Introduction
Interest in exploration for indium resources has been increasing world-wide (Schwarz-Schampera and Herzig, Reference Schwarz-Schampera and Herzig2002), not least in Europe due to the significant dependence on supply for the high-technology electronics industry using this critical metal.
Indium rarely forms minerals of its own, but is usually enriched in the crystal structure of other minerals like sphalerite and chalcopyrite in polymetallic Cu- and Zn-sulfide deposits (Schwarz-Schampera and Herzig, Reference Schwarz-Schampera and Herzig2002) and is typically closely related geochemically with Sn-bearing minerals in sulfide deposits (Qian et al., Reference Qian, Xinzhi, Jiayong and Shuxun1998). High indium concentrations in sphalerite from Sn-rich polymetallic ores are reported commonly worldwide e.g. from Freiberg in Germany, Mt. Pleasant, New Brunswick in Canada and Goka in Japan (Seifert and Sandmann, Reference Seifert and Sandmann2006).
Indium-bearing polymetallic veins have also been discovered in southern Finland (Fig. 1), mainly in the Sarvlaxviken area in the anorogenic 1.64 Ga Wiborg rapakivi batholith (Cook et al., Reference Cook, Sundblad, Valkama, Nygård, Ciobanu and Danyushevsky2011; Valkama et al., Reference Valkama, Sundblad, Nygård and Cook2016b) but also in the adjacent 1.90 Ga Svecofennian crust (Villar et al., Reference Villar, Sundblad and Lokhov2016; Villar, Reference Villar2017). The structurally controlled quartz veins in the Sarvlaxviken area are exceptional for the high indium contents, presence of roquesite and very high indium contents in sphalerite and chalcopyrite (Cook et al., Reference Cook, Sundblad, Valkama, Nygård, Ciobanu and Danyushevsky2011).

Fig. 1. Location of the Wiborg Batholith within the Fennoscandian Shield. The Sarvlaxviken area is indicated with a square in the western part of the Wiborg Batholith.
The purpose of this investigation is to use fluid inclusion data on the indium-bearing polymetallic quartz veins in the Sarvlaxviken area in order to identify a depositional model for these veins and to bring new data and an overall insight into the genesis of indium-bearing vein deposits in anorogenic rapakivi granites. It is evident already from the studies of Cook et al. (Reference Cook, Sundblad, Valkama, Nygård, Ciobanu and Danyushevsky2011); Valkama et al. (Reference Valkama, Sundblad, Cook and Ivashchenko2016a,Reference Valkama, Sundblad, Nygård and Cookb); Villar et al. (Reference Villar, Sundblad and Lokhov2016) and Villar (Reference Villar2017) that the exploration potential for indium is huge in all rapakivi areas of the Fennoscandian Shield.
Geological background
The Fennoscandian Shield (Fig. 1) is a Precambrian multi-orogenic crustal complex in northern Europe, which consists of 3.1–2.6 Ga Archaean crust in the northeast and 2.5–0.9 Ga Proterozoic crust in the central and southwestern parts (Gaál and Gorbatschev, Reference Gaál and Gorbatschev1987). One of the most significant pieces of the Proterozoic crust was formed during the Svecofennian, when 1.92–1.89 Ga juvenile suspect terranes formed off-shore an Archaean continent. These exotic fragments collided subsequently with the Archaean continent and resulted in a series of metamorphic events of which the last one created anatectic melts and formation of 1.80–1.84 Ga Late Svecofennian granites (Gaál and Gorbatschev, Reference Gaál and Gorbatschev1987). Anorogenic rapakivi granites were emplaced as batholiths and stocks into the Svecofennian crust during the Mesoproterozoic of which the 1.65–1.63 Ga Wiborg Batholith (Fig. 1), in the south-eastern part of the shield, is the oldest and largest (Vaasjoki et al., Reference Vaasjoki, Rämö and Sakko1991; Rämö and Haapala, Reference Rämö, Haapala, Lehtinen, Nurmi and Rämö2005).
The Sarvlaxviken area is located in the southeastern part of the Fennoscandian Shield, ~90 km east of Helsinki, Finland, along the western margin of the Wiborg Batholith (Figs 1 and 2). The Svecofennian crust in that area is dominated by 1.84 Ga Late Svecofennian anatectic microcline granites (Kurhila et al., Reference Kurhila, Mänttäri, Vaasjoki, Rämö and Nironen2011). The microcline in these Late Svecofennian granites has been altered thermally to orthoclase in a 10–20 km narrow zone along the intrusive margin of the Wiborg Batholith, when this rapakivi granite complex was emplaced into the Svecofennian crust (Vorma, Reference Vorma1972; Villar et al., Reference Villar, Sundblad and Lokhov2016). The Wiborg Batholith is dominated by wiborgite, a spectacular coarse-grained granite type, with 1–3 cm sized rounded orthoclase phenocrysts, mantled by a rim of plagioclase, within an even-grained groundmass of quartz, plagioclase, orthoclase, biotite and occasional hornblende (Simonen and Vorma, Reference Simonen and Vorma1969). Other rapakivi granite varieties in the Sarvlaxviken area include the Hormnäs, Stormossen and Marviken granites, which constitute <1 km even-grained stocks and dykes, all representing later intrusion stages than the wiborgite, but still belonging to the Wiborg Batholith (Nygård, Reference Nygård2016).

Fig. 2. Distribution of granite types, veins and ore boulders in the Lillträsket-Sarvlaxviken area. Sample numbers are indicated for the Lillträsket, Mjölknäs, Virbäcken and Korsvik-1 veins.
The igneous components in the Wiborg Batholith are typical A-type granites with a pronounced within-plate granite character; the wiborgites have chondrite-normalized rare-earth element (REE) fractionation patterns with significant negative Eu anomalies and enrichments in light rare-earth elements (LREE) compared to the heavy rare-earth elements (HREE), while the later (topaz-bearing) granites display even more pronounced negative Eu anomalies and have fairly flat chondrite-normalized REE fractionation trends (Rämö and Haapala, Reference Rämö, Haapala, Lehtinen, Nurmi and Rämö2005). The individual granite types in the Sarvlaxviken area are no exceptions to this (Nygård, Reference Nygård2016); the wiborgite in the Sarvlaxviken area shows all the geochemical characteristics of the wiborgites elsewhere in the batholith, including similar chondrite-normalized REE fractionation patterns and low Rb/Ba ratios (~0.2) while the Hormnäs, Stormossen and Marviken granites (Fig. 2) display significantly different contents of these elements. The Marviken granite is the most extreme of them, with pronounced enrichments of the HREE and very high Rb/Ba ratios (~14), thus having typical characteristics of an ore-fertile magma (Nygård, Reference Nygård2016). A N-S trending porphyric granite dyke, with a similar geochemical pattern to the Marviken granite, cuts the wiborgite on the northeastern side of the Sarvlaxviken bay, parallel with the polymetallic quartz veins at Korsvik, Högberget, Virbäcken and Mjölknäs, a few hundreds of metres east and west of the porphyric dyke respectively.
Polymetallic vein deposits, of various styles and host rocks, have been discovered recently in the Sarvlaxviken area (Fig. 2); quartz veins in wiborgite at Korsvik, Högberget, Mjölknäs and Virbäcken (Cook et al., Reference Cook, Ciobanu, Shimizu, Danushevskiy, Valkama and Sundblad2008, Reference Cook, Sundblad, Valkama, Nygård, Ciobanu and Danyushevsky2011; Valkama et al., Reference Valkama, Sundblad, Nygård and Cook2016b), two types of alteration assemblages in the Marviken granite (Valkama et al., Reference Valkama, Sundblad, Nygård and Cook2016b) and a third type of alteration assemblage in the orthoclase-altered parts of the Late Svecofennian granite, <1 km from the intrusive contact of the Wiborg Batholith (Villar et al., Reference Villar, Sundblad and Lokhov2016; Villar, Reference Villar2017). All vein types, also those in the Late Svecofennian granite, are considered to have formed from the late-stage rapakivi granites (Valkama et al., Reference Valkama, Sundblad, Nygård and Cook2016b; Villar et al., Reference Villar, Sundblad and Lokhov2016; Villar, Reference Villar2017).
The wiborgite-hosted polymetallic deposits occur in three distinct generations (#1, #2a, #2b) of NNE- to NNW-trending quartz veins (Valkama et al., Reference Valkama, Sundblad, Nygård and Cook2016b). Most parts of the veins consist of quartz, with minor amounts of dark mica, chamosite and fluorite. Sulfide and/or oxide opaque minerals occur irregularly and are locally the dominant vein minerals for a few metres along strike. The oldest veins (generation #1) constitute the Högberget type, characterized by a 5 cm distinct alteration halo around the 0.5 cm thick quartz vein and a Li-As-W-Zn-dominated metal association. The ore minerals are principally arsenopyrite with smaller amounts of sphalerite and chalcopyrite, together with minor bornite, molybdenite, cassiterite, pyrite, galena and wolframite. The Mjölknäs type is characterized by a Pb-Zn-dominated metal association, represented by galena and sphalerite, and was also considered to belong to generation #1 by Valkama et al. (Reference Valkama, Sundblad, Nygård and Cook2016b) although there are no crosscutting relations to other vein generations to support this statement. Veins belonging to generation #2a (Virbäcken type) and #2b (Korsvik type) have been distinguished from generation #1 (and from each other) based on crosscutting field relations; vein generation #2a has generally a NNE trend while the dominating trend for vein generation #2b is NNW. Furthermore, the two vein generations (#2a and #2b) also show variations in the metal contents although both are characterized by a Cu-As-In-dominated metal association; the Ag grades are distinctly higher in generation #2a and the In grades increase from generation #1 (0–100 ppm), via generation #2a (40–150 ppm) to generation #2b (600–1500 ppm). The ore minerals are dominated by chalcopyrite with smaller amounts of arsenopyrite and sphalerite together with minor roquesite, pyrite, cassiterite, stannoidite, bornite, galena, wolframite and Bi-minerals (Valkama et al., Reference Valkama, Sundblad, Nygård and Cook2016b).
A paragenetic summary of the opaque phases in the Högberget (generation #1), Virbäcken (generation #2a) and Korsvik (generation #2b) veins is presented in Fig. 3, showing increasing abundance of chalcopyrite, cassiterite, wolframite and roquesite, but decreasing abundance of molybdenite, sphalerite, galena and silver minerals through time. This can also be seen in the metal contents in these veins, reported by Valkama et al. (Reference Valkama, Sundblad, Nygård and Cook2016b); increasing contents of Cu, Sn, W and In, but decreasing contents of Mo, Zn, Pb and Ag through time. From a structural perspective, the fractures hosting vein generations #2a and #2b are considerably more brittle than the fractures hosting vein generation #1, indicating more shallow deposition levels with time. In consequence, if the paragenetic, geochemical and structural observations are matched with each other, we see a contradictory, or even chaotic, zonation pattern with respect to where in the vein system each metal precipitated.

Fig. 3. Paragenetic summary of the opaque phases in the Högberget (generation #1), Virbäcken (generation #2a) and Korsvik (generation #2b) veins.
Positive evidence for intense ore-forming processes in the Marviken granite and in the Late Svecofennian granites, the latter immediately west of the Wiborg Batholith, exist through discoveries of numerous local boulders with metal enrichments as well as extensive and complex soil anomalies with respect to a number of metals, but sulfide-bearing veins in outcrop have so far not been located in these granites. The occurrences of metal-rich veins in the Marviken granite can, according to studies of the ore-bearing boulders, be divided into a Cu-As-Sn-dominated type in 1–3 cm thick zones of greisen alteration assemblages and a Mo-Bi-dominated type in a 20–30 cm thick zone in a Be-rich alteration assemblage (Valkama et al., Reference Valkama, Sundblad, Nygård and Cook2016b). Metal enrichments in the Late Svecofennian granite have also been revealed south of Lake Lillträsket, where a 50 cm wide Zn-rich alteration zone in a granite boulder has been found associated with a widespread and complex geochemical soil anomaly (with respect to Zn, Cd, In, Ag, Fe, Pb, Bi and As). A short (<20 m) glacial transport distance from the bedrock source has been suggested for these soil anomalies, based on detailed ground magnetic anomaly patterns (unpublished data) and susceptibility data, partly on the bedrock and partly in the same soil samples in which the geochemical anomalies were detected (Vind, Reference Vind2014). The metal-rich soil anomalies occur where the Late Svecofennian granite is part of the thermal alteration zone that Vorma (Reference Vorma1972) identified for the transfer of microcline into orthoclase outside the Wiborg Batholith. This thermal alteration is accompanied by widespread hydrothermal potassic alteration in the Lillträsket area, which led Villar et al. (Reference Villar, Sundblad and Lokhov2016) and Villar (Reference Villar2017) to conclude that metal enrichments in the concealed bedrock must be located in the Late Svecofennian granite, <1 km from the intrusive margin of the Wiborg Batholith.
Sample material
Fourteen samples of hydrothermal 0.5–2 cm thick vein quartz and associated ore minerals have been collected for the present study. Sample numbers, vein name and coordinates are summarized in Table 1. All samples were carefully selected in order to provide a meaningful relation between the fluid inclusion data from the quartz (and/or fluorite/cassiterite) and the precipitation conditions for the opaque minerals. Most of the samples investigated in the present study (Korsvik, Virbäcken and Mjölknäs) are those for which Cook et al. (Reference Cook, Sundblad, Valkama, Nygård, Ciobanu and Danyushevsky2011) and Valkama et al. (Reference Valkama, Sundblad, Nygård and Cook2016b) made their observations of ore minerals. This implies that the fluid inclusion data in this study have been obtained mainly from quartz (and/or fluorite/cassiterite) grains at distances of mm to cm from the ore minerals (or even intergrown with them), regardless of the In grades. The only vein for which larger distances exist between quartz investigated for fluid inclusions and abundant opaque minerals is the Högberget-1 vein where the fluid inclusion samples (KS1401 and KS1402) were taken at a distance of 10–20 m along strike and in the same vein, from where Valkama et al. (Reference Valkama, Sundblad, Nygård and Cook2016b) reported intense occurrence of ore minerals (Figs 4 and 5a). Sample KS1406 was collected in another quartz vein (Högberget-2; generation #1), 80 m WSW of the Högberget-1 vein. The sample location for KS1406 is at a distance of 1 m from sample EN1329, for which Valkama et al. (Reference Valkama, Sundblad, Nygård and Cook2016b) reported high Li, W and As contents. The sample of the Mjölknäs vein, generation #1 (SWAS 205), is identical to the sample documented by Valkama et al. (Reference Valkama, Sundblad, Nygård and Cook2016b).
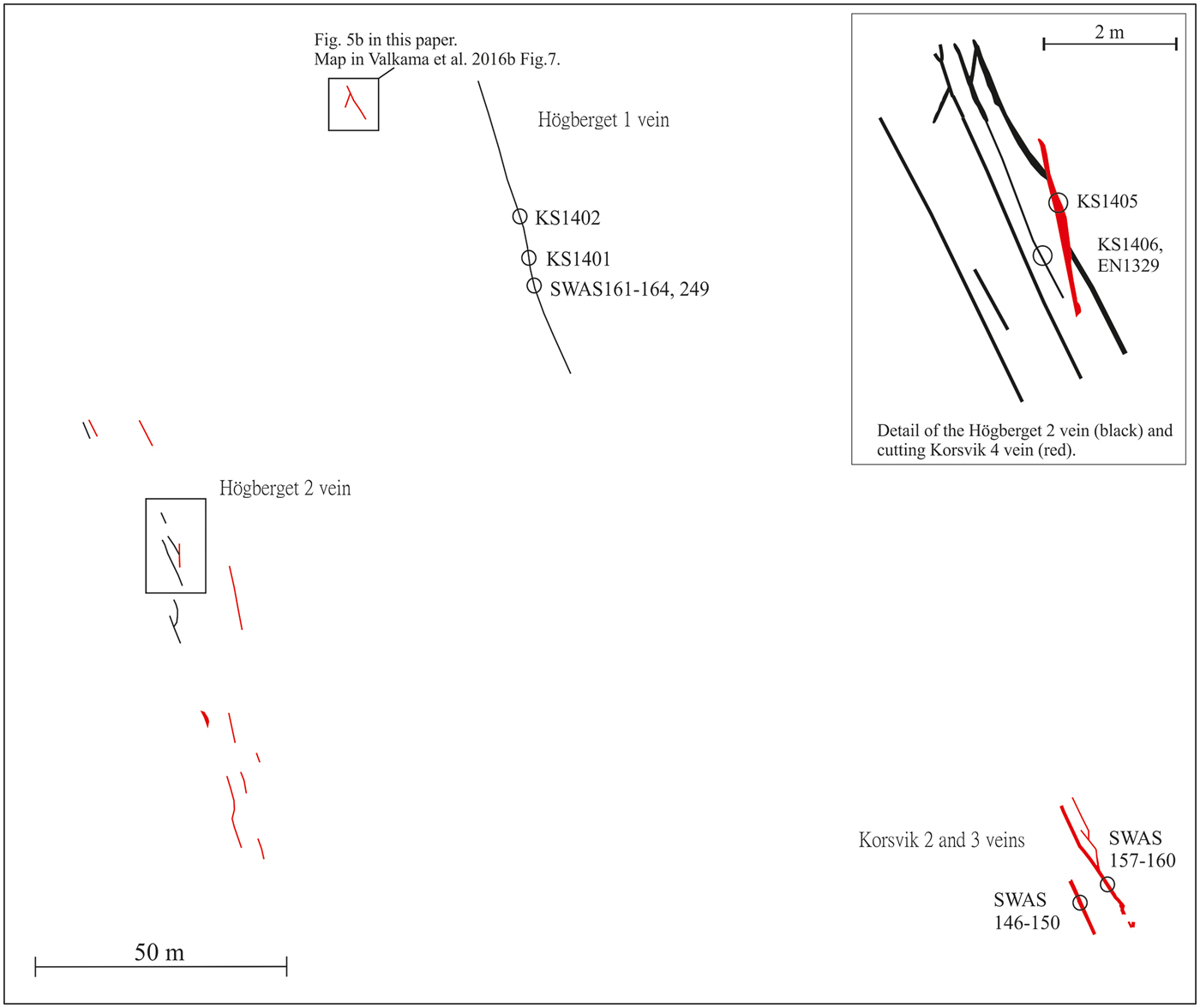
Fig. 4. Spatial relations between the polymetallic quartz veins in the Högberget-Korsvik area with sample numbers indicated for the Högberget-1 and -2 veins as well as the Korsvik -2, -3 and -4 veins. Black lines represent the Högberget type (generation #1) and the red lines the Virbäcken and Korsvik types (generations #2a and #2b).

Fig. 5. (a) The Högberget-1 vein, representing generation #1, photographed towards N by Jeremy Woodard (with Nadya Priyatkina as scale) on the site where sample KS 1402 was collected. (b) Cutting relations between quartz veins representing generations #2a and #2b at Högberget (photo modified after Valkama et al., Reference Valkama, Sundblad, Nygård and Cook2016b).
Table 1. Fluid inclusion samples.
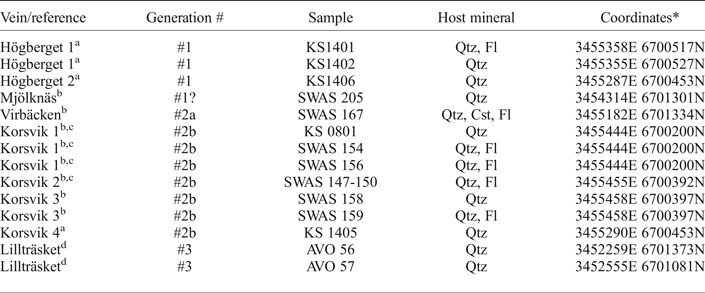
References: a = this study, b = Valkama et al. (Reference Valkama, Sundblad, Nygård and Cook2016b), c = Cook et al. (Reference Cook, Sundblad, Valkama, Nygård, Ciobanu and Danyushevsky2011), d = Villar (unpublished data). Qtz = quartz, Fl = fluorite, Cst = cassiterite.
* Coordinates E/N in the Finnish national grid system.
Samples representing generation #2a; SWAS 167 (the Virbäcken vein) and generation #2b; KS0801, SWAS 154, SWAS 156, SWAS 147-150, SWAS 158 and SWAS 159 (the Korsvik veins) are also the same as those in which Cook et al. (Reference Cook, Sundblad, Valkama, Nygård, Ciobanu and Danyushevsky2011) and Valkama et al. (Reference Valkama, Sundblad, Nygård and Cook2016b) reported abundant ore minerals. Sample KS 1405 was collected from a quartz vein that belongs to generation #2b, cutting and faulting the Högberget-2 vein (belonging to generation #1, see Figs 4 and 5b). Since all metal-rich veins in the Marviken and Late Svecofennian granites are hosted by various types of alteration assemblages (and not in proper quartz veins), it was not possible to collect adequate hydrothermal minerals for fluid inclusion studies from the Marviken and Lillträsket areas. Instead, two barren hydrothermal quartz vein samples (generation #3), assumed to represent the rapakivi-induced epigenetic metal-enriched environment in the Late Svecofennian granite, were sampled south of Lake Lillträsket (AVO 56 and AVO 57).
Analytical techniques
The samples were prepared at the University of Turku as doubly-polished 150 μm thick sections (Fig. 6) for fluid inclusion observation and analysis. A conventional petrographic microscope was used to get an overview of the samples and the distribution of fluid inclusions. The microthermometric analyses were performed with a Linkam THM 600 heating/freezing stage (working range −196 to +600°C) adapted for a Nikon microscope at the Department of Geological Sciences, Stockholm University. Calibration was made with commercial SynFlinc® synthetic fluid inclusion standards. Phase transitions of fluid inclusions were observed with a 40× long-working distance objective. The accuracy of the measurements is estimated to be ±0.1°C for temperatures below +30°C and ±1.0°C for higher temperatures. Fluid inclusions with evidence of leakage or necking down were not analysed. Salinities of aqueous fluid inclusions were calculated after data in Bodnar (Reference Bodnar, Samson, Anderson and Marshall2003) while determination of salinities from gas hydrate dissociation temperatures in CO2-bearing aqueous inclusions was made after data in Bakker et al. (Reference Bakker, Dubessy and Cathelineau1996).

Fig. 6. Doubly polished thick section of sample SWAS 158 from the Korsvik-3 vein (generation #2b), with sulfide/oxide minerals deposited along the contact to the host granite.
Fluid inclusion studies
To obtain an adequate documentation of the fluids involved in the formation of both the ore and the gangue minerals (most ore minerals are not suitable for conventional microthermometric analysis), fluid inclusions were studied on quartz, either associated spatially with the ore minerals or where it is the dominating vein mineral and more rarely on occasional cassiterite and fluorite grains. Fluid inclusions in all samples belonging to all vein generations (#1, #2a, #2b and #3), have a random three-dimensional distribution in the host minerals and were classified as primary fluid inclusions according to Roedder (Reference Roedder1984). The sizes of the inclusions are in general <10 μm, but can reach 35 μm in quartz, 20 μm in cassiterite and 45 μm in fluorite. The inclusions in vein generations #1, #2a and #2b consist mainly of an aqueous liquid and a vapour (Fig. 7a,b,c), but in a few quartz-hosted inclusions there is also an additional carbon dioxide component in the vapour phase (Fig. 7d). The inclusions in quartz and cassiterite appear with variable proportions of the liquid and the vapour phase (from 5 to almost 90 vol.% vapour), whilst the inclusions in fluorite have constant phase ratios with ~5 vol.% vapour (Fig. 7e). The quartz-hosted fluid inclusions in the samples belonging to vein generation #3 are all liquid aqueous fluid inclusions.

Fig. 7. (a and b) Quartz-hosted fluid inclusions with varying phase proportions. (c) Vapour-rich fluid inclusion in cassiterite. (d) CO2-bearing aqueous fluid inclusions in quartz. (e) Aqueous fluid inclusions in fluorite.
Microthermometric results
The microthermometric results are presented in Table 2 and in Figs 8 and 9. The initial melting of ice (the temperature, at which first liquid is recognized during warming of frozen inclusions) was observed for all samples (except for the CO2-bearing inclusions) in the interval from −22° to −30°C which indicate that NaCl and KCl are the most likely principal salts (Davis et al., Reference Davis, Lowenstein and Spencer1990) in the aqueous solution (even though smaller amounts of other salts such as CaCl2 can be present). The initial melting temperature was observed for the majority of the inclusions between the metastable eutectic at −28°C and the stable eutectic at −22.9°C of the H2O–NaCl–KCl system (Davis et al., Reference Davis, Lowenstein and Spencer1990). A composition dominated by CaCl2, or other divalent cations, would give significantly lower initial melting temperatures (Davis et al., Reference Davis, Lowenstein and Spencer1990).

Fig. 8. Total homogenization temperatures (°C) plotted vs. salinity (eq. mass% NaCl) in fluid inclusions in quartz (circles), cassiterite (squares) and fluorite (diamonds) from the Sarvlaxviken veins. Diagrams (a) and (b) represent the Högberget-1 vein (generation #1), diagram (c) the Högberget-2 vein (generation #1), diagram (d) the Mjölknäs vein (generation #1?), diagram (e) the Virbäcken vein (generation #2a) while diagrams (f) to (l) represent the Korsvik veins (generation #2b). Further information about the samples is given in Table 1.

Fig. 9. A plausible interpretation of the homogenization temperatures and salinities obtained from this study. Hot vapour, exsolved from a magma, rises (red arrow) from depth along the fracture and interacts with the granite walls. Condensation, cooling and boiling of the vapour (blue arrows) result in a liquid with progressively higher salinity by continuous vapour-liquid separation.
Table 2. Microthermometric data from Sarvlaxviken.

L = homogenization to the liquid state, V = homogenization to the vapour state.
Final melting of ice in all aqueous inclusions hosted by vein quartz generation #1, #2a and #2b, took place in the range 0 to −12.3°C, which corresponds to salinities of 0–16.2 eq. mass% NaCl. Dissociation of gas hydrates in the CO2-bearing aqueous inclusions occurred between +4.0 and +7.1°C which correspond to a salinity of 5.0–8.0 eq. mass% NaCl. The measurements revealed no discernible differences between the quartz vein generations #1, #2a and #2b (Figs 8 and 9). Inclusions with low salinities dominate in all samples, but higher salinities are often recorded in metal-rich parts of the veins. The inclusions hosted by cassiterite show final ice melting temperatures of −3.0 to −5.8°C, corresponding to salinities of 4.9–8.9 eq. mass% NaCl. Fluid inclusions in fluorite have final ice melting between −0.2 and −3.3°C and a salinity of 0.3–5.4 eq. mass% NaCl.
Total homogenization temperatures of fluid inclusions in quartz were recorded over a broad temperature interval from 73 to 410°C, including CO2-bearing inclusions, with homogenization both to the liquid and vapour state. Cassiterite shows a similar range of homogenization temperatures to liquid and vapour from 111 to 519°C. Fluorite has a more limited range of temperatures from 123 to 169°C with homogenization to liquid in all cases.
Partial homogenization of the CO2 in the few aqueous CO2-bearing inclusions occurred at +20.6 to +28.2°C to liquid and vapour. Melting of the CO2 was observed between −56.9 and −57.2°C, indicating an almost pure CO2 phase with less than 3 mol.% CH4 or N2 (based on data from van den Kerkhof and Thiéry, Reference van den Kerkhof and Thiéry2001).
The fluid inclusions in the quartz vein samples from the Lillträsket area (generation #3) lack a gas bubble and, therefore, it was not possible to obtain a homogenization temperature, but this type of inclusion is assumed to have been trapped at temperatures below ~100°C (Roedder, Reference Roedder1984). The salinity of aqueous inclusions is normally calculated from the ice melting temperature of two-phase liquid and vapour inclusions, but to avoid metastable ice melting in these all-liquid inclusions (Roedder, Reference Roedder1984), final ice melting temperatures were measured after inducing an artificial vapour bubble in the inclusions by heating and stretching them. After this treatment they displayed final ice melting temperatures between −0.3 and −0.6°C which correspond to salinities in the range 0.5–1.1 eq. mass% NaCl (Fig. 9).
Discussion
The ore-forming conditions in the Sarvlaxviken area
The fluid inclusion data for the veins in the Sarvlaxviken area demonstrate that the mineralizing fluid that entered the fractures in the wiborgite was a low- to moderately-saline aqueous fluid. A few randomly occurring inclusions enclose an additional pure CO2 phase, which implies that the aqueous fluid initially contained some CO2. Magmatic fluids commonly contain CO2 as one of the volatile components (Heinrich, Reference Heinrich2005) and fluid inclusions in In-enriched vein deposits have been reported to include CO2 together with an aqueous low- to moderately-saline fluid (Schwarz-Schampera and Herzig, Reference Schwarz-Schampera and Herzig2002; Moura et al., Reference Moura, Botelho, Olivo, Kyser and Pontes2014). However, the significance of the CO2 phase is not completely established, but the appearance of a CO2 phase in a few inclusions, and not in all, suggests that the CO2 has separated and escaped from an originally homogenous H2O–CO2 fluid, a process that is known to occur in response to the rapid cooling and a pressure decrease (Wilkinson, Reference Wilkinson2001). Furthermore, the presence of fluorite and sulfides in the veins demonstrates that the metal-transporting fluid also carried F and S.
Even if it had been possible to distinguish between three distinct generations of ore-bearing quartz veins in the Sarvlaxviken area, the paragenetic sequences (Fig. 3) and metal associations (Valkama et al., Reference Valkama, Sundblad, Nygård and Cook2016b) show complex and ambiguous zonation patterns with respect to time and assumed depositional levels in the crust. The first vein generation (#1), which also represents the deepest deposition level, has the highest Zn and Pb contents (as well as significant W grades). The second vein generation (#2a) shows the highest Ag grades, while the third vein generation (#2b) that is associated with brittle fracturing at a presumed high crustal level has the highest contents of Cu, Sn and W, metals that normally form in the bottom of a complex vein system. The fluid inclusion data support this chaotic system; there are no clear temperature gradients when moving from generation to generation. Instead, the fluid inclusion data from the three vein generations #1, #2a and #2b are indistinguishable and indicate that the same fluid was involved in all three generations.
The variable phase proportions in coexisting fluid inclusions suggest that the precipitation appears to have been very sudden and that the inclusions were sealed synchronously under heterogeneous conditions (Roedder, Reference Roedder1984; Wilkinson, Reference Wilkinson2001). The alternatives, that the fluid inclusions were affected by post-entrapment deformation related modifications (stretching and recrystallization; Audétat and Günther, Reference Audétat and Günther1999) with leakage and changes in shape and composition, or the inflow of some unrelated fluid, are not to be expected as the quartz veins are well preserved and not deformed. When volatiles exsolve from a magma in an early stage of immiscibility, a separate aqueous volatile-rich fluid is produced. Upon ascent, this aqueous phase commonly unmixes during a second stage of immiscibility into a highly saline (30–70 eq. mass% NaCl) liquid and a low-salinity (<10 eq. mass% NaCl) vapour phase (Bodnar et al., Reference Bodnar, Burnham and Sterner1985; Roedder, Reference Roedder1992; Heinrich, Reference Heinrich2005). The salinities of the two phases depend on the actual PT conditions. Even though the salinities of the measured fluid inclusions of the Sarvlaxviken quartz veins span a wide range, no salinities were obtained above 16 eq. mass% NaCl. This suggests that the aqueous fluid trapped as inclusions represents the upward moving vapour phase after unmixing from a liquid, or an aqueous phase directly separated from a magma. The somewhat higher salinity (up to 16 eq. mass% NaCl), compared to what is usually reported (<10 eq. mass% NaCl) for the low-salinity vapour phase, and the spread in homogenization temperatures (~100 to ~500°C) may be explained by combined processes of phase separation involving condensation, cooling and boiling of an initially low-salinity (<3 eq. mass% NaCl) vapour (Fig. 9) during its ascent through the fractured granite. Discharge of the fluids along faults or fracture systems, associated with the magmatic activity, resulted in a conductive cooling of the vapour by interaction with the fractured wall rock. Decreased temperature by conductive cooling is an effective mechanism that will lead to a decreased solubility and precipitation of the metals transported in the fluid (Heinrich, Reference Heinrich2005).
At first, the aqueous fluid probably had a high temperature (500–600°C) and was exsolved during the last stage of magma crystallization (Fig. 10a). In response to fracturing (pressure decrease), liquid/vapour phase separation occurred and steam was formed that rose upwards in the fractures. It is generally believed (Schwarz-Schampera and Herzig, Reference Schwarz-Schampera and Herzig2002; Seifert and Sandmann, Reference Seifert and Sandmann2006) that In-bearing deposits originate from magmatic systems and that it's moderate geochemical incompatibility during magma crystallization and the high volatility (Sun, Reference Sun1982) eventually result in mobilization and transport of indium into the volatile-rich magmatic vapour. Hot magmatic vapour-like fluids can carry high concentrations of metals (Heinrich, Reference Heinrich2005). High-temperature (500–900°C) fumaroles of water vapour derived from a degassing magma contain significant amounts of dissolved indium (Symonds et al., Reference Symonds, Rose, Reed, Lichte and Finnegan1987). The F contents in the rapakivi granites in the Sarvlaxviken area are (as elsewhere in the Wiborg Batholith; Rämö and Haapala, Reference Rämö and Haapala1995, Reference Rämö, Haapala, Lehtinen, Nurmi and Rämö2005) commonly in the range of 0.1–0.2 wt.% but on average ca. 0.5 wt.% in the Marviken granite (Nygård, Reference Nygård2016), the latter thus a significantly F-rich granite. Experimental studies have indicated a strongly depressed solidus, due to the presence of an F-rich melt, to temperatures from 700°C to a final stage of 500°C (Costi et al., Reference Costi, Dalĺagnol, Pichavant and Rämö2009).

Fig. 10. Simplified model for the formation of the Sarvlaxviken polymetallic quartz veins; (a) Vapour exsolved from a magma rises from depth (red arrow), interacts and alters the walls of the fractured wiborgite. (b) Cooling of the ascending hot magmatic vapour along the fracture walls (blue arrows) leads to contact condensation into liquid water, reheating, boiling and subsequent cooling of the liquid phase (C = condensation and cooling, B = boiling) with a progressive increase in concentration of salt and metal components. Ore minerals are deposited. (c) The open fracture is finally filled and sealed by quartz.
As the temperature of the steam decreased adjacent to the contact with the fracture walls (Fig. 10b), droplets of a higher density liquid phase separated. Initially, at still relatively high temperatures, small portions of this liquid were carried as droplets in the ascending dispersed vapour flow, but with decreasing temperatures the droplets formed a descending liquid layer which became saturated with metals that started to precipitate where the concentration of salt increased. The salinity rose rapidly along the granite wall while salt-free water boiled off and passed upwards as steam, the process continued with a cooling/heating cycle with condensation of new droplets that fell back down the fracture where water was reheated and boiled off, like circulation in a steam engine. The highest salinities were recorded in fluid inclusions in metal-rich parts of the veins. Combined condensation and boiling of an aqueous liquid from a vapour phase is an effective mechanism for producing high-salinity fluids (Roedder, Reference Roedder1984). The vapour flow continued in the centre of the fracture, but was blocked near the fracture walls by the liquid condensate and the precipitated ore minerals and quartz (Fig. 10b). Finally, the fractures were sealed by quartz at which point the fluid flow ceased (Fig. 10c). Condensation, cooling and boiling of the vapour resulted in a turbulent flow with complex geometry and a temperature-salinity pattern which was reflected by the variable liquid-to-vapour proportions and salinities in the fluid inclusions. Published data of metal concentration in fluid inclusions from a variety of ore deposits show that different metals preferentially concentrate either in the liquid (e.g. Zn, Fe, Sn, Pb) or in the vapour phase (e.g. Cu, As) depending on whether they can be transported in the liquid as Cl-complexes or in the vapour as sulfur (HS)-complexes (Heinrich et al., Reference Heinrich, Günther, Audétat, Ulrich and Frischknecht1999). This may explain the irregular metal enrichments observed by Valkama et al. (Reference Valkama, Sundblad, Nygård and Cook2016b) in the Sarvlaxviken veins. The quartz veins in the Lillträsket area were also formed from late-stage granite activity, but at the very last stage of vein formation (thus considered to represent a generation #3) from a cool (<100°C) and low-salinity (<1 eq. mass% NaCl) aqueous fluid. The investigated quartz veins at Lillträsket are late and the fluid inclusion data can therefore not really be considered to reflect the conditions for the main widespread ore-mineralizing system in that area. However, it is instead highly likely that the fluids that were responsible for the metal concentration processes in the Lillträsket area were very similar to the fluids described for the three generations of polymetallic quartz veins in the Sarvlaxviken area. This assumption is also very realistic for the metal-enriched systems in the Marviken granite. This granite is by far the most F-rich granite in the area (and also displays other ore-fertile geochemical criteria like a high Rb/Ba ratios and characteristic inclined HREE pattern) and has a high potential to be a source for the necessary F-rich fluids. The fact that a granite dyke, with Marviken geochemical characters (high F contents, high Rb/Ba ratios and an inclined HREE pattern), exist on the eastern side of the Sarvlaxviken bay, parallel with adjacent polymetallic quartz veins, provides further support for a link between the Marviken granite (and associated dykes) and the ore-forming fluids responsible for the Sarvlaxviken quartz veins and the alteration dominated veins in the Lillträsket and Marviken areas.
Comparison with other In-bearing deposits
Indium-bearing deposits appear in a large variation of sizes and ore types (Schwarz-Schampera and Herzig, Reference Schwarz-Schampera and Herzig2002) such as polymetallic structurally controlled veins, complex granite-related vein-stockwork systems, volcanic-hosted massive Cu-Zn sulfide deposits, base-metal-rich porphyry copper deposits, skarn deposits, epithermal deposits and fumarole precipitates in active magmatic systems. Indium-rich deposits in polymetallic vein and complex granite-related vein-stockwork systems are among the most important types (Seifert and Sandmann, Reference Seifert and Sandmann2006).
The fluid inclusion data presented in this paper are basically similar to data for In-bearing vein-type deposits in Argentina, Bolivia, Canada, Germany and Japan (Schwarz-Schampera and Herzig, Reference Schwarz-Schampera and Herzig2002; Seifert and Sandmann, Reference Seifert and Sandmann2006; Sinclair et al., Reference Sinclair, Kooiman, Martin and Kjarsgaard2006; Jovic et al., Reference Jovic, Guido, Schalamuk, Ríos, Tassinari and Recio2011; Shimizu and Morishita, Reference Shimizu and Morishita2012) with proposed formation temperatures in the range from ca. 200 to 400°C. Differing results were presented by Dill et al. (Reference Dill, Garrido, Melcher, Gomez, Weber, Luna and Bahr2013) who suggested significantly lower deposition temperatures (between 130 and 250°C) for the San Roque epithermal Au-Cu-Zn-Pb-Ag veins in Argentina. An extended temperature interval (from <200 to 450°C) was proposed by Moura et al. (Reference Moura, Botelho, Olivo, Kyser and Pontes2014) for the Mangabeira Sn-In deposit in Central Brazil, which is more in conformity with the Sarvlaxviken data.
Based on the composition of condensates from fumaroles it was shown that metals were transported in magmatic vapour, predominantly as chloride species (Symonds et al., Reference Symonds, Rose, Reed, Lichte and Finnegan1987). Experimental work by Seward et al. (Reference Seward, Henderson and Charnock2000) has demonstrated that at 300–350°C, indium is preferably transported by the hydrothermal fluids as chloride (InCl−4) or hydrolysed (InClOH+) complexes. Fluid inclusion studies of the Sarvlaxviken veins show that metals and quartz precipitated from fluids with low to moderate salinities. Other In-enriched veins are typically characterized by <9 eq. mass% NaCl (Schwarz-Schampera and Herzig, Reference Schwarz-Schampera and Herzig2002; Seifert and Sandmann, Reference Seifert and Sandmann2006; Sinclair et al., Reference Sinclair, Kooiman, Martin and Kjarsgaard2006; Jovic et al., Reference Jovic, Guido, Schalamuk, Ríos, Tassinari and Recio2011; Shimizu and Morishita, Reference Shimizu and Morishita2012; Dill et al., Reference Dill, Garrido, Melcher, Gomez, Weber, Luna and Bahr2013). In the fluid inclusion study of the Mangabeira Sn-In deposit, Moura et al. (Reference Moura, Botelho, Olivo, Kyser and Pontes2014) recorded a large range of salinities, comparable to what now has been documented for the ore-forming fluid in the Sarvlaxviken area, i.e. in the range from 0 to 20 eq. mass% NaCl. Progressive cooling, shown by the spread in temperatures and salinities, has been explained as a result of mixing/dilution of the hot magmatic vapour with cooler meteoric water. The latter is suggested especially for epithermal deposits (Heinrich, Reference Heinrich2005; Seifert and Sandmann, Reference Seifert and Sandmann2006). However, an initial single-phase magmatic fluid can separate into coexisting phases with variable vapour to liquid ratios of low-salinity vapour and liquid with a higher salt content in response to declining P-T conditions (Heinrich, Reference Heinrich2005). As demonstrated in the present study of the ore-forming conditions in the Sarvlaxviken area, the wide variations in temperatures (from 100 to 500°C) and salinities (from 0 to 16 eq. mass% NaCl) can, as an alternative, be the results of combined processes involving condensation, cooling and boiling.
Conclusions
The In-bearing polymetallic quartz veins in the Sarvlaxviken area can be divided into three distinct generations (#1, #2a and #2b) based on their structural relationships and distinct metal associations. The latest metal-bearing vein generation (#2b) has exceptional indium grades (up to 1500 ppm) and shows evidence of brittle fracturing in open spaces. Fluid inclusion evidence presented in this study, provides new information on the transport and depositional processes of metals in anorogenic granites. The study shows that the combined phase separating processes; condensation, cooling and boiling of an aqueous magmatic vapour, enriched in CO2-F-Cl-S and metals, generated the In-bearing polymetallic veins in the Sarvlaxviken area. The combined processes resulted in a separation of the hot magmatic vapour into a liquid with enhanced salinities (up to 16 eq. mass% NaCl) and a low-salinity (<3 eq. mass% NaCl) vapour. Deposition occurred at temperatures from ~500°C to <100°C in a turbulent flow with complex geometry. Fluid inclusion data show that the depositional conditions were similar regardless of vein generation. The results point towards a model where the accumulation of specific metals at different vein sites or vein generations to a large extent depend on the relative abundance of transporting complexes; chloride in the liquid-dominated phase and sulfur species in the vapour-rich phase.
Even though no fluid inclusion data were generated for the metal-rich alteration veins in the Lillträsket and Marviken areas, it is assumed that the fluids that created these metal accumulations were of the same kind as the fluids that were responsible for the polymetallic quartz veins in the Sarvlaxviken area. All these fluids are considered to have been generated by the F-rich Marviken granite (and dykes), which show all geochemical criteria for an ore-fertile granite.
The investigated quartz veins in the Lillträsket area are considered to represent a very late vein generation (#3), that were deposited from the final residual low-temperature and (at this stage) barren fluid phase.
Acknowledgements
Financial support for this study was received from the K.H. Renlund Foundation. Arto Peltola is thanked for the preparation of doubly polished thin sections. The constructive comments of reviewers (Jens Andersen and Maria Pura Alfonso) contributed to improvement of this manuscript.