyce and Fallick2000); ‡Homogenization temperature (T h) after Noronha (Reference Noronha1984a); Jaques et al. (Reference Jaques, Noronha, Liewig and Bobos2016); Polya et al. (Reference Polya, Foxford, Stuart, Boyce and Fallick2000).
The δ18O isotope (V-SMOW) of the chlorites yielded values of +1.5 (1σ) for (Fe,Mg-chlorite), +3.8 (1σ) for (Fe-chlorite) and +6.91 (1σ) for (Fe,Mn-chlorite). The isotopic fractionation for chlorite and H2O calculated with the bond-type approach depends on the composition which should be known before interpreting the isotopic data (Cole, Reference Cole1985, Reference Cole1994; Savin and Lee, Reference Savin, Lee and Bailey1988). Oxygen isotopic exchange experiments in the chlorite-H2O system (Wenner and Taylor, Reference Wenner and Taylor1971) were calculated assuming a temperature of 200–500°C, inferred from fluid-inclusion studies, and deduced on the basis of chlorite geothermometry according to the procedure described by Bourdelle et al. (Reference Bourdelle, Parra, Chopin and Beyssac2013). The δ18OF (fluid, F) oxygen isotopic fractionation for the chlorite-H2O pair (Wenner and Taylor, Reference Wenner and Taylor1971), assuming a temperature of 300°C, yield values (Table 3) for the ancient fluid values of +1.45 (1σ), +3.75 (1σ) and +6.86 (1σ) in equilibrium with Fe,Mg-, Fe- and Fe,Mn-chlorite, respectively. Assuming a minimum temperature of 400°C for the crystallization of Fe,Mn-chlorite, the calculated δ18OF yielded a value of 8.17 (1σ). The δ18OF calculated for Fe,Mg-chlorite and Fe-chlorite yielded values of +0.50 (1σ) and +2.80 (1σ) at a temperature of 250°C.
Quartz collected from W-mineralization (Fig. 3c, Santa Helena breccia) has δ18O (V-SMOW) of +12.6 (1σ) and from Mo-mineralization (Fig. 3a, Venise breccia) of +11.40 (1σ). The δ18OF fractionation for the quartz-H2O pair (Clayton et al., Reference Clayton, O'Neil and Mayeda1972) yields a value of +5.67 (1σ) and +4.51 (1σ) for the quartz precipitation at 300°C, based on isotope thermometry. Lower values of +3.65 (1σ) and +2.45 (1σ) were obtained for quartz at a temperature of 250°C close to the isotopic values obtained from the ancient fluid responsible for the Fe-chlorite crystallization.
The δ18O isotopes (V-SMOW) measurement on adularia collected from the Venise breccia (Fig. 3a,e) yielded a value of +10 (1σ), where the δ18OF fractionation for the feldspar-H2O pair (Matsuhisa et al., Reference Matsuhisa, Goldsmith and Clayton1979) is +1.75 (1σ).
Discussion
Previous studies of the Borralha W, (Cu,Mo) ore deposit have demonstrated a clear relationship between mineralization and chlorite formation, with ripidolite and daphnite intimately associated with the evolving stage of sulfide deposition (Noronha, Reference Noronha1983, Reference Noronha1984a,Reference Noronhab; Mateus, Reference Mateus1985). The progression from tungstate to sulfide deposition coupled with chlorite crystallization identified in this W, (Cu,Mo) ore system, records an unequivocal connection with the chemical evolution of mineralizing fluids along with the declining P-T conditions under which the ore-forming processes took place. The following mineral assemblages are well documented: Fe,Mn- and Fe-chlorite crystallize during the W-ore stage, where a Cu contribution was indicated by the presence of chalcopyrite, whereas Fe,Mg- and Mg,Fe-chlorite throughout the sulfide ore stage is characterized by molybdenite, bismuthinite and pyrite assemblages.
Wolframite crystallized from fluids with slightly acid to neutral pH, where WO42− is easily complexed with Fe2+, Mn2+ and Ca2+. Note that stability of the Fe2+ end-member (ferberite) is much more sensitive to the large variation of f O2 than is the Mn2+ end-member wolframite (hübnerite); the composition of ferberite might be affected strongly by the oxidation state of the environment (Hsu, Reference Hsu1976). Furthermore, tungsten may be transported in various aqueous solutions under different conditions where the dominant species above 400°C is tungstic acid, whereas HWO4− and WO42−, and the alkali-tungstate ion pairs are the most stable species at lower temperatures (Higgins, Reference Higgins1985; Wood and Vlassopoulos, Reference Wood and Vlassopoulos1989).
Experimental studies on the stability of H4[Si(W3O10)4].2H2O at high P-T conditions and variable pH in the presence of Fe2+, Mn2+ and Ca2+ have confirmed the separation of silico-tungstic acid under the effect of solution neutralization (Gundlach, Reference Gundlach1967) according to the reaction:

Two distinct tungstate vs. silicate assemblages are demonstrated by this work: one consists of scheelite and Fe,Mn-chlorite, whereas the other involves Mn-rich wolframite ± scheelite + Fe-chlorite. In the first case, [WO4]2− combined preferentially with Ca2+ to form scheelite, favouring the incorporation of the existing Mn2+ into the chlorite structure. Crystallization of scheelite together with Mn-bearing wolframite suggests the availability of all three bivalent cations in the hydrothermal fluid. Thus, if Mn2+ is partitioned primarily to Mn-bearing wolframite and Ca2+ to scheelite, chlorite will be enriched in Fe2+ and small amounts of Mn2+ (~0.26 apfu) occur in Fe-chlorite, as demonstrated by the Mn-rich wolframite ± scheelite + Fe-chlorite assemblage.
Fe-rich wolframite crystallized in more acidic conditions than did Mn-rich wolframite and scheelite, and the presence of scheelite associated with Mn-rich wolframite, indicate that the minerals are not contemporary. Mn-rich wolframite is associated with a late-evolving W-mineralization stage possibly triggered by rising alkalinity of the fluid as a result of an increase in S activity and the Ca2+ availability of the system. This can be correlated with a late alkaline metasomatic reaction which is very common in geochemical settings surrounding the development of W-ore deposits (Wood and Samson, Reference Wood and Samson2000).
The result of [SiO4]4− and [WO4]2− separation is direct crystallization of scheelite (I) from the magmatic-hydrothermal fluid and Fe,Mn-chlorite followed by Mn-rich wolframite and scheelite (II) together with Fe-chlorite from the hydrothermal-meteoric fluid. The observed Mn-rich wolframite + scheelite (II) + chalcopyrite assemblage confirms a mixed Mn-rich wolframite-sulfide evolving stage, where scheelite replaced Mn-rich wolframite (seen clearly during optical microscopy) and sulfide minerals precipitated according to the reaction:
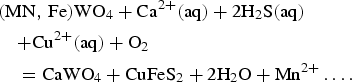
The common association of tungstate with some sulfide minerals indicates that sulfur is commonly present and plays a role in the stability of wolframite whereas the stability fields of ferberite (FeWO4) and hübnerite (MnWO4) do not depend on the presence of oxygen and sulfur (Hsu, Reference Hsu1975). Crystallization of Mg,Fe-chlorite associated with chalcopyrite (Fig. 5d) in the Santa Helena breccia, in a mineral assemblage comprising Fe-chlorite, secondary fine-grained muscovite (sericite), Mn-bearing wolframite and scheelite, is particularly interesting. The suite of mineralogical reactions should be expected to begin with the silicates followed by the development of the tungstate minerals. The presence of the chalcopyrite + Mg,Fe-chlorite assemblage suggests that Fe from wolframite was consumed during the crystallization of chalcopyrite, whereas the Mg,Fe-chlorite is a byproduct after the formation of secondary muscovite from biotite according to the reaction:

In this case, the Mg,Fe-chlorite is not a suitable guide to sulfide mineralization.
Crystallization of Fe,Mg-chlorite associated with molybdenite in the Venise breccia pipe is clearly related to different geochemical conditions imposed by some chemical rejuvenation of hydrothermal fluids, expressed by an increase in f S2 and a pH variation from alkaline to neutral pH. Molybdenite, in contrast to ferberite or hübnerite, crystallized in a more reduced environment according to the reaction:

This reaction generates more protons in the fluid and produces conditions favourable to the destabilization of primary biotite, and enriching the fluid in Mg2+ and Fe2+. Releasing this fluid through the cleavage planes of molybdenite, contributed to the later crystallization of Fe,Mg-chlorite in the system (Fig. 5f). The distribution of Fe,Mg-chlorite is widespread and often occurs together with hydrothermal quartz, adularia or secondary albite (Fig. 3a) which means that the fluid was widely distributed throughout in the Venise breccia pipe.
The Fe- and Fe,Mn-chlorite is described here associated with W-mineralization for the first time in the magmatic-hydrothermal Borralha system. The structure and chemistry of the chlorites reveal important details of the conditions of crystallization. The XRD data confirmed that there are no expandable phases in the Fe-, Fe,Mn- or Fe,Mg-chlorite structure, thus making them suitable for geothermometry. The presence of metals (i.e. Mn) in the chlorite structure affected the 002/001 intensity ratios. Large amounts of Fe [Fe/(Fe + Mg + Mn) = 0.4–0.95] and Mn [Mn/(Fe* + Mg) > 0.05] in chlorites are diagnostic of the chemical environments during ore formation (Inoue et al., Reference Inoue, Kurokawa and Hatta2010).
The temperatures estimated using the method of Bourdelle et al. (Reference Bourdelle, Parra, Chopin and Beyssac2013) indicate three events correlated with the mineralization stages described by Noronha (Reference Noronha1983): (1) Early tungsten stage: formation of scheelite (I) and Fe,Mn-chlorite at temperatures from 400 to 500°C; (2) Late tungsten-sulfide stage II: formation of Mn-rich wolframite + scheelite (II) + sulfide (chalcopyrite), and Fe-chlorite and secondary muscovite at 250–350°C; and (3) Sulfide stage III: formation of molybdenite (+bismuthinite) and Fe,Mg-chlorite at ≤250°C.
The δ18OF (+6.86 and +8.16 1σ) values calculated (Wenner and Taylor, Reference Wenner and Taylor1971), responsible for Fe,Mn-chlorite crystallization in the temperature range of 300–400°C, show that the Fe,Mn-chlorite and scheelite (stage I) were likely to have been deposited from magmatic-hydrothermal fluids. The oxygen isotopic results from Fe,Mn-chlorite are very close to those reported by Borsevski et al. (Reference Borsevski, Dolomanova, Lisovskaia, Medvedovskaia and Rojdestvenskaia1979) for chlorite from Sn-ore deposits in the Transbaikal (Serlovaia Gora and Hapceranga) Russia, where measured values of +6.1 and + 4.4‰ are due to equilibration with a hydrothermal fluid with an isotopic signature of ~+6.05‰.
The temperature estimation of tungsten and sulfide deposition (stage II) based on fluid-inclusion studies of quartz from the Borralha deposit ranges from 270 to 350°C for tungsten associated with aqueous-carbonic fluids with an average salinity of 10 wt.% eq. NaCl; and from 250 to 300°C for chalcopyrite associated with aqueous fluids (Noronha, Reference Noronha1984b). Oxygen isotopic results from Fe- and Fe,Mg-chlorite (stage II and III) confirm the same isotopic association between chlorite and the aqueous fluid. Assuming a temperature of ~300°C, the calculated δ18OF for the Fe-chlorite-H2O pair is ~+3.75 (1σ). The δ18O isotopic data obtained on quartz from W (Santa Helena breccia) mineralization yielded a value of +12.6 (1σ), in equilibrium with a calculated δ18OF of +5.67 (1σ). Such a δ18OF value, quite close to a magmatic-hydrothermal signature, would suggest that quartz and Fe-chlorite formation could be related to a mineral–water system with a mixed contribution from meteoric and magmatic-hydrothermal fluids during the late tungsten-sulfide (II) stage. The δ18O isotopic data of Fe,Mg-chlorite related to Mo-mineralization (Venise breccia, stage III) is light and close to +1.45 (1σ), assuming a temperature of 190°C where the calculated δ18OF is ~+0.05 (1σ). The estimated temperatures are supported by the geothermometer of Bourdelle et al. (Reference Bourdelle, Parra, Chopin and Beyssac2013) for Fe,Mg-chlorite crystallization between 200 and 250°C.
The δ18OF fractionation for the feldspar-H2O pair (Matsuhisa et al., Reference Matsuhisa, Goldsmith and Clayton1979) in the case of adularia yielded a value of +1.75 (1σ) and for the quartz-H2O pair ~+4.45 (1σ). The δ18O values for waters that deposited the latest assemblage of quartz-Fe,Mg-chlorite-adularia range from +0.50 to +4.45 (1σ). Oxygen isotope thermometry of early quartz and adularia indicate equilibrium temperatures of ~220°C (Matsuhisa et al., Reference Matsuhisa, Goldsmith and Clayton1979).
The isotopic signatures can be compared with the δ18O data obtained from quartz (episyenitic rocks) from the post-D3 Hercynian granite of Gerês, which revealed values of ~+10.97‰ ± 1.9‰, in equilibrium with an aqueous fluid where the calculated δ18OF is ~+5.16‰ (Jaques et al., Reference Jaques, Noronha, Liewig and Bobos2016). δ18O values of 10 and 11‰ have been determined for other granites in the Portuguese Hercynian rocks (Reavy et al., Reference Reavy, Stephens, Fallick, Halliday and Godinho1991).
Magmatic quartz from the Panasqueira (Portugal) W-ore, has a high δ18O value of ~+13‰ and the δ18O value of primary magmatic waters is +11 ± 1‰ (Kelly and Rye, Reference Kelly and Rye1979). The fluids responsible for ore formation exhibited relatively constant fluid inclusion homogenization temperatures (254–260°C) with salinities of 7.4–8.7 wt.% NaCl equivalent and a calculated fluid δ18O with values from 3.8 to 4.4‰ (Polya et al., Reference Polya, Foxford, Stuart, Boyce and Fallick2000). This means, that the ore-forming stage fluid can be explained by isotopic exchange of meteoric waters equilibrated with granite at ~350°C (Polya, Reference Polya1989).
Conclusions
Chlorite minerals may indicate enriched, and economically more favourable, hydrothermal systems. The samples studied show a genetic link between Fe-, Fe,Mn- and Fe,Mg-chlorite and W- and Mo-mineralization supported by textural relationships, geothermometry and isotopic signatures. The composition of chlorite was influenced directly by thermal gradients developed during different stages of the mineralizing process in the Borralha magmatic-hydrothermal system. The identification of a possible hydrothermal alteration halo was not possible, but the chlorite phases associated with W- and Cu,Mo-mineralization are an excellent indicator which help to identify the direction in which the mineralized area may be found and a means of estimating the crystallization temperature of chlorite and tungstate or sulfide.
Acknowledgements
The present study was developed in the context of the NEWORES-ERAMIN project financed by FCT-Lisbon, Portugal. The manuscript benefitted from thoughtful reviews by K. Sundblad (Univ. of Turku), K. Billström, (Univ. of Lund) and R. Törnroos (Univ. of Helsinki) which greatly improved the final version. The authors gratefully acknowledge J.F.W. Bowles for editorial handling. Thanks are also due to F. Guimarães (LNEG-Porto, EMPA facility), S. Leal (geological map design), and V. Ramos (photomicrographs). The authors thank Eric O'Brien for help with the English.
Supplementary material
To view Supplementary material for this article, please visit https://doi.org/10.1180/minmag.2017.081.104